I. INTRODUCTION
With the rapid consumption of fossil fuels for energy demands and increasing environment crisis, an urgent demand for clean energy sources have attracted a wide horizon of research interest over the past few years. Hydrogen has been considered an ideal and sustainable alternative source for fossil fuels for the human society owing to the highest possible energy density, renewable, and zero greenhouse gases emission properties. Reference Chow, Kopp and Portney1–Reference Li, Yu, Low, Fang, Xiao and Chen3 Among the clean-energy developing methods, electrochemical splitting of water to produce hydrogen is a promising strategy due to its low energy consumption, short reaction period, high-purity product, and environmentally friendly process. However, such methods need to seek highly efficient hydrogen evolution reaction (HER) electrocatalysts that can provide a large mediation for the reaction at a low overpotential, Reference Wang, Zhou, Li and Shu4–Reference Wang, Cui, Liu, Xing, Asiri and Sun6 which represents a vital step to accelerate the development of hydrogen economy but remains challenging for now. Reference Shi and Zhang7
Over the recent years, great efforts have been made to explore the high efficiency, low cost, and strong durability of nonprecious metal substitutes. Reference Han, Fang, Jing, Sun, Lei, Lai and Cao8–Reference Lin, Chiu, Wu, Hsieh and Wu10 As yet, many nonprecious metals, Reference Fan, Liu, Yan, Gu, Yang, Yang, Qiu and Yao11,Reference Liu, Zhang, Xiong and Ma12 transition metal alloys, Reference Lu, Hutchings, Yu, Zhou, Forest, Tao, Rosen, Yonemoto, Cao, Zheng, Xiao, Jiao and Chen13–Reference Zeng, Liu, Zhao, Nie, Han, Wang, Huang, Song, Zhong and Li16 transition-metal carbides, Reference Li, Wang, Liu, Li, Wang, Dong, Dai, Li and Lan17–Reference Gong, Wang, Hu, Zhou, Feng, Duchesne, Zhang, Chen, Han, Li, Jin, Li and Lee19 chalcogenides, Reference Chang, Hai, Pang, Zhang, Shi, Liu, Liu, Zhao, Li and Ye20–Reference Yu, Feng, Jeon, Guan and Lou24 nitride, Reference Yan, Tian, Wang, Wu, Meng, Zhao and Fu25–Reference Zhang, Xie, Zhou, Wang and Pan27 oxide Reference Yan, Tian, He and Chen28–Reference Wu, Zhang, Shi, Liu and Zhang30 and borides Reference Masa, Weide, Peerers, Sinev, Xia, Sun, Somsen, Muhler and Schuhmann31 have been widely investigated for their electrocatalytic HER properties. Even though a great deal of research work has been put in during the past few decades, the HER catalysts still face several challenging problems: (i) the catalytic performance of the most reported HER electrocatalysts are much lower than the Pt/C catalysts and need to be further promoted; (ii) some electrocatalysts that show good HER activities are still dependent on the assistance of noble metal materials; (iii) the hybrid catalysts with the combination of two or more phases cannot easily maintain their activities due to complicated components and structures; (iv) many electrocatalysts suffer from the bad stability toward HER. Recently, metal phosphides-based composites (MPCs), which are known as the alloy of phosphorus and a transition metal, showing metallic and submetallic properties (such as the high electron transfer and small or no band-gap energy) Reference Pi, Wu, Zhang, Chen and Wang32 have been discovered as excellent HER catalysts, such as Mo-, W-, Fe-, Co-, Ni-, and Cu-based phosphides. Reference Tian, Yan, Chen, Liu and Chen33–Reference Han, Zhang, Yuan, Ji and Du38 Among these, Cu3P is usually investigated as the electrode material for lithium-ion batteries in early articles. Reference Ni, Ma, Lv, Yang and Zhang39,Reference Zhou, Yang, Wang, Dai, Zhao, Xue, Han, Fan and Li40 Very recently, Sun and co-workers proposed the preparation of self-standing Cu3P nanowire arrays on commercial porous copper foam as a monolithic high-efficient three-dimensional (3D) cathode for electrocatalytic hydrogen evolution with long-term stability. Reference Tian, Liu, Cheng, Asiri and Sun41 Shen and his group reported synthesis of Cu3P nanocubes and developed it as an excellent catalyst for electrocatalytic hydrogen generation in acidic solution. Reference Ma, Shen, Zhou, Zhu, Xi, Ji and Kong42 Chen et al. developed Cu3P nanosheet as an efficient and robust bifunctional catalyst for electrocatalytic overall water splitting. Reference Hou, Chen, Wang, Liang, Lin, Fu and Chen43 However, except for the above reports, the study of Cu3P as an electrocatalyst for hydrogen evolution comprises only few studies up to now.
For catalysts of HER, besides chemical constituents and morphology characters, electric conductivity also plays an important role in their electrochemical performance. Reference Xing, Liu, Asiri and Sun44 Despite that most of the MPCs possess metallic and metalloid features, it is still necessary to increase the electronic conductivity for the catalysts by using the assisted method, because there are contact resistances between the catalytic active materials. Such strategies to fabricate the catalysts’ nanostructure arrays on a carbon fiber cloth (e.g., the WP2 nanowire arrays on carbon cloth as a 3D flexible electrode for efficient electrocatalytic hydrogen evolution) Reference Pi, Wu, Zhang, Chen and Wang45 or commercial porous metal foam (e.g., electrodeposition of Ni–P nanoparticles film on copper foam as a bifunctional electrocatalyst for water splitting to produce hydrogen) Reference Liu, Gu and Li46 are promising approaches to guarantee rapid electron transport for electrochemical reactions. In addition, hybridization with carbon is another efficient strategy to increase the conductivity of active materials. For example, Zhang et al. and Wang et al. reported carbon-coated Fe3O4 nanospindles Reference Zhang, Wu, Hu, Guo and Wan47 and nanorings Reference Wang, Liang, Zhu, Mei, Zhang, Yang and Qian48 as electrode materials for lithium-ion batteries, respectively. Gao et al. reported the preparation of carbon-coated MoP nanoparticles as efficient hydrogen evolution electrocatalyst. Reference Gao, Liu, Li, Guo, Zou and Zou49 Compared with the counterpart without the carbon coating, all of them show an enhanced performance on account of the carbon decoration, which effectively increases the electron transport of the catalysts. At the same time, the carbon decoration of the catalyst can also protect the catalyst from corrosion of the electrolyte solution, which can further enhance the stability of the catalyst.
In this work, we report a facial one-pot synthesis strategy to prepare in situ carbon-decorated Cu3P particles (Cu3P@C) via rapid reaction of the precursors of copper acetylacetonate [Cu(acac)2] and triphenylphosphine (PPh3) at 425 °C for 1 h with the assistance of a vacuum system. As far as we know, this is the first time the Cu3P@C hybrid has been synthesized in such a short reaction time under mild conditions. As a catalyst for electrochemical hydrogen evolution, the Cu3P@C hybrid was investigated in the current work. Compared with pure Cu3P particles, the Cu3P@C hybrid shows an enhanced electrocatalytic performance for hydrogen evolution with excellent stability. It is evidenced that the in situ decoration of carbon efficiently facilities and ensures a fast electron transport for the electrochemical reaction. The results of our study make the present Cu3P@C-based hybrid a promising catalyst for practical applications toward energy conversion and pave a way for designing and fast fabricating in situ carbon-decorated HER catalysts from the organometallic reaction precursors.
II. EXPERIMENTAL SECTION
A. Materials
Copper acetylacetonate [Cu(acac)2], copper (Cu) nanoparticles, and triphenylphosphine (PPh3) were purchased from Alfa Aesar (Shanghai, China). Commercially available Pt/C (20%) catalyst and Nafion solution (5 wt%) were purchased from Shanghai Chemical Regent Company (Shanghai, China). The ethanol and toluene solvents were obtained from Adamas-beta (Shanghai, China). All the chemicals were used as received, without further purification. The deionized water used in our experiments was purified using a Millipore system (Millipore, Milli-Q Advantage A10, Shanghai, China).
B. Synthesis of Cu3P@C-based hybrid catalyst
The Cu3P@C-based hybrid catalyst was synthesized via one-pot liquid-phase phosphidation strategy with the assistance of a vacuum system. In a typical synthesis: 30 mg of Cu(acac)2 and 180 mg of PPh3 were uniformly mixed and encapsulated in a vacuum (∼3 × 10−4 Pa) quartz tube. Afterward, the sealed tube was loaded into a muffle furnace, which was then heated from room temperature to 425 °C in 213 min, and kept at this reaction temperature for 1 h. After that, the tube was cooled down to room temperature naturally, the black products were collected from the silica tube, then the product was repeatedly washed by toluene and absolution ethanol with ultrasonic treatment, and finally dried at 60 °C in a vacuum oven for further investigation.
C. Synthesis of Cu3P catalyst
For comparison, the Cu3P nanoparticles without carbon decoration were also prepared by directly phosphating Cu nanoparticles using a similar phosphidation reaction process: 32 mg of Cu and 180 mg of PPh3 were uniformly mixed and encapsulated in a vacuum (∼3 × 10−4 Pa) quartz tube. Afterward, the sealed tube was loaded into a muffle furnace, which was then heated from room temperature to 400 °C in 200 min, and kept at this reaction temperature for 20 h. After that, the tube was cooled down to room temperature naturally, the black products were collected from the silica tube, the product was then repeatedly washed by using toluene and absolution ethanol with ultrasonic treatment, and finally dried at 60 °C in a vacuum oven for further investigation.
D. Characterizations
The purity and phase of the samples were identified using the X-ray diffractometer (XRD) data collected by the powder XRD (Rigaku D/MAX2500PC, Rigaku Corporation, Beijing, China) with Cu Kα radiation (1.54178 Å). The field-emission scanning electron microscopy (FE-SEM, TESCAN MIRA 3, Boyue Instrument Ltd, Shanghai, China) was utilized for morphological and structure observation with an accelerating voltage of 25 kV, and the corresponding energy dispersive X-ray (EDX) spectrum of the samples were obtained by using a matched X-ray spectrometer for the FE-SEM. Measurements were also performed using the transmission electron microscopy (TEM; Hitachi, Tokyo, Japan) to further reveal the microstructure of the obtained products. The specific surface area was tested by using an automated surface area and pore size analyzer (Quadrasorb 2MP, Quantachrome Instruments, Boynton Beach, Florida). The Raman spectrums were taken under ambient conditions by using a Raman spectrometer (Labram HR 800 Raman, Horiba Corp, Shanghai, China). The X-ray photoelectron spectra (XPS) characterizations were performed on a Thermo ESCALAB 250Xi X-ray photoelectron spectrometer (Thermo Fisher Scientific, Waltham, Massachusetts) with Al Kα X-ray sources.
E. Electrochemical measurements
All the electrochemical characterizations were performed by using an electrochemical workstation (CHI 660E; CH Instruments, Shanghai, China) at room temperature in a typical three-electrode setup, and 0.5 M H2SO4 was utilized as the electrolyte solution. The graphite rod was used as the counter electrode, and saturated calomel electrode (SCE) was used as the reference electrode. A piece of carbon fiber paper (CFP) coated with the Cu3P-based catalyst was used as the working electrode. The fabrication process for the working electrode is described as follows: 10 mg of the Cu3P-based catalyst was dispersed in 500 μL of water–ethanol solution (with volume ratio of 300:190) containing 10 μL of 5 wt% Nafion solution by ultrasonic treatment for several hours to form a homogeneous ink. Afterward, a certain volume of the catalyst ink was loaded onto the CFP substrate (catalyst loading density ∼1 mg/cm2). Here, the CFP substrate was cleaned by repeat ultrasonic treatment for 10 min with deionized water, acetone, and ethanol solutions, respectively. The potential measured by the SCE reference electrode in our work was converted to potential against reversible hydrogen electrode (RHE) according to the equation: E RHE = E SCE + 0.242 + 0.059 pH, and the iR compensation was also applied to all initial data for further analysis. For linear sweep voltammetry (LSV) tests, the sweeping potential range is −0.6 to 0 V (versus RHE) in 0.5 M H2SO4, and the scan rate was set to be 2 mV/s. The electrochemical impedance spectroscopy (EIS) tests were measured at −0.1 V (versus RHE) within the frequency ranging from 100 kHz to 0.01 Hz. The cyclic voltammetric (CV) measurements were conducted from +0.1 to −0.3 V (versus RHE) at a scan rate of 100 mV per second. The time dependency response of catalytic current density during electrochemical reaction for the Cu3P@C hybrid catalyst was carried out at a constant potential of 205 mV (versus RHE) after equilibrium.
III. RESULTS AND DISCUSSION
The approach of reacting organometallic precursors with PPh3 in an evacuated and sealed silica tube has been confirmed as an efficient method to prepare the MPC catalytic materials. The high boiling point of PPh3 makes it possible to synthesize MPC products via a solution-phased route during the reaction, and the graphitization of the carbon species from the organometallic sources can in situ decorate the MPCs. Reference Jiang, Wang, Li and Yang50 In this work, by reacting Cu(acac)2 with PPh3 in the vacuum and encapsulated quartz tube, the Cu3P@C hybrid was successfully synthesized. The reaction process schematic diagram of the Cu3P@C product is shown in Fig. 1(a); the thermal decomposition of organometallic compound Cu(acac)2 provided the carbon and copper sources while PPh3 acted as the phosphorus source and reacted with the newly born copper species to produce the new type of transition-metal phosphide/carbon hybrid product, and the Cu3P@C hybrid shows a specific surface area of 122 m2/g with a wide pore size distribution from 2 to 10 nm (Fig. S1). Additionally, the Cu3P nanoparticles without the carbon decoration for the comparison were also prepared by directly phosphating Cu nanoparticles using the same phosphidation procedure. Cu3P is a well-known form of copper phosphide, and Fig. 1(b) shows its crystal structure. Cu3P crystallized in the hexagonal type with space group of P63 cm, and the corresponding lattice constants are a = 6.958, b = 6.958, and c = 7.178 Å, respectively. The crystal structure and phase purity of the obtained Cu3P@C and Cu3P samples were characterized by powder XRD. The XRD patterns of the as-prepared products, as shown in Fig. 1(c), can be accurately indexed to the typical CuP3 phase (JCPDS card no. 71-2261), indicating that the CuP3-based catalysts are successfully prepared.

FIG. 1. (a) Synthesis process of the Cu3P@C product. (b) Crystal structure of the Cu3P (purple ball—copper atom, pink ball—phosphorus atom). (c) XRD patterns for the Cu3P and Cu3P@C samples.
FE-SEM was used to observe the morphologies of the as-prepared samples. It can be seen from Figs. 2(a) and 2(b) that the sample consists of microparticles coated with sheet-like materials, and the TEM image [Fig. 2(c)] further reveals such hierarchical structure for the Cu3P@C hybrid. Figure S2 shows the SEM and TEM images of pure Cu3P, which indicates that the pure Cu3P products are the nanoparticles with irregular morphology. EDX spectra of the sample in Fig. 2(d) demonstrates that it is composed of C, Cu, and P, and the atomic ratio for Cu and P is about 3.3:1, which is close to the expected stoichiometry for the Cu3P. Furthermore, as shown in Fig. 2(e), the SEM image and the corresponding EDX elemental mapping images for the sample indicate that the Cu and P elements are evenly distributed in the Cu3P@C hybrid, and the carbon coats on them. In addition, the Raman spectra [Fig. 3(a)] of the Cu3P@C sample shows two intensive peaks with wave numbers of 1346 and 1585 cm−1. The D-band at about 1346 cm−1 is attributed to the vibration of carbon atoms with dangling bonds in the plane termination of disordered graphite, whereas the G-band at 1585 cm−1 is associated with the vibration of sp 2 hybridized carbon atoms in a graphite layer, Reference Lee, Wei, Kysar and Hone51 which further confirms the presence of carbon in the Cu3P@C hybrid. The disorder degree of carbon is in proportion to the relative intensity ratio of the two bands (I D/I G), and the low I D/I G ratio implies the high graphitization of carbon, which can further promote the charge transfer for the electrochemical reaction. Reference Chen, Qi, Guo, Lei, Zhang and Cao26 However, there are no characteristic peaks of graphite for the pure Cu3P nanoparticles. All of the above results strongly verify the successful preparation of carbon-decorated Cu3P particles.

FIG. 2. (a) Scanning electron microscopy (SEM), (b) magnified SEM, and (c) TEM images for the Cu3P@C hybrid sample. (d) EDX spectra and (e) SEM image and the corresponding EDX elemental mappings of C (red), P (blue), and Cu (green) for the Cu3P@C hybrid sample.

FIG. 3. (a) Raman spectrums for the Cu3P and Cu3P@C hybrid. (b) XPS survey spectrum for the Cu3P@C hybrid. XPS spectra in the P 2p (c) and Cu 2p (d) regions for the Cu3P@C hybrid.
To further explore the chemical composition of the prepared Cu3P@C hybrid, the XPS test was performed. Figure 3(b) shows the XPS survey spectra, and the Cu3P@C sample can be obtained that only contains P, Cu, C, and O. The signal of O could be originated from the adsorbed oxygen, oxygen-containing groups, and surface oxidation of the sample in air. The strong peak of C also confirms the existence of carbon in the Cu3P@C hybrid. Figures 3(c) and 3(d) show the magnified close-up and fitted spectra in the P and Cu regions for the Cu3P@C hybrid, respectively. As shown in Fig. 3(c), the P 2p region shows two peaks at 129.6 and 130.3 eV, reflecting the binding energy (BE) of P 2p 3/2 and P 2p 1/2, respectively. The primary P 2p 3/2 at 129.6 eV comes from the P–Cu bond. Here, the peak at 134.2 eV can be attributed to the oxidization of P species as a result of air contact. In the Cu region [Fig. 3(d)], the peak at the BE of 932.9 and 952.7 eV are indexed to Cu 2p 3/2 and Cu 2p 3/1, respectively. According to an early report, both of the hydrogenase and metal complex toward HER combine proton relays from pendant acid–base groups near the metal center. Reference Wilson, Shoemaker, Miedaner, Muckerman, Dubois and Dubois52,Reference Sun, Han, Lei, Chen and Cao53 For WP2 Reference Pi, Wu, Zhang, Chen and Wang54,Reference Pi, Wu, Guo, Wang, Zhang, Wang and Chen55 and MoP2 Reference Zhu, Tang, Liu, Wang, Asiric and Sun56 hydrogen evolution electrocatalyst, they are also characterized by pendant base P (δ−) near the metal center M (δ+). For elements Cu and P, the BEs are 932.6 eV and 130.2 eV, Reference Moulder, Chastain and King57 respectively, therefore the BE of P 2p 3/2 shifts to low energy and that of Cu 2p 3/2 exhibits a positive shift, indicating that the P site has a partial negative charge (δ−) and the Cu site has a partial positive charge (δ+) in the Cu3P@C hybrid catalyst, then the electron density will have a small transfer from Cu to P, which is the ligand effect, Reference Pu, Liu, Asiri and Sun58,Reference Chen, Qi, Zhang and Cao59 and it is desirable for an excellent performance HER electrocatalyst. On the base of the above XPS analysts, it can be deduced that the Cu3P catalyst may follow a similar catalytic reaction principle toward hydrogen evolution with hydrogenase, metal complex, and other transition-metal phosphide electrocatalysts.
The HER catalytic performances of the Cu3P and Cu3P@C catalysts with the same loading were evaluated using a three-electrode electrochemical workstation with the electrolyte solution of 0.5 M H2SO4. The commercially available Pt/C catalyst and bare CFP substrate were also examined for comparison. Figure 4(a) shows the LSV polarization curves for Pt/C, bare CFP, Cu3P, and Cu3P@C hybrid. It is found that the Pt/C catalyst exhibits super excellent HER activity with a near-zero overpotential, whereas the bare CFP substrate shows a rather poor activity with negligible current density. The pure Cu3P particles exhibit fairly good activities. As observed, to achieve the cathodic current density of 10 mA/cm2, it needs overpotential of 263 mV for the pure Cu3P catalyst. However, it is notable that when using the Cu3P@C hybrid catalyst, the electrocatalytic activities were dramatically improved, and it only requires overpotential of 203 mV to drive the same current density, which can compare favorably with the performances of other reported Cu3P catalysts (Table S1). It is well known that Tafel slop is another important factor utilized to further get an insight into the electrocatalytic activity toward HER. A smaller Tafel slop indicates a better HER performance which leads to an enhanced HER rate with an increase in overpotential. Figure 4(b) shows the Tafel plots for the Pt/C, Cu3P, and Cu3P@C hybrid, respectively. These linear portions are fitted to the Tafel equation [η versus log (current density) and η is the overpotential]. The Tafel slope of 31 mV/dec for Pt/C catalyst is consistent with the reported value. Reference Tian, Liu, Cheng, Asiri and Sun41 The value of Tafel slop for the pure Cu3P catalyst is calculated to be 91 mV/dec. However, when using the Cu3P@C hybrid catalyst, this value decreases to 83 mV/dec, which suggests that the Cu3P@C hybrid catalyst possesses a superior catalytic activity toward HER over the pure Cu3P catalyst, and base on the early report, Reference Tian, Liu, Cheng, Asiri and Sun41 it can also be obtained that the HER for the both catalysts follows a Volmer-Heyrovsky mechanism [Fig. 4(c)], wherein the electrolytic reaction is limited by the electrochemical desorption step. Besides, by using the extrapolation method to the Tafel plot, the exchange current density of the Cu3P@C hybrid catalyst is calculated to be 0.025 mA/cm2, which is about twice that of the pure Cu3P catalyst (0.013 mA/cm2), highlighting the enhanced H2 evolving efficiency for the Cu3P@C hybrid catalyst.
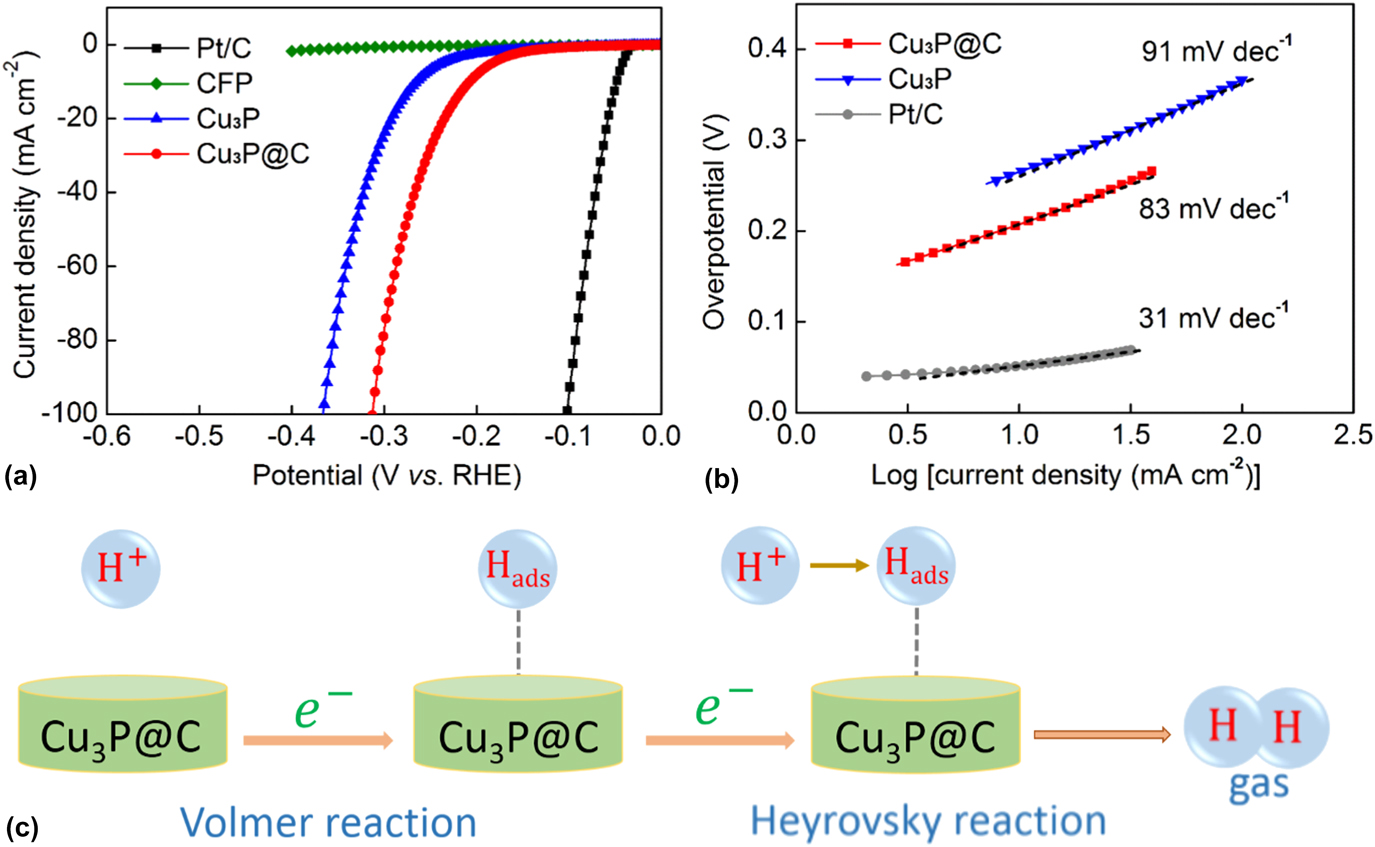
FIG. 4. (a) Polarization curves for the Pt/C, bare CFP, Cu3P, and Cu3P@C hybrid catalyst in 0.5 M H2SO4 with a scan rate of 2 mV/s. (b) Tafel plots for the Pt/C, Cu3P, and Cu3P@C. (c) HER catalytic process undergoes a Volmer–Heyrovsky mechanism for the Cu3P@C hybrid catalyst.
As an important evaluation for an electrocatalyst, the stability of the polarization curves was also characterized by attenuation tests and constant overpotential measurements for the Cu3P@C hybrid catalyst. Figure 5(a) shows the attenuation tests that were performed by 1000-times cyclic sweep for the catalytic materials in 0.5 M H2SO4 between +0.1 and −0.3 V (versus RHE) with a certain scan rate of 100 mV/s. From the CV curves, we can see that the final polarization curve of the Cu3P@C hybrid catalyst nearly overlaps with the first one. To achieve the current density of 10 mA/cm2, the overpotential only increased 5 mV for the Cu3P@C hybrid catalyst after the CV scanning of 1000 times, which shows the excellent stability of the catalyst. The catalytic stability of the Cu3P@C hybrid was further characterized by conducting constant overpotential response tests of the current density at 205 mV (versus RHE). As shown in Fig. 5(b), the responded current density for the Cu3P@C hybrid catalyst has a minor decrease after a long test period of 20 h. Here, the minor decrease may be attributed to the fact that the protons in the electrolyte solution suffer depletion and that the generated gas bubbles adsorbed on the surface of the working electrode hinder the electrolytic reaction. All of the above results confirm the robust catalytic stability toward HER for the prepared Cu3P@C hybrid catalyst.

FIG. 5. (a) Polarization curves for the Cu3P@C hybrid catalyst initial and after 1000 CV scanning between +0.1 and −0.3 V with a scan rate of 100 mV/s. (b) Current density versus time curve for the Cu3P@C hybrid catalyst for 20 h under the static potential of 205 mV.
To examine the electrode kinetics and further reveal the superior HER performance of the Cu3P@C hybrid catalyst, the EIS test was performed. The corresponding Nyquist plots and data fitting to a simplified circuit are shown in Fig. 6(a). As observed, the Niquist plots of both catalysts possess a semicircular shape. As we know, the radius of the semicircle can serve as the reflection of the charge transfer resistance (R ct) or Faraday interfacial resistance for the electrode. The Cu3P@C hybrid catalyst exhibits a R ct for the reduction of protons at the electrolyte/catalyst interface of approximately 26 Ω, which is much smaller than that of the pure Cu3P catalyst (50 Ω), indicating a better electron transfer, and also confirming that the decoration of carbon for the catalyst dramatically enhances the electronic conductivity, which consequently increases the catalytic activities of the catalyst. In addition, the low series resistance (about 2 Ω) indicates the effective electrical integration with small ohmic losses between the catalysts and the conductive substrate. Furthermore, the Nyquist plots for the Cu3P@C catalyst were also recorded at different overpotentials [Fig. 6(b)]. As expected, the R ct sharply decreases with the increasing overpotential, and this is well agreed with the tendency of HER polarization curve, which reveals the potential dependency nature of the Cu3P@C hybrid catalyst.

FIG. 6. (a) Nyquist plots and data fitting to a simplified circuit for the Cu3P and Cu3P@C. (b) Nyquist plots for the Cu3P@C hybrid under different overpotentials.
Overall, such enhanced HER catalytic activity of the Cu3P@C hybrid catalyst can be mainly ascribed to the following aspects: (i) the catalyst consists of Cu3P, known as highly active catalyst toward water splitting for hydrogen evolution; (ii) as shown in Fig. S3, the electrons transfer from the CFP substrate to carbon nanosheets and then transfer to the surface of Cu3P particles to conduct the reduction of protons to produce hydrogen. Therefore the decoration of carbon efficiently increases the electron transport for the Cu3P particles catalyst, which consequently improves the electrocatalytic performance toward hydrogen evolution; (iii) the low I D/I G ratio in the Raman spectrum implies that the carbon in a Cu3P@C hybrid has a high graphitization, comparing with the carbon with low graphitization, it can provide more enhancement for the charge transfer of the catalyst during the electrochemical reaction; (iv) the Cu3P particles are well dispersed in the graphitic carbon matrix leading to the hierarchical structure of the Cu3P@C hybrid catalyst consisting of microparticles with coated sheet-like structure, which may bring in large surface area to expose luxuriant active sites and may also favor H+ absorption on the surface of the catalyst during the electrochemical reaction.
IV. CONCLUSIONS
In this work, we proposed a facial and rapid method to synthesize in situ carbon-decorated Cu3P (Cu3P@C) via a one-pot reaction of the precursors of copper acetylacetonate [Cu(acac)2] and triphenylphosphine (PPh3) at 425 °C for 1 h with the assistance of vacuum encapsulation technique. Compared with the pure Cu3P catalyst, the Cu3P@C hybrid exhibits an enhanced electrocatalytic water-splitting performance for hydrogen evolution with excellent stability. For the Cu3P@C hybrid catalyst, the enhanced HER catalytic activities were also explored. Such results of our study provide an opportunity for the present Cu3P@C-based hybrid as an excellent electrocatalyst for water splitting toward energy conversion and pave a way for designing and fast fabricating in situ carbon-decorated HER catalysts from the organometallic reaction precursors.
Supplementary Material
To view supplementary material for this article, please visit https://doi.org/10.1557/jmr.2017.401.
ACKNOWLEDGMENTS
This work is supported by the National Natural Science Foundation of China (Grant No. 51672031). The authors also acknowledge the support from the sharing fund of large-scale equipment of the Chongqing University (106112017CDJQJ308820).