Introduction
Magnesium (Mg2+) is an essential mineral( Reference Leroy 1 ) and its binding is of central importance for enzymic reactions after combining with the enzyme or substrate. The cytosolic concentration of the free, ionised Mg2+ ion is about 1 mmol/l, but the total intracellular concentration is much higher since numerous anions such as phosphate groups in nucleic acids or ATP4– bind Mg2+ ( Reference McGuigan, Kay and Elder 2 ). Furthermore, Mg2+ acts as a modulator of synaptic transmission in the central nervous system (CNS)( Reference Morris 3 , Reference Möykkynen, Uusi-Oukari and Heikkila 4 ), at the motoric endplate( Reference Lamb and Stephenson 5 ), in immunological pathways( Reference Li, Chaigne-Delalande and Kanellopoulou 6 ) and in timekeeping( Reference Feeney, Hansen and Putker 7 ). Importantly, Mg2+ is involved in the gating of ion channels( Reference Vemana, Pandey and Larsson 8 ). Many transient receptor potential (TRP) channels are regulated by Mg2+ in a voltage-dependent manner( Reference Voets, Janssens and Prenen 9 ) and are involved in the transport of cations across the ruminal epithelium( Reference Rosendahl, Braun and Schrapers 10 – Reference Leonhard-Marek, Stumpff and Brinkmann 12 ).
The modulation of channel function in the CNS by Mg2+ is probably the reason for neurological symptoms such as ataxia, recumbency, convulsions, and finally tetanic muscle spasms in hypomagnesaemia and has been well known in cattle for some 80 years as grass tetany( Reference Sjollema 13 , Reference Sjollema 14 ).
The present review attempts to outline the principles of Mg2+ homeostasis with particular emphasis on the site and mechanism of Mg2+ absorption, renal excretion and possible imbalances such as tetany.
Magnesium homeostasis
Plasma magnesium
Despite the absence of a (known) specific regulatory system, Mg2+ in plasma is kept within the range of 0·9–1·2 mmol/l, provided that influx (via absorption) into the extracellular space (ECS) including plasma is larger than the efflux (requirement and excretion). In humans, six genomic regions have been implicated in the maintenance of plasma Mg2+ concentration( Reference Meyer, Verwoert and Hwang 15 ), and similar gene loci may explain the heritability of plasma Mg2+ concentration in dairy cows (0·20–0·43)( Reference Tsiamadis, Banos and Panousis 16 ).
Plasma Mg2+ is known to be influenced in a non-specific manner by catecholamines( Reference Rayssiguier 17 ), insulin( Reference Persson and Luthman 18 ) and parathyroid hormone (PTH)( Reference Goff, Littledike and Horst 19 ). In addition, epidermal growth factor has recently been shown to have magnesiotropic effects via TRPM6 channels, which regulate renal and intestinal Mg2+ absorption( Reference Groenestege, Thebault and van der Wijst 20 ).
Distribution of magnesium
Some 60–70 % of total body Mg2+ is bound in the skeleton. A further 30 % is found in the intracellular space (ICS) but only 1–5 % of intracellular Mg2+ is in the ionised form( Reference Houillier 21 ). The Mg2+ in the ECS only reflects about 1 % of total body Mg2+. Between 20 and 40 % of plasma Mg2+ is bound to albumin and globulin and some 10 % complexes with small anions such as citrate, phosphate and bicarbonate, so that 50–70 % are ionised.
According to a classical estimate( Reference Blaxter and McGill 22 ), the total content of Mg2+ within the body of calves can be calculated from:

where BW is body weight in kg.
Provided that a similar relationship holds for adult ruminants, the total body Mg2+ of a cow with a BW of 700 kg should be roughly 455 g, of which approximately 320 g would be skeletal, about 130 g intracellular, while only about 4–5 g would be found in the total ECS.
Regulation of magnesium homeostasis
The range of plasma Mg2+ primarily depends on the influx of Mg2+ from the gastrointestinal tract into the ECS (a) and on the efflux from the ECS into milk (b), into the ICS including soft tissue and bones during growth and the fetus during pregnancy (c), and into the intestine as endogenous secretion (d). Mg2+ not required for b–d is excreted into urine (e). Plasma Mg2+ concentration thus depends on the daily Mg2+ balance and is given by:

where a is Mg2+ absorption (g/d) (influx), b is Mg2+ efflux in milk (g/d), c is Mg2+ uptake (efflux) into the ICS (g/d), d is intestinal Mg2+ secretion (efflux) (g/d), and e is renal Mg2+ excretion (efflux) (g/d).
A scheme of Mg2+ metabolism for a cow with a BW of 700 kg and a milk production of 40 kg/d is given in Fig. 1.
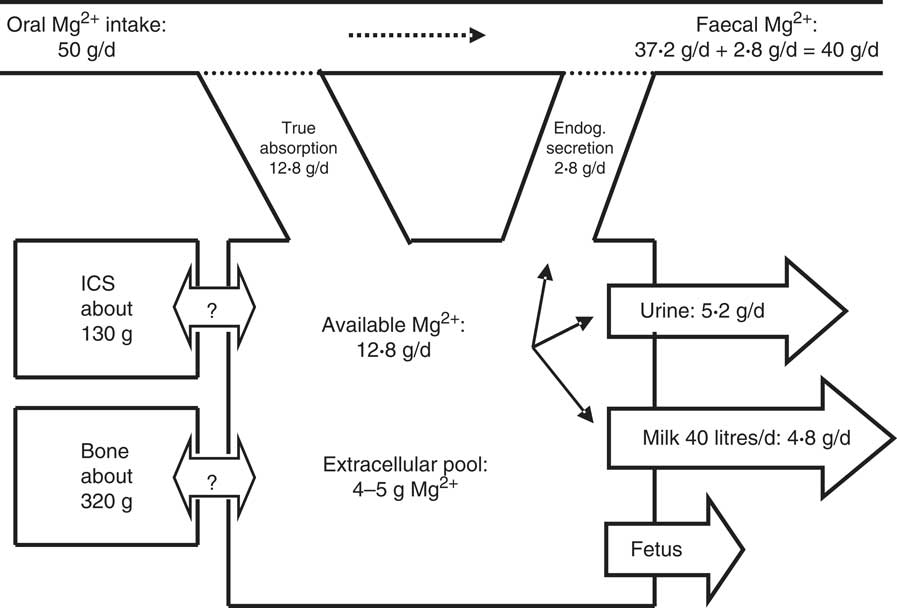
Fig. 1 Scheme of Mg2+ metabolism in a non-pregnant dairy cow of 700 kg body weight (BW). The daily Mg2+ intake is 50 g, with true Mg2+ absorption being 12·8 g/d (25·6 %). The true absorption is reduced by an endogenous (Endog.) secretion of 2·8 g/d (4 mg/kg), which accounts for an apparent absorption or Mg2+ digestion of 10 g/d (20 %). An amount of 14·8 g Mg2+/d is used for 40 kg milk secretion (120 mg/l) and the surplus (5·2 g/d) is excreted via the kidneys into urine. The pool in the extracellular space has been calculated by assuming that the plasma volume and interstitial space represent 5 and 15 % of BW, respectively, as in sheep( Reference Storry 26 ). The unidirectional flow of Mg2+ into and out of the intracellular space (ICS) and bone is not known and net flux into the ICS or bone is zero at constant BW. In pregnant cows in late gestation a flux of 0·2 g Mg2+/d towards the fetus has to be included( Reference House and Bell 207 ).
In an adult and not growing, non-pregnant cow, net uptake of Mg2+ into the ICS and bone at adequate Mg intake is marginal, so that equation (2) can be simplified to:

where a is Mg2+ absorption (g/d) (influx), b is Mg2+ efflux in milk (g/d), d is endogenous Mg2+ secretion (efflux) (g/d), and e is renal Mg2+ excretion (efflux) (g/d).
Because Mg2+ absorption (a) rarely equals Mg2+ efflux, additional mechanisms are necessary for the adjustment, which is very efficiently controlled by the kidneys at Mg2+ surplus. However, mobilisation from the skeleton or the cytosol is very limited( Reference Houillier 21 ). This suggests that Mg2+ influx was very rarely limited during evolution. Obviously, Mg2+ intake and absorption (a) were generally above requirement (b + c + d), so that an efficient renal excretion of the surplus was sufficient for the regulation of Mg2+ homeostasis (e). Moreover, Mg2+ is not very toxic, and hence transient hypermagnesaemia (rapid influx>efflux) is well tolerated( Reference Martens and Stössel 23 ). Therefore, absorption from the gastrointestinal tract is the key factor determining plasma Mg2+ levels, which can only be kept constant when the daily requirement is replaced by an adequate absorption. A better comprehension of the gastrointestinal absorption (influx) and its large variation( Reference Schonewille, Everts and Jittakhot 24 ) appears to be a key factor for understanding Mg2+ homeostasis.
Mg2+ absorption from the ruminant gastrointestinal tract
Site of magnesium absorption
Early studies in vivo suggested the distal part of the small intestine as the site of Mg2+ absorption( Reference Smith 25 ) and Storry( Reference Storry 26 ) stated that ‘there is no evidence to assume that ... a mechanism exists for the transport of calcium and magnesium across the rumen epithelium’. In contrast, Harrison et al. ( Reference Harrison, Hill and Mangan 27 ) observed Mg2+ absorption proximal to the duodenum and Marongiu from the rumen fluid( Reference Marongiu 28 ). In a later study, Rogers & van’t Klooster( Reference Rogers and van’t Klooster 29 ) measured mineral flow rates along the gut and demonstrated absorption before the duodenum. Martens( Reference Martens 30 ) summarised data from sheep and cows. The rate of absorption before the duodenum increased linearly with Mg2+ intake( Reference Martens 31 ). A net secretion was observed into the small intestine; the same amount was absorbed in the large intestine.
Regarding the location of Mg2+ absorption before the duodenum, uptake from the omasum but not from the abomasum was demonstrated( Reference Edrise and Smith 32 , Reference Field and Munro 33 ). However, Martens & Rayssiguier( Reference Martens and Rayssiguier 34 ) showed that, in vitro, the transport capacity of the rumen epithelium was large and predominant.
Physiological significance of the rumen
An important finding was that Mg2+ absorption from the forestomachs was essential for maintaining normal plasma Mg2+ and a precondition for Mg2+ homeostasis( Reference Pfeffer and Rahman 35 ). Reduced Mg2+ absorption from the forestomach was not compensated for by absorption in the intestine( Reference Tomas and Potter 36 ).
Absorption from the large intestine
Mg2+ absorption was shown to switch from the hindgut to the developed rumen after dietary transition from milk to solid feed in calves( Reference Smith 37 ) and lambs( Reference Dillon and Scott 38 ). Mg2+ absorption from the hindgut is maintained in adult animals and can be used for the treatment of acute hypomagnesaemia( Reference Meyer and Busse 39 ).
Mechanism of ruminal Mg2+ transport
Scott( Reference Scott 40 ) analysed the passive driving forces across the rumen epithelium and concluded that the chemical gradient of Mg2+ for passive movement from the rumen to plasma was opposed by the stronger electrical gradient (blood side positive 30–60 mV), which thus prevented passive paracellular uptake of the Mg2+ ion from the rumen to blood. Accordingly, Mg2+ transport across the rumen epithelium has to be energised.
The exclusion of passive paracellular diffusion suggests active, transcellular transport, which was deduced both from in vitro and in vivo experiments( Reference Martens and Harmeyer 41 – Reference Martens 43 ) showing: (a) net transport of Mg2+ from the rumen to blood; (b) saturation kinetics; (c) significantly lower transport at lower temperature; and (d) reduced transport by inhibition of Na+/K+-ATPase (ouabain or dinitrophenol). Furthermore, it was shown that ‘bulk flow’ could not explain ruminal Mg2+ transport( Reference Martens 44 ), as had been proposed for rats( Reference Behar 45 ).
The movement of Mg2+ across the multilayered epithelium includes: (a) uptake across the apical membrane; (b) the passage of Mg2+ across the various epithelial layers of the multilayered epithelium; and (c) release across the basolateral membrane. In cases where the passive gradients are sufficient, transepithelial transfer may further involve (d) possible paracellular passive movement.
Epithelial mechanisms
For many decades, characterisation of the transcellular transport pathway suffered from a lack of knowledge about Mg2+ transport. The existence of Mg2+ ion channels was still widely considered an unproven hypothesis. However, transport mechanisms for other ions (for example, Na+ or Ca2+) had been clearly established in other epithelia, such as in rabbit ileum( Reference Frizzell and Schultz 46 ). Simply put, transport of ions across epithelia is either influenced by the transepithelial potential difference (PDt) or not. By varying the electrical driving force for a specific ion (ξ)( Reference Frizzell and Schultz 46 ), and plotting the measured flux rate over ξ, it is possible to differentiate between the PD-independent flux (given by the y-intercept in the plot) and the PD-dependent flux (given by the slope of the plotted curve).
Potential-dependent Mg2+ uptake
The suggestion that Mg2+ transport occurred with the passage of a charge was deduced from a reciprocal relationship between an increase in ruminal PDt and a decrease in ruminal Mg2+ transport( Reference Tomas and Potter 36 , Reference Martens, Gäbel and Strozyk 47 ). PDt can be calculated from the apical potential difference (PDa) and the basolateral potential difference (PDb): PDt=PDa – PDb. In this relationship, the sign convention is such that the apical (ruminal) side is set to ground level and an increase in the passage of cations from the apical to the serosal (blood) side will lead to a more positive PDt and a less negative PDa, thus reducing the driving force for apical Mg2+ uptake. Mucosal to serosal Mg2+ transport rates (Jms Mg2+) revealed a linear correlation between ξ (PDt) and Jms Mg2+ within –25 and + 25 mV( Reference Leonhard-Marek and Martens 48 ). The obtained slope confirmed the suggestion of PD-dependent Jms Mg2+ transport with uptake as an ion (for example, channel-mediated), but also exhibited an intercept of the y-axis, which represents a PD-independent component (for example, via co-transport).
A PD-dependent uptake mechanism for Mg2+ in the apical membrane is supported by data from microelectrode experiments. Leonhard-Marek & Martens( Reference Leonhard-Marek and Martens 48 ) measured a PDa under open circuit conditions of –67·3 mV (cytosol negative). An increase in the mucosal K+ concentration depolarised PDa and increased PDt. These experiments suggested that the apical membrane is permeable to K+, with non-selective cation channels from the TRP family such as TRPV3 and TRPA1 likely candidates( Reference Rosendahl, Braun and Schrapers 10 ).
In further flux measurements, Mg2+ transport was reduced not only by elevation of the PDt but also by the apical K+ concentration( Reference Martens, Gäbel and Strozyk 47 , Reference Leonhard-Marek and Martens 48 ). Depolarisation of PDa by K+ is the most likely explanation for the reduced mucosal to serosal flux of Mg2+ (Jms) at high concentrations of K+ (80 mmol/l) and argues for the uptake of Mg2+ by a PD-dependent mechanism. Since the ionised intracellular Mg2+ concentration (0·54 mmol/l)( Reference Schweigel, Lang and Martens 49 ) is lower than the concentration of Mg2+ in the rumen, the uptake of Mg2+ is driven by the electrochemical gradient across the apical membrane.
The ruminal Mg2+ channel
The significant correlation between changes of PDa and Mg2+ transport led to the suggestion of an apical Mg2+ channel( Reference Martens, Gäbel and Strozyk 47 ), long before such channels were cloned. Channel-mediated transport of Mg2+ is now well established( Reference Hoenderop and Bindels 50 ). Thus, hypomagnesaemia in man is now known to be caused by the mutation of a channel of the TRP gene family, TRPM6( Reference Konrad, Schlingmann and Gudermann 51 ). TRPM6 plays a key role in the intestinal and renal absorption of Mg2+ in mice( Reference Hoenderop and Bindels 50 ). Expression of mRNA encoding for this protein by the rumen epithelium suggests a similar role in the ruminal absorption of Mg2+ ( Reference Rosendahl, Braun and Schrapers 10 ).
A further member of this channel family, TRPM7, has been demonstrated in ruminal epithelial cells as mRNA( Reference Rosendahl, Braun and Schrapers 10 , Reference Schweigel, Kolisek and Nikolic 52 ) and protein( Reference Schweigel, Kolisek and Nikolic 52 ) and is thought to play a role in intracellular Mg2+ homeostasis( Reference Demeuse, Penner and Fleig 53 ). Since experiments in TRPM7-deficient mice by Ryazanova et al. ( Reference Ryazanova, Rondon and Zierler 54 ) demonstrate disturbed intestinal Mg2+ absorption, an additional role in epithelial transport has been proposed. It has been suggested that both candidate genes are of functional importance for epithelial transport since both TRPM6 and TRPM7 subunits may be required to form a functional Mg2+ channel( Reference Dimke, Hoenderop and Bindels 55 ). MagT1 is a further candidate gene for the PD-dependent uptake pathway in ruminal epithelial cells( Reference Schweigel, Kolisek and Nikolic 52 , Reference Schweigel, Kuzinski and Deiner 56 ).
Potential difference-independent (electroneutral) Mg2+ uptake
In addition to the channel-mediated pathway, a second, PD-independent Mg2+ uptake pathway mediates Mg2+ transport( Reference Leonhard-Marek and Martens 48 ). The charge of Mg2+ is compensated by co-transport with anions or counter-transport of cations. Interestingly, the intake of high levels of readily fermentable carbohydrates( Reference Giduck and Fontenot 57 ) increased Mg2+ digestion. Furthermore, SCFA or CO2 enhanced ruminal Mg2+ absorption in vivo ( Reference Martens, Heggemann and Regier 58 ) and stimulated Jms Mg2+ in vitro ( Reference Leonhard-Marek, Gäbel and Martens 59 ). Since both fermentation products acidify the epithelium, Mg2+/2H+ exchange has been proposed to represent this transport mechanism( Reference Leonhard-Marek, Gäbel and Martens 59 , Reference Leonhard-Marek 60 ).
However, Schweigel & Martens( Reference Schweigel and Martens 61 ) found no experimental evidence for directly coupled Mg2+/2H+ exchange in isolated ruminal epithelial cells of sheep and suggested a co-transport of Mg2+ with an anion such as HCO3 – or Cl–. Furthermore, the conductance of ruminal TRP channels for monovalent cations is activated by exposure to SCFA, possibly related to swelling of the cells( Reference Stumpff, Martens and Bilk 62 , Reference Georgi, Rosendahl and Ernst 63 ). This opens the possibility that the stimulation of Mg2+ by SCFA and CO2 may not exclusively represent PD-independent Mg2+ transport but also involves stimulation of PD-dependent mechanisms. Finally, the activity of the ruminal vacuolar H+-ATPase modulates Mg2+ transport( Reference Schweigel and Martens 61 ), possibly by increasing PDa and thus enhancing the uptake of Mg2+. Such a mechanism would represent functional albeit not fixed Mg2+/H+ exchange. Currently, neither the stoichiometry nor the molecular identity of the PD-independent Mg2+ transporter is known.
Physiological consequences of two uptake mechanisms
Given that the rumen is the essential site of Mg2+ absorption under various feeding conditions, it has been proposed that both mechanisms work in parallel by ‘job sharing’ with an efficient uptake at all Mg2+ concentrations. At low ruminal Mg2+ concentrations, the PD-dependent and K+-sensitive mechanism might mediate Mg2+ transport with high affinity and low capacity. This became apparent in experiments by Ram et al. ( Reference Ram, Schonewille and Martens 64 ) and Care et al. ( Reference Care, Brown and Farrar 42 ). High ruminal K+ intake reduced Mg2+ absorption to a higher extent at low ruminal Mg2+ concentration. Consequently, a possible negative effect of K+ intake will be pronounced at high ruminal K+ (>50 mmol/l) and low ruminal Mg2+ (<2 mmol/l) concentration (see below).
Vice versa, the PD-independent and K+-insensitive mechanism has a high capacity and low affinity and will thus primarily mediate transport at high Mg2+ (>3 mmol/l) concentrations. This uptake mechanism relies exclusively on the chemical gradients of the involved ions and will rise with increasing Mg2+ concentration (Table 1).
Table 1 Characteristics of magnesium transport across the rumen epithelium

PDa, apical potential difference.
Mg2+ transport within the epithelium
The rumen epithelium is a squamous multilayered epithelium forming a functional syncytium comparable with the classical model of frog skin( Reference Ussing and Zerahn 65 ). Connections between cells of the various layers are formed by proteins such as connexin 43( Reference Graham and Simmons 66 ).
Basolateral extrusion
Mg2+ extrusion is related to the uptake of Na+. Reduction of serosal Na+ reduced Jms Mg2+ ( Reference Leonhard-Marek and Martens 67 ) and in ruminal epithelial cells, the release or uptake of Mg2+ was dependent on the direction of the Na+ gradient( Reference Schweigel, Vormann and Martens 68 ). Furthermore, application of imipramine, an inhibitor of Na+/Mg2+ exchange, reduced Mg2+ transport( Reference Leonhard-Marek, Stumpff and Brinkmann 12 , Reference Schweigel, Vormann and Martens 68 ). The characterisation of Na+/Mg2+ exchange in HEK (human embryonic kidney) cells has revealed that the human gene SLC41A1 (solute carrier family 41 member 1) encodes for this Mg2+-transporting protein( Reference Kolisek, Nestler and Vormann 69 , Reference Schweigel-Röntgen and Kolisek 70 ). The Na+/Mg2+ exchanger is indirectly energised by Na+/K+-ATPase( Reference Martens 43 ). Although evidence for the extrusion of Mg2+ from giant squid axons via Na+/Mg2+ exchange had previously been obtained( Reference Baker and Crawford 71 ), ruminants were arguably the first mammalian species in which evidence for a (secondary) active epithelial Mg2+ transport could be obtained in an essential site of Mg2+ absorption (Fig. 2).
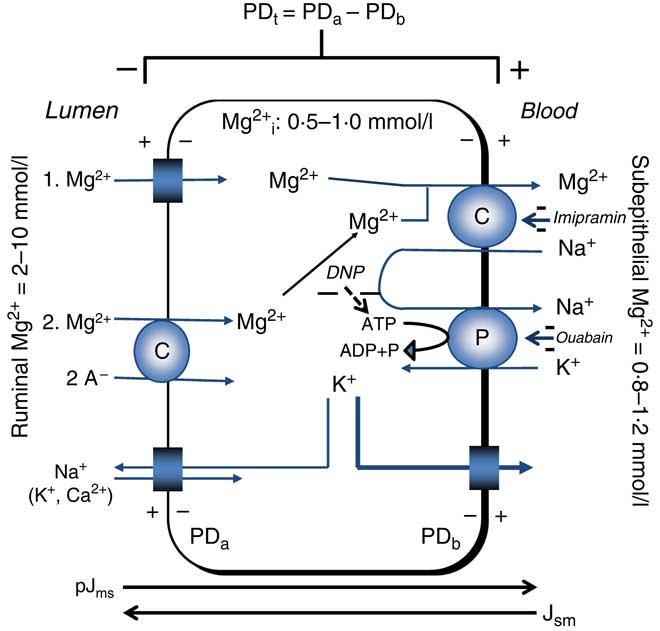
Fig. 2 Representation of transepithelial ruminal Mg2+ transport. The multi-layered epithelium is simplified to one compartment. Passive Mg2+ uptake is driven (1) mainly by the apical potential difference (PDa) or (2) by the chemical gradient of the involved free ions. The PD-dependent uptake (1) is thought to involve homo- or heteromeric assemblies of the transient receptor potential channel proteins TRPM6 and TRPM7. The molecular identity of PD-independent (2) uptake is unknown. Basolateral extrusion occurs via Na+/Mg2+ exchange via solute carrier family 41 member 1 (SLC41A1). The negative effects of inhibitors (–) on various steps of Mg2+ transport are printed in italics. pJms and Jsm represent the passive flow through the paracellular pathway. The cylindrical scheme represents a channel. PDt, transepithelial potential difference; PDb, basolateral potential difference; Mg2+ i, intracellular ionised (free) Mg2+; DNP, 2,4-dinitrophenol; A–, anion; C, carrier; P, pump (Na+/K+-ATPase). Example for PDt (+15 mV)=PDa (–45 mV) – PDb (–60 mV). Depolarisation of PDa by an increase of ruminal K+ increases PDt.
Passive paracellular Mg2+ transport
The flux of Mg2+ from the serosal to the mucosal side (Jsm Mg2+) is entirely passive( Reference Leonhard-Marek and Martens 48 ) with a permeability in the range of some 1×10–6 cm/s. This low passive flow rate limits passive transport and is unimportant under in vivo conditions.
Saturation of Mg2+ transport
Mg2+ transport saturates in vitro ( Reference Martens and Harmeyer 41 ) and in studies in vivo ( Reference Care, Brown and Farrar 42 , Reference Martens 72 , Reference Martens 73 ) and probably includes the combined transport capacities of both uptake mechanisms. However, this saturation has never been observed in conventional balance studies. Weiss( Reference Weiss 74 ) and Schonewille et al. ( Reference Schonewille, Everts and Jittakhot 24 ) analysed Mg2+ intake and digestion in cows and found a linear correlation between Mg2+ intake and Mg2+ digestion, respectively. The observed saturation under experimental conditions simulated, but very likely did not represent, the real in vivo conditions( Reference Care, Brown and Farrar 42 , Reference Martens 72 , Reference Martens 73 ). Mg2+ was almost certainly ionised in these model studies and available for transport( Reference Care, Brown and Farrar 42 , Reference Martens 72 , Reference Martens 73 ). It is to be assumed that in the normal rumen fluid, Mg2+ is only partially ionised. Indeed, chelating Mg2+ by EDTA severely depresses Mg2+ transport( Reference Leonhard, Smith and Martens 75 ).
Modulation of ruminal Mg2+ transport
In one of his first publications, Sjollema( Reference Sjollema 14 ) reported the composition of tetany-prone grass with high concentrations of K+ and N, low concentrations of Na+, while levels of Mg2+ were moderate but not low. Hence, this disease ‘does not arise by inadequate intake of Mg2+ alone’( Reference Head and Rook 76 ). Hypomagnesaemic tetany also occurred after changing the diet despite equal Mg2+ content( Reference Care, Vowles and Mann 77 ), and a decrease in plasma Mg2+ was observed even with an increase in Mg2+ intake( Reference Johnson, Helliwell and Jones 78 ). Today, there can be no doubt that various dietary factors interfere with Mg2+ transport.
The classical implications of K+
High K+ intake significantly reduced Mg2+ digestion, plasma Mg2+ concentration and, consequently, urinary excretion in sheep( Reference Fontenot, Miller and Whitehair 79 ) and cows( Reference Schonewille, Van’t Klooster and Wouterse 80 ). The reduced Mg2+ digestion was caused by a decrease in Mg2+ absorption and not by an increase in endogenous Mg2+ loss( Reference Newton, Fontenot and Tucker 81 ).
Site of K+ effect
A higher K+ intake reduced Mg2+ absorption from the forestomachs. This reduction was not compensated for in the small or the large intestine( Reference Tomas and Potter 36 ). Furthermore, K+ infusion into the abomasum or ileum did not affect Mg2+ absorption( Reference Wylie, Fontenot and Greene 82 ).
The effect of K+ and Mg2+ concentrations
There is considerable evidence showing that the effect of K+ depends on both ruminal K+ and Mg2+ concentration.
Role of K+ intake
Inhibition of Mg2+ absorption is pronounced between 1 and 3 % K+ in DM and is attenuated at higher K+ concentrations( Reference Rahnema and Fontenot 83 ). In agreement with this conclusion, Schonewille et al. ( Reference Schonewille, Ram and van’t Kloster 84 ) did not find a correlation between Mg2+ digestion and high K+ content of the diet within the range of 2·9 to 4·4 % of DM. Notably, Martens et al. ( Reference Martens, Heggemann and Regier 58 ) observed that the absorption of Mg2+ from the temporarily isolated rumen of heifers dramatically decreased between 25 and 75 mmol K+/l in the artificial rumen fluid, but not between 75 and 100 or 120 mmol K+/l. This agrees with the logarithmic relationship between mucosal K+ concentration and PDa ( Reference Leonhard-Marek and Martens 48 ).
Role of Mg 2+ intake
The proposed model of ‘job sharing’ (Table 1) of the two uptake mechanisms suggests that the effect of K+ also depends on the Mg2+ concentration. The reduction of Mg2+ absorption by K+ must be higher if Mg2+ is mainly transported via the K+-sensitive, PD-dependent mechanism. Ram et al. ( Reference Ram, Schonewille and Martens 64 ) fed sheep increasing amounts Mg2+ at two levels of K+ intake. Mg2+ absorption was reduced by 54 % at low Mg2+ intake and by 27 % at high Mg2+ intake.
The increase in K+ intake elevates ruminal K+ ( Reference Ram, Schonewille and Martens 64 ) and reciprocally decreases Na+ concentration( Reference Lang and Martens 85 ). Neither the rumen volume nor the passage rate was changed by K+ intake, excluding dilution of ruminal Mg2+ concentration or enhanced outflow( Reference Ram, Schonewille and Martens 64 ).
Meta-analysis of Mg2+ digestion: reduction by K+
The quantity of the effect of K+ on Mg2+ was analysed in a meta-analysis by Weiss( Reference Weiss 74 ) in cows, yielding the following relationship:

where digestible Mg2+ and Mg2+ intake are given in g/d, and K+ is given as % K+ in DM (thirty-nine diets, 162 cows).
Schonewille et al. ( Reference Schonewille, Everts and Jittakhot 24 ) performed a second meta-analysis with a different set of experiments and with a larger number of diets and cows, yielding:

where Mg2+ true absorption and intake are given in g/d, and K+ is given as g/kg in DM (sixty-eight diets, 323 cows).
True absorption can be transferred to apparent absorption (=digestible Mg2+) by correction for endogenous Mg2+ secretion (700 kg BW×4 mg/kg/d=2·8 g/d)( 86 , Reference Schonewille and Beynen 87 ):


Where Mg2+ (apparent absorption), digestible Mg2+ and intake are given in g/d, and K+ is given in g/kg in DM.
At a K+ concentration of 1 % in the DM, the apparent Mg2+ digestion is slightly lower (20 %) than in the calculation of Weiss( Reference Weiss 74 ) (24 %). However, Mg2+ digestion is more depressed at low Mg2+ intake.
The linear decrease in digestible Mg2+ with rising ruminal K+ (equations 4 and 7) is in contradiction to the discussed reduction of an effect of K+ at a higher Mg2+ intake. The major reason is probably the experimental design. The experiments of Ram et al. ( Reference Ram, Schonewille and Martens 64 ) and Martens et al. ( Reference Martens, Heggemann and Regier 58 ) were performed under identical conditions. Equations 4 and 5 are the result of meta-analyses of many balance studies.
The role of Na+
Insufficient Na+ intake releases aldosterone and decreases Na+ in both saliva and rumen fluid, while K+ is increased( Reference Bailey 88 – Reference Martens, Kubel and Gäbel 90 ). Accordingly, Na+ deficiency in sheep caused a decrease of Na+ in saliva and rumen fluid, an increase of K+ in both liquids, and an enhanced PDt, while Mg2+ absorption from the rumen decreased (see Table 2)( Reference Martens, Kubel and Gäbel 90 ). All of these changes were abolished by repletion of Na+. Furthermore, intravenous infusion of aldosterone in sheep caused an increase in K+ and a decrease in the Na+ concentration in the rumen. Concomitantly, ruminal Mg2+ concentration rose, while plasma Mg2+ declined( Reference Charlton and Armstrong 91 ). Since aldosterone alone does not change Mg2+ absorption( Reference Martens and Hammer 92 ), these effects are best explained by the aldosterone-induced elevation of the ruminal K+ concentration.
Table 2 Na+ deficiency and high K+ intake change the same rumen parameters and have identical effects on Mg2+ absorption

PDt, transepithelial potential difference; ↑, increase; ↓, decrease.
Notably, K+ concentration in saliva can reach some 100 mmol/l in Na+-deficient animals. Assuming a salivary flow rate of 200 litres/d, this leads to a total influx of some 780 g K+/d and presents a significant risk for reduced Mg2+ absorption. The condition is easily overlooked, because overt clinical signs of Na+ deficiency are usually missing and because the large Na+ pool in the rumen can be mobilised to cover deficiency for a long time( Reference Kemp and Geurink 93 ). Furthermore, as the K+ concentration in the rumen fluid increases, the absorption of Na+ is enhanced( Reference Lang and Martens 85 ), which may help to compensate for Na+ deficiency.
Young spring grass frequently contains extremely low concentrations of Na+ ( Reference Morris and Gartner 94 ) and was suggested as a risk factor as early as 1966 by Metson et al. ( Reference Metson, Saunders and Collie 95 ): ‘If low sodium is confirmed as yet another stress factor in the development of hypomagnesaemia, most of the present analyses [of grass] would undoubtedly qualify as tetany prone’. This suggestion is in agreement with the observation of Butler( Reference Butler 96 ) about a negative relationship between the low Na+ content of grass and the incidence of tetany. Vice versa, grass tetany caused by Na+ deficiency can be prevented by supplementation with NaCl( Reference Paterson and Crichton 97 ).
Protein and ammonia
Tetany-prone young grass in spring exhibits a high concentration of crude protein( Reference Sjollema 14 ), that causes an increase of up to 70 mmol/l ruminal ammonia( Reference Annison, Lewis and Lindsay 98 ) and is associated with grass tetany( Reference Kemp 99 ). (The term ammonia is used without discrimination between NH3 and NH4 +. Chemical symbols are used when a specification is required.) Relationships between ammonia and Mg2+ absorption have been tested with contradictory results: both inhibition of Mg2+ absorption and no effect on Mg2+ digestion at high ruminal ammonia, depending on the experimental conditions. A decrease in Mg2+ absorption was observed at a sudden increase in ruminal NH4 + concentration. Intraruminal application of large amounts of ammonium acetate in cows caused a decrease both in plasma Mg2+ concentration and urinary Mg2+ excretion( Reference Head and Rook 76 ). When working with sheep( Reference Martens and Rayssiguier 34 ) or young heifers( Reference Martens, Heggemann and Regier 58 ), respectively, Mg2+ absorption from the temporarily isolated rumen was severely reduced by increasing NH4 + concentrations which agrees with studies of the rumen pouch( Reference Care, Brown and Farrar 42 ).
However, alterations in Mg2+ metabolism were not observed in chronic experiments with a delay in sampling after raising ruminal NH4 + concentrations( Reference Moore, Fontenot and Webb 100 , Reference Gäbel and Martens 101 ). These observations led to the hypothesis that an acute increase in ruminal NH4 + reduces Mg2+ absorption, but that when ruminal NH4 + remains elevated for a period of days, an adaptational response normalises Mg2+ absorption. Gäbel & Martens( Reference Gäbel and Martens 101 ) tested this hypothesis in vivo. Acute addition of artificial rumen fluid with 40 mmol NH4 +/l into the isolated sheep rumen significantly reduced Mg2+ absorption. In balance experiments, ruminal NH4 + was rapidly increased from 4·81 (sd 0·18) to 47·9 (sd 3·1) mmol/l within 1 d. Mg2+ excretion in urine transiently decreased from 385 to 255 mg/d over 2 d, but on the 3rd day, urinary Mg2+ increased and returned to control values, despite high ruminal NH4 + (36·1 (sd 4·8) mmol/l). Obviously, a sudden change in N intake and NH4 + concentration impairs Mg2+ absorption, but adaptation occurred within 3 d.
The reason(s) for the temporary reduction of Mg2+ absorption by NH4 + have not been studied. Ammonia is transported across the rumen epithelium both as NH3 and NH4 +, depending on the pH( Reference Abdoun, Stumpff and Wolf 102 ). At a (physiological) pH of<7·0, NH4 + is predominantly transported across cation channels in the apical membrane( Reference Rosendahl, Braun and Schrapers 10 , Reference Abdoun, Stumpff and Wolf 102 ), decreasing PDa ( Reference Lu, Stumpff and Deiner 103 ) and increasing PDt ( Reference Gäbel and Martens 101 ). Since a variable fraction of the NH4 + that is taken up is extruded in the form of NH3, protons are released and decrease the cytosolic pH( Reference Rosendahl, Braun and Schrapers 10 , Reference Lu, Stumpff and Deiner 103 ), which stimulates apical Na+/H+ exchange and Na+ transport( Reference Abdoun, Stumpff and Wolf 102 ). These intraepithelial alterations of PDa and intracellular pH (pHi) offer some suggestions. Firstly, PDa changes by some 10 mV when NH4 + is elevated to 40 mmol/l( Reference Lu, Stumpff and Deiner 103 ) which may inhibit channel-mediated uptake in analogy to what has been discussed for K+. However, interactions between pHi and Mg2+ transport are a further possibility. Thus, the enhanced uptake of Na+ due to stimulation of Na+/H+ exchange( Reference Abdoun, Stumpff and Wolf 102 ) should elevate cytosolic Na+ (Na+ i), which can be expected to interfere with basolateral extrusion of Mg2+ via Na+/Mg2+ exchange. The possible mechanisms of adaptation are still unclear.
Ruminal pH
Only unbound Mg2+ in solution is available for transport across the ruminal epithelium and, accordingly, chelating Mg2+ by EDTA strongly reduces Mg2+ transport( Reference Leonhard, Smith and Martens 75 ). The range of free Mg2+ in the ruminal fluid varies from 34 to 77 % of the total amount( Reference Grace, Caple and Care 104 , Reference Dalley, Isherwood and Sykes 105 ) and depends on various factors( Reference Johnson, Helliwell and Jones 78 , Reference Dalley, Isherwood and Sykes 105 , Reference Xin, Tucker and Hemken 106 ). One major factor determining the digestion of Mg2+ is the particle size of MgO( Reference Xin, Tucker and Hemken 106 ). Furthermore, free Mg2+ concentration in the rumen depends on pH( Reference Dalley, Isherwood and Sykes 105 ). The curvilinear relationship between rumen pH and Mg2+ solubility exhibits a steep slope between pH 5 and 7, which varies with diet( Reference Dalley, Isherwood and Sykes 105 , Reference Horn and Smith 107 , Reference Johnson and Aubrey Jones 108 ) (Fig. 3). Most likely, increasing pH leads to the deprotonation of anionic binding sites in the ingested matter which are then available for binding of Mg2+. An enhancing effect of low ruminal pH on Mg2+ digestion was suggested early on by Wilcox & Hoff( Reference Wilcox and Hoff 109 ), and is probably related to an increase in unbound Mg2+. Horn & Smith( Reference Horn and Smith 107 ) found a close and negative relationship between rumen pH and Mg2+ absorption before the duodenum. The obvious effect of pH on ionised Mg2+ is very likely the major reason for the influence of the diet on Mg2+ absorption, particularly with regard to carbohydrates. A causal relationship cannot be deduced from these studies, but the pH determines Mg2+ solubility with consequences for transport.

Fig. 3 Scheme of Mg2+ solubility in rumen fluid (redrawn from Dalley et al. ( Reference Dalley, Isherwood and Sykes 105 )). The slope of Mg2+ solubility between pH 5 and 7 is influenced by the diet (←, →).
There is also reason to believe that Mg2+-transporting proteins may be affected directly by changes in pH. Thus, patch clamp studies demonstrate that the conductance of monovalent cations is enhanced by a low pH in cells overexpressing TRPM6 and TRPM7( Reference Li, Jiang and Yue 110 ). At present it is unclear if Mg2+ conductance is similarly affected. Conversely, an acidic pH has been shown to decrease the expression of TRPM6 and other Mg2+-transporting proteins( Reference Nijenhuis, Renkema and Hoenderop 111 ), which probably contributes to the renal Mg2+ wasting that is observed in metabolic acidosis in man( Reference Houillier 21 , Reference Dai, Friedman and Quamme 112 ). Interestingly, chronic usage of proton pump inhibitors impairs gastrointestinal Mg2+ absorption( Reference Atkinson, Reynolds and Travis 113 ). The possible role of ruminal pH in the aetiology of grass tetany is not clear, because both higher( Reference Horn and Smith 107 ) or lower pH has been reported( Reference Balch and Rowland 114 ). However, the close inverse correlation between ruminal pH and Mg2+ absorption before the duodenum( Reference Horn and Smith 107 ) suggests that a high ruminal pH interferes with Mg2+ digestion, particularly at low DM intake in cold weather (H Meyer, personal communication) or as a consequence of pre-existing subclinical hypomagnesaemia with plasma Mg2+ concentration ≤0·8 mmol/l and no visible clinical signs such as ataxia or muscle spasms.
Mg2+ absorption and readily fermentable carbohydrates
A low level of fermentable carbohydrates in tetany-prone grass has been suggested to decrease Mg2+ availability( Reference Metson, Saunders and Collie 95 ). Vice versa, drenching of grazing dairy cattle with a starch solution increased plasma Mg2+ concentration( Reference Wilson, Reid and Molloy 115 ) and digestion of Mg2+ ( Reference Giduck and Fontenot 57 ) although Mg2+ absorption was not consistently improved( Reference House and Mayland 116 ). In ruminal fluid, the addition of fermentable carbohydrates causes: (a) an increase in the concentration of SCFA( Reference Giduck, Fontenot and Rahnema 117 ), (b) a decrease in pH( Reference Giduck, Fontenot and Rahnema 117 ), which (c) enhances Mg2+ solubility( Reference Dalley, Isherwood and Sykes 105 ), (d) a decrease in NH4 + concentration, and (e) an increase of the number and size of rumen papilla( Reference Martens, Rabbani and Shen 118 ), with the latter increasing the area for Mg2+ absorption( Reference Gäbel, Martens and Suendermann 119 ). Hence, Mg2+ digestion was enhanced in sheep by lactose( Reference Rayssiguier and Poncet 120 ).
The exact mechanism of the stimulation of Mg2+ transport by SCFA or HCO3 –/CO2 is not clear( Reference Leonhard-Marek 60 ). Notably, addition of fermentable carbohydrates to the diet with production of SCFA enhanced Mg2+ absorption from the caecum of rats( Reference Demigné, Rémésy and Rayssiguier 121 ) and, in mice, inulin increased Mg2+ absorption and expression of TRPM6 and TRPM7 in the hindgut( Reference Rondon, Rayssiguier and Mazur 122 ). In studies with goats, Schonewille et al. ( Reference Schonewille, Ram and Van’t Klooster 123 ) have demonstrated that the depressive effect of K+ can be compensated for by the addition of fermentable carbohydrates. Various reasons for this are conceivable. Influx of protonated SCFA with subsequent dissociation can be expected to lead to cell swelling, which, in turn, enhances monovalent currents both in cells hyperexpressing TRPM7 channels( Reference Bessac and Fleig 124 ) and in native ruminal epithelial cells( Reference Stumpff, Martens and Bilk 62 , Reference Georgi, Rosendahl and Ernst 63 ). However, the most likely hypothesis is that the PD-independent pathway is stimulated by SCFA. Replacement of SCFA by gluconate significantly reduced the Jms flux of Mg2+ and reduced uptake into cells( Reference Leonhard-Marek, Gäbel and Martens 59 , Reference Schweigel and Martens 61 ). This reduction in Mg2+ transport does not reflect binding by gluconate, because gluconate only weakly binds Mg2+ ( Reference Stumpff and McGuigan 125 ), and does not affect the epithelial transport of Mg2+ ( Reference Leonhard-Marek, Marek and Martens 126 ).
Mg2+ intake and digestion
The meta-analyses of Weiss( Reference Weiss 74 ) and Schonewille et al. ( Reference Schonewille, Everts and Jittakhot 24 ) demonstrated a linear correlation between Mg2+ intake and digestible Mg2+, suggesting a constant rate of Mg2+ absorption with no adaptation. However, McAleese et al. ( Reference McAleese, Bell and Forbes 127 ) orally dosed 28Mg2+ in sheep and observed a higher 28Mg2+ absorption at deficient Mg2+ intake. In line with these findings are the results of Schweigel et al. ( Reference Schweigel, Kolisek and Nikolic 52 , Reference Schweigel, Kuzinski and Deiner 56 ): incubation of isolated rumen epithelial cells in a low- or high-Mg2+ medium caused a corresponding increase or decrease of in- and efflux mechanisms of Mg2+. Although the expression of TRPM7 was only slightly altered, both the expression of the Na+/Mg2+ exchanger( Reference Schweigel, Kolisek and Nikolic 52 , Reference Schweigel, Kuzinski and Deiner 56 , Reference Schweigel, Park and Etschmann 128 ), corresponding to SLC41( Reference Schweigel-Röntgen and Kolisek 70 ), and the Mg2+ channel MagT1 increased significantly at low Mg2+ incubation and vice versa( Reference Schweigel, Kolisek and Nikolic 52 ), supporting the assumption of the adaptation of Mg2+ transport at low Mg2+.
Allsop & Rook( Reference Allsop and Rook 129 ) suggested that Mg2+ absorption is suppressed after increasing plasma Mg2+ concentration by intravenous infusion and concluded that ‘the most probable major site of action is therefore on the uptake of Mg from the reticulorumen’. Martens & Stössel( Reference Martens and Stössel 23 ) tested this hypothesis and measured Mg2+ absorption from the isolated rumen in sheep. Mg2+ (net) transport was not influenced by increased plasma Mg2+ concentration or after 5 weeks of hypomagnesaemia, which is in contrast to the suggestion of McAleese et al. ( Reference McAleese, Bell and Forbes 127 ) and can probably be explained by the method used: sheep were orally dosed with equal amounts of 28Mg2+, and the appearance of the isotope in blood was taken as Mg2+ absorption. However, it is highly likely that the ratio between the radioactive isotope (28Mg2+) and the total concentration of Mg2+ was much higher in Mg2+-deficient sheep than in controls. Accordingly, a higher absorption of 28Mg2+ into blood could be expected even without a change of the total rate of Mg2+ – a possibility that was not considered by the authors since absorption from the rumen was not known at that time.
Endogenous Mg2+ secretion
Storry( Reference Storry 26 ) made an estimation of Mg2+ secretion in various secretions of sheep (saliva, gastric juice, bile, etc.) and estimated a daily secretion of 192 mg/d in a 40 kg sheep or 4·8 mg/kg (live weight),with similar secretion rates of 3·4 and 5·04 mg/kg found by Care( Reference Care 130 ). A significant part of the endogenous Mg2+ loss is related to high flow rates of saliva. In sheep, Dua & Care( Reference Dua and Care 131 ) estimated a secretion of about 40 % of the Mg2+ amount in the ECS or 2–3 mg/kg (live weight). This involuntary endogenous loss of Mg2+ is not constant. In sheep on an artificial, low-Mg2+ diet, secretion dropped to 0·4–1·4 mg/kg( Reference Allsop and Rook 129 ), which is probably related to the linear correlation between plasma Mg2+concentration and endogenous secretion of Mg2+ into the gut in general( Reference Allsop and Rook 132 ), and into the small intestine( Reference Martens 31 ) or the bile in particular( Reference Care 130 ).
Schonewille & Beynen( Reference Schonewille and Beynen 87 ) summarised data for the endogenous Mg2+ secretion by dairy cows (within a range from 1·5 to 6·0 mg/kg) and proposed 4 mg/kg, a value that is also used by the Gesellschaft für Ernährungsphysiologie (German Society for Nutritional Physiology)( 86 ).
Animal breeds and Mg2+ absorption
The digestion of Mg2+ in cows( Reference Greene, Baker and Hardt 133 ) and ruminal Mg2+ transport are influenced by animal breed( Reference Leonhard-Marek, Marek and Martens 126 ). Greene et al. ( Reference Greene, Baker and Hardt 133 ) have shown that Mg2+ absorption is greater in Brahman than in Jersey, Holstein or Hereford cows. Leonhard-Marek et al. ( Reference Leonhard-Marek, Marek and Martens 126 ) measured the net Mg2+ transport in vitro across isolated rumen epithelium of four breeds of sheep (Merino, Schwarzkopf, Skudde and Heidschnucke). Skudde transported significantly less Mg2+ under short-circuit conditions. The wide variation of Mg2+ digestion seen in different studies might have a genetic background and may contribute to heritability of Mg2+ in plasma( Reference Tsiamadis, Banos and Panousis 16 ). The significance of a genetic variation of Mg2+ transport proteins has been shown in man, where mutation of TRPM6 channels reduced transcellular Mg2+ transport in the intestine and kidney( Reference Hoenderop and Bindels 50 ).
Vitamin D and Mg2+ homeostasis
PTH and vitamin D3 are the principal regulators of Ca2+ metabolism. Interactions between PTH, calcitriol and Mg2+ in cows are well established( Reference Goff, Littledike and Horst 19 , Reference Schneider, Parkinson and Houston 134 , Reference Moate, Schneider and Leaver 135 ), but the results are, in some cases, contradictory( Reference Littledike and Goff 136 , Reference Hardwick, Jones and Brautbar 137 ). Calcitriol increased plasma Ca2+ and Mg2+ concentrations in hypomagnesaemic sheep( Reference Schneider, Parkinson and Houston 134 ) and Ca2+ concentration in cows, but decreased Mg2+ concentration( Reference Moate, Schneider and Leaver 135 , Reference Sansom, Allen and Davies 138 ). A calcitriol-dependent uptake of Mg2+ into soft tissue has been suggested( Reference Moate, Schneider and Leaver 135 , Reference Yano, Horiuchi and Matsui 139 ). Calcitriol did not change faecal excretion in cattle( Reference Moate, Schneider and Leaver 135 ), although calcitriol increased Mg2+ absorption from the rumen in sheep( Reference Beardsworth 140 ).
The infusion of bovine PTH in cows caused an increase in 1,25-dihydroxyvitamin D3 (1,25(OH)2D3), Ca2+ and Mg2+ in plasma and a decrease in Mg2+ in urine( Reference Goff, Littledike and Horst 19 ), indicating enhanced Mg2+ resorption in the kidney. Dua et al. ( Reference Dua, Leonhard and Martens 141 ) observed a trend for increased Mg2+ absorption from the reticulo-rumen of sheep after the onset of PTH or PTH-related protein infusions.
While interactions between the PTH and 1,25(OH)2D3 axis and Mg2+ metabolism can thus be observed, the physiological significance of this interaction is not clear. The effect of 1,25(OH)2D3 on epithelial Ca2+ transport is classical and related to increased expression and activity of TRPV5 and TRPV6 channels( Reference Hoenderop and Bindels 50 ). These channels are non-selective cation channels with a high selectivity for Ca2+ over monovalent cations. However, a certain, albeit low, permeability to Mg2+ is to be expected. In summary, the possible stimulation of Mg2+ transport by 1,25(OH)2D3 or effects of PTH should be considered as a side-effect of Ca2+ homeostasis.
Ionophores and Mg2+ digestion
Ionophores like monensin and lasalocid significantly increase Mg2+ digestion( Reference Greene, Schelling and Byers 142 ). Both ionophores lowered ruminal K+ concentrations in steers, suggesting a diminution of the reduction of K+ on Mg2+ transport.
Sequestration of magnesium
A new environment, temperature changes or prolonged transport of animals may lead to a shift in the distribution of Mg2+ from the ECS into the ICS( Reference Larvor 143 ). The stress hormone adrenaline has well-documented effects. Rayssiguier( Reference Rayssiguier 17 ) intravenously infused adrenaline in sheep and observed a rapid decline in plasma Mg2+ concentration. This decrease was blocked by the β-receptor inhibitor propranolol. Adrenaline or theophylline stimulates lipolysis and increases NEFA, as a possible cause of sequestration( Reference Elliott and Rizack 144 ). Prevention of both lipolysis and increase in NEFA by application of sodium nicotinate abolished changes in plasma Mg2+ concentration in theophylline-treated sheep( Reference Larvor and Rayssiguier 145 ). Furthermore, β-agonists such as adrenaline activate the Mg2+ channel TRPM7, stimulating uptake of Mg2+ into the cytosol( Reference Takezawa, Schmitz and Demeuse 146 ). Since TRPM7 is expressed throughout the body, a sequestration of Mg2+ into the cytosolic compartment is to be expected. The pathogenesis of transport tetany probably involves this adrenaline-dependent type of hypomagnesaemia( Reference Ali, Al-Qarawi and Mousa 147 ). It may also play a role as a secondary factor in classical grass tetany, in particular after the onset of the first clinical signs and may function as a trigger for tetanic muscle spasms.
Urinary Mg2+ excretion
Adjusted renal handling (influx ≠ efflux) is a precondition for the regulation Mg2+ homeostasis (Fig. 1) and includes two steps: filtration and re-absorption( Reference Houillier 21 ).
Mg2+ filtration
Plasma Mg2+ varies from 0·9 to 1·2 mmol/l. Some 60–80 % or 0·48–0·96 mmol/l of plasma Mg2+ is ultrafiltrable so that, on a daily basis, roughly 29–59 g Mg2+/d will appear in the glomerular filtrate of a cow of 650 kg BW (calculated with glomerular filtration rate (GFR) data of Murayama et al. ( Reference Murayama, Miyano and Sasaki 148 )). Possible effects of GFR on Mg2+ filtration are not known.
Re-absorption proximal tubule
In the proximal tubule 20–30 % of the filtered Mg2+ is reabsorbed( Reference Houillier 21 ). The fractional reabsorption rate in this part of the nephron is remarkably constant and probably occurs passively in an unregulated manner.
Re-absorption ascending limb of Henle
Most Mg2+ (60–70 %) is reclaimed in the thick ascending limb of Henle (TAL)( Reference Houillier 21 ). The paracellular and passive transport in the TAL is mainly driven by PDt (lumen positive). Energised by the basolateral Na+/K+-ATPase, this potential is generated by the apical uptake of Na+, K+ and Cl– via NKCC2 with subsequent recycling of K+ via renal outer medullary K (ROMK) channels and basolateral extrusion via ClC-Kb. Mg2+ absorption is mediated by the tight junctional channel protein, claudin-16 (paracellin-1), which interacts with claudin-19 to form a cation-selective channel( Reference Günzel and Yu 149 , Reference Konrad, Schaller and Seelow 150 ). Reduction of the passive driving force by blocking NKCC2 with furosemide( Reference Quamme 151 ) increases magnesuria in sheep( Reference Larvor and Rayssiguier 145 ). Mg2+ transport in the TAL is stimulated by PTH( Reference Shareghi and Agus 152 ) in rabbits and, accordingly, a reduced urinary excretion of Mg2+ has been found after PTH infusion in vivo in cows( Reference Goff, Littledike and Horst 19 ).
The passive transport across this pathway is regulated by Mg2+ availability. Hypomagnesaemia in mice increases both claudin-16 protein and mRNA abundance, while Mg2+-loaded animals down-regulated claudin-16( Reference Efrati, Hirsch and Kladnitsky 153 ). The expression of claudin-16 is inhibited by calcitriol( Reference Kladnitsky, Rozenfeld and Azulay-Debby 154 ) and further influenced by a variety of hormones such as glucagon, insulin, calcitonin, vasopressin or isoproterenol( Reference Quamme 155 ), which makes it difficult to evaluate these effects in vivo. Furthermore, Ca2+ transport via claudin-16 is reduced by Mg2+ ( Reference Ikari, Hirai and Shiroma 156 ): ‘A competitive transport of Mg2+ and Ca2+ via the common paracellular route in TAL could explain the coupling between Mg2+ and Ca2+ excretion’( Reference Ferrè, Hoenderop and Bindels 157 ) (see below).
The remarkable roles of NKCC2, ROMK, CIC-Kb, and claudins 16 and 19 in Mg2+ homeostasis clearly emerge from genetic studies in human subjects( Reference Houillier 21 ). Thus, Mg2+ homeostasis is severely impaired by a mutation of the claudin-16 gene( Reference Kausalya, Amasheh and Günzel 158 ). Patients with this autosomal recessive disorder suffer from hypomagnesaemia, hypermagnesuria and hypercalciuria. In Japanese black cattle homozygous deletion (not mutation) of the claudin-16 gene has been reported( Reference Ohba, Kitagawa and Kitoh 159 , Reference Hirano, Kobayashi and Itoh 160 ), with reduced renal Mg2+ clearance and reabsorption( Reference Ohba, Kitoh and Nakamura 161 ).
Re-absorption distal tubule
Approximately 5–10 % of the filtered Mg is reabsorbed in the distal convoluted tubule (DCT) via active transport. Luminal Mg2+ uptake is mediated by TRPM6, driven by PDa ( Reference Houillier 21 ). Renal TRPM6 is regulated by epidermal growth factor, which has been considered to be the first autocrine/paracrine magnesiotropic hormone( Reference Groenestege, Thebault and van der Wijst 20 ). Mg2+ deficit increases TRPM6 mRNA and protein expression in mice( Reference Groenestege, Hoenderop and van den Heuvel 162 , Reference Rondon, Groenestege and Rayssiguier 163 ). Neither PTH nor 1,25(OH)2D3 stimulated TRPM6 expression in the kidney( Reference Groenestege, Hoenderop and van den Heuvel 162 ). Interestingly, TRPM6 expression is influenced by the acid–base status of the animal. Metabolic acidosis decreases renal TRPM6 expression and thus increases Mg2+ excretion, whereas metabolic alkalosis led to the opposite effects( Reference Nijenhuis, Renkema and Hoenderop 111 ). The tight control of Mg2+ transport by TRPM6 has led to the conclusion that TRPM6 functions as a gatekeeper of Mg2+. The efflux mechanism across the basolateral membrane is still uncertain, but may involve Na+/Mg2+ exchange as in the rumen( Reference Leonhard-Marek, Stumpff and Brinkmann 12 , Reference Schweigel, Park and Etschmann 128 ) or the intestine (cyclin and CBS domain divalent metal cation transport mediator 4; CNNM4)( Reference Yamazaki, Funato and Miura 164 ).
The adaptation of Mg2+ transport in the TAL and DCT has raised questions regarding the signalling cascade. Particularly intriguing is the rapid adaptation of Mg2+ excretion by the re-absorption of almost all filtered Mg2+ under low dietary Mg2+ intake, so that plasma Mg2+ concentration is almost perfectly maintained. Because mutation of the Ca-sensing receptor (CaSR) causes disturbances of Mg2+ homeostasis in man( Reference Cole and Quamme 165 ), the CaSR is emerging as an important player in the regulation of reabsorption of both Ca2+ and Mg2+ via luminal and basolateral sensing mechanisms( Reference Houillier 21 , Reference Ferrè, Hoenderop and Bindels 157 ). More recently, Stuiver et al. ( Reference Stuiver, Lainez and Will 166 ) identified a protein (CNNM2), the mutation of which causes a disturbance in Mg2+ homeostasis. CNNM2 is located in the basolateral membrane of the TAL and DCT, and is up-regulated under Mg2+ deficiency. CNNM2 ‘might contribute to a Mg2+ sensing mechanism rather than transporting Mg2+ itself’ and should thus considered to be a Mg2+ homeostatic factor( Reference Sponder, Mastrototaro and Kurth 167 ).
Urinary Mg2+ excretion
The adaptation of renal Mg2+ transport activity in cows to various levels of intake has been illustrated by Schonewille( Reference Schonewille, van‘t Klooster and Wouterse 168 ) and Holtenius et al. ( Reference Holtenius, Kronqvist and Briland 169 ). Urinary excretion of Mg2+ rises in a quasi-exponential manner with plasma Mg2+ concentration. However, urinary Mg2+ drops rapidly with falling plasma Mg2+, but levels off at 0·61–0·73 mmol/l, after which Mg2+ almost ceases to be excreted in urine( Reference Storry and Rook 170 ). Accordingly, a dairy cow with a plasma Mg2+ concentration<0·8 mmol/l has to be considered at risk of hypomagnesaemia. This range of Mg2+ concentration appears to be a threshold. In a recent meta-analysis of Mg2+ metabolism in man, a concentration of ≥0·87 mmol/l leads to substantial urinary Mg2+ excretion( Reference Zhang, Del Gobbo and Hruby 171 ).
Urinary Mg2+ excretion is a more sensitive indicator of Mg2+ availability than the plasma concentration. Rook & Balch( Reference Rook and Balch 172 ) observed a much more pronounced decline of Mg2+ in urine than in plasma following a change in diet. The tight control of Mg2+ transport activity, particularly in the DCT( Reference Houillier 21 ) but also in the TAL( Reference Efrati, Hirsch and Kladnitsky 153 ), explains these classical observations.
The adjustment of renal Mg2+ excretion to changes in dietary intake with altered Mg2+ absorption (influx) not only ensures the maintenance of Mg2+ homeostasis in most feeding situations (Fig. 1), but also provides the practitioner with a diagnostic tool. According to the data of Kemp( Reference Kemp 173 ), Mg2+ influx can be considered to be sufficient at urinary Mg2+>4·4 mmol/l, while a range of 0·87–4·4 mmol/l might indicate a risk of Mg2+ shortage. Urinary Mg2+<1 mmol/l is probably a reliable indicator of insufficient intake/absorption.
Interaction of magnesium and calcium
Mutual interactions of transport between Ca2+ and Mg2+ have been observed( Reference Quamme 174 ). Hypercalcaemia caused a large increase in urinary Mg2+ excretion. Vice versa and again in rats, infusion of Mg2+ caused an increase of urinary Ca2+ associated with a reduction in Ca2+ uptake via TRPV5( Reference Bonny, Rubin and Huang 175 ). A mutual interaction of Ca2+ and Mg2+ has also been found in cows, with negative interactions observed both on the level of the kidney( Reference Roche, Morton and Kolver 176 ) and the rumen( Reference Care, Brown and Farrar 42 , Reference Oehlschlaeger, Wilkens and Schroeder 177 ).
Magnesium in milk
The Mg2+ concentration in milk is much higher than in plasma and exhibits a high heritability (0·60) in cows( Reference van Hulzen, Sprong and van der Meer 178 ). The higher Mg2+ concentration in milk requires active transport from plasma to milk. Nothing is known about this mechanism, which is most probably genetically determined and subject to modulation or regulation, leading to the wide variation in milk Mg2+ concentration. Cerbulis & Farrell( Reference Cerbulis and Farrell 179 ) analysed Mg2+ in the milk of different breeds with a range of 99–120 mg/l, with one cow at 268 mg/l. The average concentration of Mg2+ in the milk of all animals was 112 mg/l, close to the recommendation of Schonewille & Beynen( Reference Schonewille and Beynen 87 ) of 120 mg/l. Assuming a milk yield of 30–40 litres/d, a cow will lose some 3–5 g Mg2+/d, which approaches the total amount of Mg2+ in the ECF (see Fig. 1). It is important to realise that Mg2+ efflux via milk is continued probably with some (genetic) variation even in hypomagnesaemic cows( Reference Rook and Storry 180 ) so that excretion of Mg2+ in milk exacerbates Mg2+ deficiency.
Goff & Horst( Reference Goff and Horst 181 ) suggest that the concentration of Mg2+ in colostrum is 100 mg/l, although higher values of 238–322 mg/l were found by Shappel et al. ( Reference Shappell, Herbein and Deftos 182 ) on the day of parturition in heifers and cows, with a rapid and exponential decline postpartum within 2 to 3 d to the normal level of 120 mg/l. The total amount in colostrum on the day of parturition amounted to 1·57–4·97 g/d. The rapid change of Mg2+ in milk after parturition probably explains the much higher concentration of Mg2+ in early colostrum( Reference Kehoe, Jayarao and Heinrichs 183 ). Kehoe et al. ( Reference Kehoe, Jayarao and Heinrichs 183 ) reported 733 mg/kg (range 230–1399 mg/kg) in the colostrum of fifty-five fully milked out cows from different herds within 4 h of calving. Assuming a volume of 5 litres yields a rough estimate of 3·6 g Mg2+ excretion in colostrum results, which underlines the significant Mg2+ demand at parturition.
Magnesium and tetany
Plasma Mg2+ and tetany
Sjollema( Reference Sjollema 13 , Reference Sjollema 14 ) first demonstrated the relationship between the clinical symptoms of grass tetany and hypomagnesaemia. However, the Mg2+ concentration in the plasma of afflicted animals exhibits some variation (Table 3), and the severity of the nervous disturbances is not closely related to the plasma Mg2+ concentration( Reference Halse 184 ). Possibly, the speed of plasma Mg2+ decline promotes the onset of clinical manifestations( Reference Allcroft and Burns 185 ).
Table 3 Status of Mg2+ metabolism and plasma Mg2+ concentration
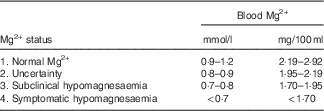
At values below 0·9 mmol/l, both an adequate supply of Mg2+ or impending clinical hypomagnesaemia are possibilities, so that a safe assessment of Mg2+ status should involve a determination of urinary Mg2+ excretion. Even then, difficulties in judging Mg2+ status can be clearly seen in a study involving non-pregnant lactating cows with normal Mg2+ intake (29–32·5 g/d) and plasma Mg2+ concentration of 0·75–1·1 mmol/l( Reference Schweigel, Voigt and Mohr 186 ). After intravenous infusion of Mg2+ and despite a slight increase in plasma Mg2+ in four of the nine animals, the fractional renal Mg2+ excretion decreased, indicating Mg2+ retention after the Mg2+ load and pointing towards a possible Mg2+ deficit. Despite these uncertainties, low plasma Mg2+ concentrations almost invariably precede the onset of neurological symptoms with impaired function of the CNS.
Clinical hypomagnesaemia
Classical hypomagnesaemic tetany was originally observed a few days after cows had been let out to graze in spring( Reference Blaxter and McGill 22 ). At first sight, it appears surprising that the relatively large Mg2+ pools in the ICS (130 g) or bones (about 320 g) of cattle cannot acutely be mobilised to maintain physiological plasma Mg2+ ( Reference Blaxter and McGill 22 ), although a small mobilisation of 0·5 g/d has been reported in cows( Reference Storry and Rook 170 ), comparable with observations in human subjects( Reference Houillier 21 ). Mobilisation of Mg2+ from bone is unlikely, because the ratio between Ca2+ and Mg2+ in bone is 42 to 1, and substantial withdrawal from bone would disrupt Ca2+ homeostasis( Reference Fontenot, Allen and Bunce 187 ). Furthermore, both PTH secretion and sensitivity of bone to PTH are decreased under conditions of hypomagnesaemia or alkalosis( Reference Kopic and Geibel 188 ). Cytosolic Mg2+ is only partly available for redistribution too; only 1–5 % is available in the ionised form with the rest bound primarily to ATP or sequestered in microsomes and mitochondria( Reference Houillier 21 ). Accordingly, a massive efflux of Mg2+ from the cytosol into the ECS might interfere with cellular energy metabolism and cellular enzyme function.
Impaired function of the central nervous system
Hypomagnesaemic tetany is observed frequently as plasma Mg2+ drops below 0·7 mmol/l( Reference Meyer 189 ) and was originally suggested to be caused by impaired synaptic transmission at the motoric endplate( Reference Hemingway and Ritchie 190 ). This hypothesis was not confirmed by Todd & Horvath( Reference Todd and Horvath 191 ). The possible involvement of the CNS was first discussed by Chutkow & Meyers( Reference Chutkow and Meyers 192 ) at low Mg2+ concentrations in the cerebrospinal fluid (CSF) of Mg2+-deficient rats. The hypothesis of a decreased Mg2+ concentration in the CSF as a reason for clinical signs was tested by Meyer & Scholz( Reference Meyer and Scholz 193 ) in Mg2+-deficient sheep by measuring the Mg2+ concentration in plasma and CSF. They found that while the Mg2+ concentration in the CSF is kept constant over a wide range of plasma Mg2+ concentrations, it begins to decrease at plasma levels<0·5 mmol/l so that at<0·25 mmol/l, Mg2+ in CSF decreases almost linearly with the concentration in plasma. Allsop & Pauli( Reference Allsop and Pauli 194 ) further tested the discussed causal correlation between Mg2+ in CSF and clinical signs. Mg2+ concentrations of<0·25 mmol/l in the solution of CSF perfusion produced episodes of tetany that were abolished by higher Mg2+ concentrations. Because these effects were not accompanied by changes in blood parameters, the clinical symptoms were considered to be caused by the non-controlled activation of muscles by processes within the CNS. However, little is known about the regulation of Mg2+ in the CSF. After rectal infusion of MgCl2, Reynolds et al. ( Reference Reynolds, Bell and Sims 195 ) observed that the Mg2+ concentration in the CSF remained constant in calves with normal plasma Mg2+, while in calves with subnormal plasma Mg2+ (<0·75 mmol/l), an increase in Mg2+ was observed in the CSF with a delay up to 120 min. These results suggest carrier-mediated transport into the CSF and might explain why a rapid decline of plasma Mg2+ causes a fall of Mg2+ in the CSF, whereas a slow decrease allows for sufficient Mg2+ transport into the CSF. This conclusion agrees with the observation of Allcroft & Burns( Reference Allcroft and Burns 185 ) who suggested that the speed at which plasma Mg2+ level decreases is critical for triggering clinical symptoms.
There are a number of reasons why a drop of Mg2+ in the CSF might trigger hyperexcitability. Mg2+ is a physiological antagonist of Ca2+-induced transmitter release at synapses( Reference Thomson 196 ), and low Mg2+ in the CSF might facilitate Ca2+-dependent transmitter release and the excitation of CNS neurons that, amongst others, activate muscles. The activity of the glutamatergic NMDA receptor (N-methyl-d-aspartate) in the CNS is inhibited by external Mg2+ in a PD-dependent manner and at low Mg2+ in the CSF, more receptors are activated, which should result in hyperexcitability( Reference Morris 3 , Reference Mayer, Westbrook and Guthrie 197 ). Furthermore, the activity of the inhibitory γ-aminobutyric acid (GABA) receptor is enhanced by Mg2+. Conversely, the inhibitory effects of GABA are reduced when Mg2+ falls, facilitating neuronal activation( Reference Möykkynen, Uusi-Oukari and Heikkila 4 ). Hence, a decrease of Mg2+ in the CSF induces hyperexcitability of excitatory neurons (NMDA) while reducing activity of inhibitory neurons (GABA).
The effect of Ca2+ concentration in the CSF on the onset of clinical symptoms is still controversial. Reynolds et al. ( Reference Reynolds, Bell and Sims 195 ) and Allsop & Pauli( Reference Allsop and Pauli 194 ) observed diminished Mg2+ and Ca2+ concentrations in the CSF. However, plasma Ca2+ concentration did not correlate with clinical symptoms in sheep( Reference Meyer 189 ).
Subclinical hypomagnesaemia
It is important to note that the appearance of clinically relevant neurological symptoms is not obligatory, even when plasma levels of Mg2+ are low. Hypomagnesaemia of about 0·5 mmol/l was induced in sheep by feeding a low-Mg2+ diet for 5 weeks without appearance of any neurological symptoms( Reference Martens and Stössel 23 ). It is very likely that Mg2+ concentration in the CSF is maintained when the induction of hypomagnesaemia with a low-Mg2+ diet is gradual (see above). It should be noted that even in the absence of clear neurological symptoms, animals may suffer from various non-neurological manifestations of hypomagnesaemia.
Interactions between hypomagnesaemia and the regulation of Ca2+ metabolism were observed early on. Thus, Allen et al. ( Reference Allen, Sansom and Davies 198 ) showed a correlation between subclinical hypomagnesaemia and the occurrence of milk fever with plasma Mg concentrations of<0·8 mmol/l. Subclinical hypomagnesaemia has a negative effect on the release of PTH( Reference Littledike and Goff 136 , Reference Anast, Mohs and Kaplan 199 , Reference Rayssiguier, Garel and Davicco 200 ), the functioning of PTH on the target organ( Reference MacManus, Heaton and Lucas 201 , Reference Goff 202 ) and the conversion of 25(OH)D3 to 1,25(OH)2D3 (calcitriol)( Reference Horsting and DeLuca 203 ). Moreover, in organ cultures of fetal rat bone, the release of Ca by supplementing 1,25(OH)2D3 or PTH was reduced at low (<0·8 mmol/l) Mg concentration( Reference Johannesson and Raisz 204 ). Furthermore, regulation of Ca homeostasis was found to deteriorate with induction of secondary hypocalcaemia in calves with hypomagnesaemia( Reference Rayssiguier, Garel and Davicco 200 ). These results correspond very well with in vivo observations of Sansom et al. ( Reference Sansom, Manston and Vagg 205 ), who found that the mobilisation of Ca from bone was lowered significantly in cows with hypomagnesaemia. A subsequent study by van de Braak et al. ( Reference van de Braak, van’t Klooster and Malestein 206 ) confirmed these findings.
Conclusions and perspectives
A correlation between the clinical symptoms of ‘grass staggers’ or ‘grass tetany’ and hypomagnesaemia more than 80 years ago initiated myriads of studies about Mg2+ metabolism in ruminants. These studies led to a stepwise improvement in understanding the pathogenesis: (a) hypomagnesaemia was not caused by a Mg2+-deficient diet, but by reduced availability from the diet; (b) the site and mechanisms of Mg2+ absorption were described; and (c) the factors that influence Mg2+ transport and digestion were characterised. Despite a considerable increase in knowledge about the pathogenesis and prevention of hypomagnesaemic tetany, many open questions remain. Further work is necessary to identify if channels other than TRPM6, TRPM7 or MagT1 contribute to PD-dependent uptake of Mg2+ in the rumen. In particular, the role of various other TRP channels expressed by the rumen has to be clarified( Reference Rosendahl, Braun and Schrapers 10 ). The PD-independent uptake mechanism is still not well characterised and its molecular identity is unknown( Reference Leonhard-Marek 60 , Reference Schweigel and Martens 61 ). Furthermore, the antagonism between Mg2+ and Ca2+ in the gut and the kidney deserves attention. In particular, renal excretion in vivo is of major interest, because renal Mg2+ transport is regulated according to the Mg2+ requirement. A better understanding of this mechanism could lead to improved diagnosis of the Mg2+ status of cattle.
Acknowledgements
The studies of the authors were supported by the Deutsche Forschungsgemeinschaft (DFG). The manuscript was written by H. M., and was improved and optimised by F. S., S. L.-M. and M. R.
There is no conflict of interest.