Introduction
A myriad of factors of dietary, endogenous and bacterial origin occur in the lumen of the small intestine, and therefore have the potential to affect the digestive processes there within. Pancreatic lipase (also referred to as TAG acyl hydrolase(Reference Embleton and Pouton1) and originally named steapsin(Reference Palmer2); EC 3.1.1.3) is one of a range of enzymes secreted by the pancreatic acinar cells that is involved in digesting dietary lipids for subsequent absorption in the small intestine.
cDNA for human pancreatic lipase was first isolated in the 1980s(Reference Lowe, Rosenblum and Strauss3). The primary and tertiary structures of human pancreatic lipase were described soon after(Reference Winkler, D'Arcy and Hunziker4). Three subgroups of human pancreatic lipases have been identified which share about 70 % sequence identity(Reference Van Tilbeurgh, Bezzine and Cambillau5). Pancreatic lipase-related proteins 1 and 2 have similar structure and action to pancreatic lipase, although it is possible they may have different substrate specificity. These enzymes are also produced by the pancreas and secreted into the duodenum, and are characterised in detail elsewhere(Reference Lowe6–Reference Berton, Sebban-Kreuzer and Crenon13). The term ‘pancreatic lipase’ in the present review refers to ‘classical’ or ‘colipase-dependent’ pancreatic lipase from this point on.
Human pancreatic lipase is a 50 kDa peptide chain consisting of two structural domains: the catalytically active, 336-amino acid long N-terminal domain, and the shorter (113 residues) C-terminal domain(Reference Miled, Canaan and Dupuis14), which binds to the cofactor colipase (see below). The lipase family of enzymes acts at the interface of lipid and aqueous mixtures. This interfacial binding is unique in digestive enzymes. The majority of lipases are water soluble and catalyse cleavage of water-insoluble TAG(Reference Beisson, Tiss and Riviere15). Pancreatic lipase differs from other mammalian digestive hydrolase systems in that it is not a single domain enzyme. Its catalytic activity is increased in the presence of the cofactor colipase. As pancreatic lipase acts at the interface of lipid and water, its action is also dependent on the presence of bile acids or other moieties capable of emulsifying lipids(Reference Embleton and Pouton1). As a result of these factors, the classic lock-and-key model does not lend itself well to understanding lipase catalytic behaviour and activity.
Plasma levels of pancreatic lipase are routinely measured in the diagnosis of pancreatic dysfunction(Reference Yadav, Agarwal and Pitchumoni16). In addition, pancreatic enzyme mixtures are often given therapeutically to patients with pancreatic exocrine deficiency, including those with chronic pancreatitis and cystic fibrosis(Reference Ferrone, Raimondo and Scolapio17). In measuring the levels of pancreatic lipase activity, a number of tests that use synthetic substrates have been developed. To reduce interference of other lipolytic factors in plasma, these lipase activity assays are carried out at high (often unphysiological) pH. While such models are clearly useful diagnostic tools, it must be noted that these types of assays do not adequately predict the action of pancreatic lipases under gastrointestinal conditions.
Tetrahydrolipstatin (commonly referred to as orlistat) is a hydrogenated derivative of a naturally occurring compound originally isolated from Streptomyces toxytricini (Reference Hadvary, Sidler and Meister18). It was developed as a pharmacological agent to inhibit dietary lipid absorption during the 1980s and was approved for drug use in 1998(Reference Padwal and Majumdar19). Orlistat inhibits a range of human lipases (including gastric and pancreatic) through covalent binding to the active site serine residue that is essential for enzymic action(Reference Hadvary, Sidler and Meister18).
Previous clinical data suggest that orlistat reduces fat absorption by about 30 % in human subjects. Double-blind, placebo-controlled evidence also demonstrates the efficacy of orlistat in reducing body weight in obese individuals(Reference Sjostrom, Rissanen and Andersen20–Reference Finer, James and Kopelman22), as well as further benefitting other anthropometric and plasma biomarkers of CVD risk in this population group(Reference Davidson, Hauptman and DiGirolamo23, Reference Rossner, Sjostrom and Noack24). The clinical efficacy of orlistat is tempered by common gastrointestinal side effects, including steatorrhoea and faecal incontinence that result from excess dietary lipids entering the colon. While poor compliance for orlistat usage has been reported as a result of these unwanted side effects(Reference Elfhag, Finer and Rossner25), inclusion of extra viscous, non-fermentable fibre in the diet appears to greatly reduce their occurrence(Reference Cavaliere, Floriano and Medeiros-Neto26).
The above evidence for orlistat would suggest that inhibition of pancreatic lipase is an effective strategy for benefitting weight management (and therefore obesity therapy). Through the study of the physiological and biochemical processes involved in intestinal lipid hydrolysis, it may be possible to develop novel pharmaceutical and/or food products that effectively inhibit pancreatic lipase but do not cause unwanted gastrointestinal side effects.
The rest of the present review will summarise evidence on how pancreatic lipase functions, including details on how physiological factors affect its activity and secretion.
Pancreatic lipase
Secretion
Pancreatic acinar cells are highly polarised, with a small apical membrane domain, and a much larger basolateral domain(Reference Wäsle and Edwardson27). They synthesise, store and secrete a variety of enzymes into the duodenal lumen on demand. These enzymes, including pancreatic lipases, are stored in zymogen granules within the apical pole of the cells. A wide range of secretagogues, including acetylcholine and cholecystokinin (CCK), stimulate basolateral membrane receptors in the acinar cells. This generates an intracellular response driven by Ca++, diacylglycerol and cAMP(Reference Williams28). These secondary messengers stimulate fusion of the zymogen granules with the apical membrane, leading to digestive enzyme secretion from the cells. These processes are discussed in greater detail elsewhere(Reference Wäsle and Edwardson27, Reference Thevenod29). While the intracellular pathways involved in pancreatic enzyme release (including protein sorting and zymogen granule binding) are not fully understood(Reference Wäsle and Edwardson27, Reference Williams28), a number of pathways have been suggested for control of the luminal factors relevant to lipolytic activity.
While it is currently unsure whether the release of separate pancreatic enzymes is independent of each other or not(Reference Maouyo and Morisset30), CCK appears to be the main hormonal drive for increased zymogen secretion and, therefore, lipase secretion. CCK is released from I cells and enteric nerves in the duodenal and jejunal mucosa in response to the presence of fats and amino acids in the intestinal lumen(Reference Williams28). Released CCK is believed to affect intestinal stimulation through contact with CCKA receptors found within the pancreas and the intestinal crypt epithelia(Reference Crawley31, Reference Wank32).
Further stimulation for release of pancreatic enzymes comes from parasympathetic acetylcholine release from pre- and postganglionic neurones. This acetylcholine release stimulates muscarinic M3 receptors, which in turn triggers the intracellular cascades driving zymogen granule exocytosis. Cholinergic stimuli will occur within cephalic, gastric and intestinal phases of digestion(Reference Power and Schulkin33).
Pancreatic lipase appears to lose catalytic activity below pH 5. This observation would suggest a potential exacerbation of low lipase activity in disorders causing pancreatic exocrine insufficiency, as not only will lower amounts of lipase be secreted into the duodenal lumen, but it may also be catalytically inactive due to low intestinal pH, caused by a lack of pancreatic bicarbonate secretion.
Previous observations in fasting (healthy) human subjects have noted that peak levels of pancreatic enzyme secretion (including pancreatic lipase) appear to occur at the commencement of phase III of the duodenal migrating motor complex (characterised by regular, high-amplitude phasic contractions). Additional peak levels of secretion also appear to occur at non-specific time points within the interdigestive (fasting) stages of motility(Reference Keller, Groger and Cherian34, Reference Domínguez-Muñoz, Bregulla and Nelson35).
During the fed state, intestinal motor responses to the presence of nutrients in the duodenum appear to occur over the same period of time as pancreatic secretory responses(Reference Keller, Runzi and Goebell36). This is to be expected, as both responses are elicited by similar hormonal drives.
Secretin which is released from duodenal S cells in response to the presence of luminal contents and parasympathetic stimulation may also work in synergy with CCK in up-regulating the release of pancreatic enzymes(Reference Chey, Lee and Chang37). Release of pancreatic polypeptide and somatostatin from the pancreas results in local inhibition of acinar cell exocytosis(Reference Konturek, Konturek and Domschke38). Cholinergic stimulation of pancreatic enzyme exocytosis is also inhibited by elevated circulating concentrations of pancreatic polypeptide(Reference Adrian, Besterman and Mallinson39), glucagon-like peptide-1(Reference Wettergren, Schjoldager and Mortensen40, Reference Wettergren, Schjoldager and Mortensen41) and peptide YY (released from the ileum) and ghrelin and leptin(Reference Konturek, Zabielski and Konturek42, Reference Symersky, Biemond and Frolich43).
Catalytic action
The pancreatic lipase complex catalyses hydrolysis of the ester bonds that attach fatty acids to the glycerol backbone in di- and triacylglycerols. Glycerol contains an alcohol –OH group at each of its three carbons to which fatty acids can be linked by ester bonds(Reference Perona, Ruiz-Guttierez and Nollet44). Pancreatic lipase has no activity towards the central sn-2 ester bonds, but is specific to the cleavage of the outer sn-1 and sn-3 esters. However, sn-2 ester bonds slowly undergo a non-enzymic isomerisation to 1-monoacylglycerols under the alkaline conditions of the small intestine, subsequently making them available for hydrolysis as well, potentially allowing full TAG hydrolysis(Reference Embleton and Pouton1).
Pancreatic lipase retains the same serine 152–histidine 263–aspartate 176 triad that is conserved within other members of the lipase family(Reference Winkler, D'Arcy and Hunziker4, Reference Colin, Deprez-Beauclair and Allouche45). Serine–histidine–aspartate triads also drive catalytic activity in serine proteases(Reference Miled, Canaan and Dupuis14). Four main steps, involving changes in conformation or charge, occur to the pancreatic lipase molecule(Reference Miled, Canaan and Dupuis14):
(1) Initial closed conformation: a loop structure within the N-terminal (residues 237–261), often referred to as the ‘lid’ domain, initially covers the active site. Two other domains maintain the lid in this closed confirmation by van der Waal's forces; β5 (75–84) and β9 (203–223).
(2) Transition to open confirmation: the β5 domain moves away from the lid domain, causing the lid domain to uncover the active site (the open conformation).
(3) Formation of an oxyanion hole: the movement of the β5 domain also creates an electrophilic region around the triad serine residue. This oxyanion hole helps stabilise the intermediate catalytic product formed during the reaction. Aromatic side chains from residues tyrosine 114, phenylalanine 215 and phenylalanine 77 endow the oxyanion hole with hydrophobicity, as does the presence of proline 180, isoleucine 209 and leucine 202.
(4) Binding to substrate and catalysis: two acyl-binding sites in the β9 domain allow the carbonyl carbon of the primary ester bond access to the triad serine 152 residue. The sn-1 (or because of molecular symmetry, the sn-3) acyl chain is held within the oxyanion hole. The sn-2 chain lies within a second hydrophobic groove formed by side chains of lid domain residues (251 to 259) and by isoleucine 78 in the β5 domain(Reference Van Tilbeurgh, Egloff and Martinez46). This results in hydrolysis of the acylglycerol substrate. Two residues (leucine 213 and phenylalanine 215) on the β9 domain contact with alkyl chains are implicated in increasing hydrolysis product stability(Reference Miled, Canaan and Dupuis14).
Interestingly, pancreatic lipase still shows appreciable in vitro activity to hydrolyse dietary TAG in the absence of colipase, but cannot function without the presence of bile salts, as shown in Fig. 1.
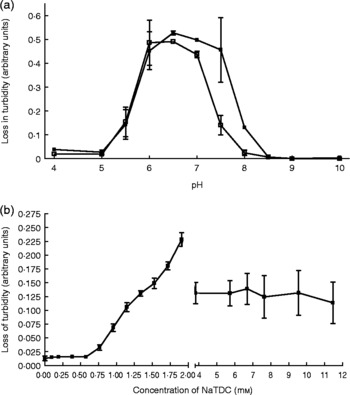
Fig. 1 Effect of colipase presence and bile salt concentration on porcine pancreatic lipase activity. (a) Lipase activity over a pH range in the presence (■; 23·8 μg/ml) and absence (□) of colipase. (b) Lipase activity over a range of bile salt (sodium taurodeoxycholate; NaTDC) concentrations at pH 7. Values are means, with standard errors represented by vertical bars. Olive oil micelles were used as a substrate using procedures modified from Vogel & Zieve(Reference Vogel and Zieve97).
Effect of pH on pancreatic lipase activity
As they must maintain activity under a wide range of physiological conditions, gastrointestinal enzymes show a much greater resistance to irreversible denaturation. In particular, pancreatic lipase has to be stable under a wide range of pH within the small intestine. The enzyme is secreted as part of the pancreatic juice at about pH 8. The approximate pH within the small intestine is suggested to be from about 6·5 in the duodenum to over 7 in the distal ileum. However, previous assessments agree that there is a generally higher pH more distally in the small intestine, and a much wider pH range occurs in both the healthy and diseased state (see Table 1).
Table 1 Reported pH range in the human small intestine

* Range includes highest and lowest mean duodenal pH values for participants with varying duodenal chymotrypsin activity.
† Range includes highest and lowest mean segmental values given.
‡ Only mean proximal and distal values cited.
§ Range includes highest and lowest mean pH values over a 240 min time-course in duodenum and jejunum.
Control of small-intestinal pH
Control of acid output of the stomach (i.e. pH of the digesta entering the small intestine) is reviewed in detail elsewhere(Reference Dockray, Varro and Dimaline47, Reference Hersey and Sachs48). However, as pancreatic lipase activity, and that of other hydrolytic enzymes in the small intestine, favours a more neutral pH, processes must be in place to quickly and effectively raise intestinal pH. The pH of the small-intestinal lumen can be raised by secretion of bicarbonate ions at three separate sites: pancreatic and hepatic ducts and small-intestinal epithelial cells (particularly those located in intestinal crypts). The model of bicarbonate secretion is analogous in these three sites at a cellular level (see Fig. 2), although it must be noted that the small-intestinal epithelia also allows paracellular migration of bicarbonate, due to the intercellular junctions being considerably leakier(Reference Allen, Flemstrom and Garner49) than hepatic and pancreatic duct junctions. Stimulated output of bicarbonate from the luminal epithelium has been suggested to vary along the length of the small intestine, with the highest in outputs in the proximal duodenum equating to approximately 200 μmol/cm per h(Reference Isenberg, Hogan and Koss50). This suggests (assuming small-intestinal length of 5 m) an intestinal output of bicarbonate of up to 100 mmol/h. Secretin stimulation of healthy human participants did not appear to affect hepatobiliary bicarbonate concentration (30 mmol/l pre-stimulation v. 35 mmol/l post-stimulation), but secretion volume rose from 20 ml/h to nearly 80 ml/h(Reference Nyberg51), suggesting a total stimulated bicarbonate output of < 3 mmol/h. Similar studies on pancreatic juice suggest a stimulated volume output of 200 ml/h(Reference Ochi, Harada and Mizushima52) with bicarbonate output reaching 90 mmol/l(Reference Stevens, Conwell and Zuccaro53) (total stimulated output of < 20 mmol/h).

Fig. 2 Putative cellular mechanisms involved with bicarbonate secretion from pancreatic duct, hepatic duct and small-intestinal epithelial cells. Intracellular accumulation of bicarbonate occurs through conversion of CO2 (which passively diffuses into the cell from the blood) by carbonic anhydrase and the action of the basolateral Na–HCO3 co-transporter (NBC). In the unstimulated cell, bicarbonate is removed from the cell by anion exchangers (AE) at the apical and basolateral membranes. Within the stimulated cell, the basolateral AE action is halted. The conductance of the apical chloride leak channel (CFTR) is raised, which results in higher localised chloride concentrations apically, thus driving increased bicarbonate release through the apical AE. During the latter stages of stimulation of bicarbonate release (i.e. when the apical and luminal concentration of bicarbonate is high), it is believed that the apical AE becomes inhibited. Bicarbonate efflux then occurs through the CFTR. Passive diffusion of bicarbonate from the blood to the lumen (left of figure) can only occur in the leaky epithelia of the intestine. Adapted from details in Allen & Flemström(Reference Allen and Flemström54), Kanno et al. (Reference Kanno, LeSage and Glaser98) and Steward et al. (Reference Steward, Ishiguro and Case99). Other membrane transporters indirectly involved in driving these processes, such as the basolateral Na+:K+:2Cl− ATPase and K leak channels, are not included for clarity. NHE, Na+–H+ exchanger.
Secretin is the principal hormonal stimulant driving bicarbonate and fluid secretion into the small intestine(Reference Konturek, Zabielski and Konturek42, Reference Allen and Flemström54, Reference Saetre, Andersen and Houe55). Secretin acts through attachment to G-protein-coupled receptors that also show affinity for vasoactive intestinal peptide(Reference Ulrich, Holtmann and Miller56). Unlike pancreatic enzyme secretions, pancreatic release of bicarbonate appears to be unaffected by pancreatic polypeptide(Reference Adrian, Besterman and Mallinson39).
Bile acids
Bile acids are secreted by the liver, and stored in the gallbladder in man. From here, they are ejected into the duodenum via the biliary duct by contraction of the surrounding smooth muscle. Bile acids are synthesised from cholesterol within hepatocytes. About 95 % of bile acids within secreted bile are actually from the recirculating pool of bile that occurs from liver to duodenum to distal ileum/large bowel to liver(Reference Muller and Jansen57). Recycled bile acids are reabsorbed at the hepatocyte serosal (or sinusoidal) membrane by a range of specific Na-dependent and Na-independent transporters.
The majority of bile acids secreted (both recycled and new) in man are conjugated within the hepatocytes to glycine and taurine amino acid residues. This process is catalysed by bile acyl-CoA:amino acid N-acyltransferase, which is localised in peroxisomes and the cytosol(Reference Ferdinandusse and Houten58). Conjugated bile acids are considerably more soluble in the intestinal milieu than unconjugated bile acids(Reference Kullak-Ublick, Stieger and Meier59), thus endowing bile salts with greater solubility to benefit their role in making dietary lipids more available to pancreatic lipase. Bile acids and salts act as biological detergents to emulsify dietary lipids, thereby greatly increasing the surface area of the lipid–aqueous interface for pancreatic lipase action. Critical micellar concentrations of different types of bile acids tend to occur within a low millimolar range, but bile acids occur well in excess of this concentration in the small intestine(Reference Borgstrom and Erlanson60, Reference Tiss, Ransac and Lengsfeld61).
Following conjugation, bile salts are then secreted into hepatic canaliculi – secretory lumens formed between two or more adjacent hepatocytes and their tight junction complexes. Transport of conjugated bile across the canalicular membrane occurs through ATP-driven bile salt export pumps(Reference Kullak-Ublick, Stieger and Meier59). The canaliculi empty into larger bile ductules that eventually drain into the common bile duct(Reference Coleman62). In man, and many other mammals, the bile drains into the gallbladder for subsequent release. The rat does not have a gallbladder, and so is considered a poor model of human bile circulation, and hence a poor model of fatty acid and cholesterol metabolism.
CCK appears to be the major effector of bile acid secretion, acting to mediate bile release through sphincter of Oddi relaxation and contraction of the smooth muscle surrounding the gallbladder(Reference Schmidt, Creutzfeldt and Schleser63). Other stimuli for gallbladder contraction/sphincter of Oddi relaxation appear to be motilin and vagal efferent impulses, whereas pancreatic polypeptide and somatostatin release acts to inhibit gallbladder output(Reference Niebergall-Roth, Teyssen and Singer64).
Previous reports suggests that bile acid action results in an emulsion of stable lipid particles of less than 0·5 μm in diameter(Reference Embleton and Pouton1). In vitro, higher ratios of unconjugated bile acids appear to inhibit lipase activity(Reference Knarreborg, Jensen and Engberg65), while conjugated bile acids stimulate lipolysis below critical micellar concentrations, but appear to be strongly inhibitory at higher concentrations(Reference Borgstrom and Erlanson60).
A number of reports have suggested that bile acids actually inhibit lipase activity as a result of the observed effect of reducing interfacial adhesion (for example, Gargouri et al. (Reference Gargouri, Julien and Bois66); Patton & Carey(Reference Patton and Carey67)). In the physiological situation, however, the detergent action of bile will greatly increase the surface area within the small-intestinal lumen, thereby increasing total accessibility(Reference Van Tilbeurgh, Bezzine and Cambillau5).
Colipase
Human procolipase is a ninety-five-amino acid protein secreted by the pancreatic acinar cells. It is rapidly converted to colipase by tryptic and proteolytic action(Reference Kerfelec, Allouche and Colin68), causing cleavage at the Arg 5–Gly 6 bond(Reference Cordle and Lowe69). Within this conversion, a five-amino acid fragment is lost. This pentapeptide, also referred to as enterostatin(Reference Okada, York and Bray70), has been suggested to be important in appetite control in animal studies(Reference D'Agostino, Cordle and Kullman71).
Due to shared pathways of secretion, a similar range of neurohumoral mediators is likely to affect up- and down-regulation of procolipase release as that of pancreatic lipase (see above).
Colipase is an 11 000 Da protein that is catalytically inactive on its own, and binds to pancreatic lipases in a 1:1 ratio(Reference Dahim and Brockman72). Structurally, colipase is a small amphipathic protein, stabilised against acid denaturation by five disulfide bridges(Reference Sugar, Mizuno and Momsen73). As it can interact with both aqueous and non-aqueous compounds, it is believed to be important in helping ‘anchor’ pancreatic lipases to lipid droplets and micelles. The presence of NEFA in lipid micelles has been suggested to favour colipase interfacial binding(Reference Dahim and Brockman72, Reference Borgstrom74).
Murine knock-out studies have shown that procolipase-deficient mice had a 60 % lower postnatal survival rate (presumably due to a decrease in the available energy during weaning) and also had reduced body weight (by 30 %) compared with wild types during and after weaning. In addition, when knock-out mice were fed a high-fat diet, they exhibited fat malabsorption through steatorrhoea(Reference D'Agostino, Cordle and Kullman71).
Crystallographic analysis suggests that colipase binds to the non-catalytic, C-terminal domain of pancreatic lipase(Reference Van Tilbeurgh, Bezzine and Cambillau5). This interaction between colipase and the C-terminal is through the amino acids in two colipase hairpin loops. The weak association between the two proteins is increased in the presence of a lipid interface(Reference Van Tilbeurgh, Bezzine and Cambillau5). The binding of colipase to pancreatic lipases is also believed to play a role in stabilising the open-lid conformation(Reference Dahim and Brockman72). Mutations at the Glu 15 residue of the colipase protein, which occurs within the area that binds to the lipase lid, greatly reduced the potential of colipase to maintain the open-lid confirmation(Reference Van Tilbeurgh, Bezzine and Cambillau5).
The main area of the colipase that interacts with micelles is believed to be the 70–85 loop(Reference Van Tilbeurgh, Bezzine and Cambillau5). Further residues have been suggested to be important in this interaction through a range of studies. Computational modelling of colipase binding with lipid droplets and bile salt micelles hypothesises that hydrophobic finger areas of the colipase molecule are the interfacial binding site(Reference Kerfelec, Allouche and Colin68). Interfacial interactions of colipase have been shown by in vitro study to be dependent on the lipid substrate involved, as well as the ionic strength and pH of the aqueous phase(Reference Kerfelec, Allouche and Colin68). Previous studies have demonstrated that colipase–lipase binding occurs in such a way that the hydrophobic regions of the colipase molecule are orientated towards the lipid–aqueous interface(Reference Van Tilbeurgh, Bezzine and Cambillau5, Reference Cordle and Lowe75). Site-directed mutagenesis towards this area revealed that Tyr 55 and 59 residues appeared to be important in the ability of colipase to act as a cofactor(Reference Cordle and Lowe75) in pancreatic lipase produced from human cDNA. Further suggestion that this hydrophobic region of colipase was integral in interface binding came from NMR analysis of conserved areas of porcine and equine colipases. Within these studies, two aromatic residues (Tyr 55 and either Trp or Phe residues at the 52 position) were also associated with interaction with bile salt micelles(Reference Dominguez, Sebban-Kreuzer and Bornet76).
Calcium ions
Unlike other factors involved in intestinal lipolysis, Ca++ concentration within the small-intestinal lumen is mainly as a result of dietary intake. Ca is believed to be involved in the catalytic activity of the pancreatic lipase–coenzyme complex. Previous in vitro lipolysis studies have demonstrated an increase in purified human pancreatic lipase activity of about 8 % in the presence of 0·5 mm-Ca compared with a Ca-free solution. No further increase in activity was seen with higher concentrations of Ca up to 4 mm(Reference Lessinger, Férard and Mignot77). Early work in this area using radioisotope studies suggested that Ca has no binding affinity for either colipase or lipase. However, low concentrations (plateau of maximal activity at 40 μm-Ca++) still appeared to increase lipolysis Vmax (maximum velocity of reaction) and decrease the apparent K m for the olive oil emulsion substrate(Reference Kimura, Futami and Tarui78). It has been postulated that the presence of Ca++ reduces the lag phase of pancreatic lipase-mediated lipolysis(Reference Alvarez and Stella79). The presence of Ca ions was shown to reduce the surface charge of micelle droplets, but this did not correlate with lag phase duration(Reference Wickham, Garrood and Leney80). From the above evidence, it appears that Ca++ is not essential for intestinal lipolysis. It is unlikely that luminal concentrations (largely governed by dietary intake) will fall below micromolar levels, and even if they do, this only has minor consequence to pancreatic lipase activity.
Luminal control of intestinal lipolytic activity
Enteroendocrine system
While control of intestinal lipolysis is mediated by endocrine, neural and paracrine factors basolaterally, the initial stimulus that affects the release of these factors comes from the composition of the digesta within the gut lumen. Conditions that drive the release of these agonists and antagonists of pancreatic exocrine secretion and other processes involved in intestinal lipolysis are mediated through sampling (chemo-sensing) of the luminal contents by enteroendocrine cells(Reference Dockray81, Reference Sternini, Anselmi and Rozengurt82). Mechanical and luminal stimuli cause release of enteroendocrine intracellular Ca++ stores, resulting in the release of humoral mediators of digestive control. Within the small intestine, specific luminal factors are known to drive lipolytic activity.
Factors such as the presence of an acidic bolus and digested proteins and fats have been demonstrated to cause the release of CCK or secretin from the small-intestinal mucosa in human studies(Reference Liddle83). In particular, strong CCK releases have been noted in response to the presence of luminal fatty acid with a chain length of > eleven carbons(Reference McLaughlin, Luca and Jones84) or the amino acids tryptophan and phenylalanine(Reference Liddle83). Secretin release appears to be mainly driven by intestinal luminal acidity(Reference Holst, Lauritzen and Jensen85, Reference Nishiwaki, Satake and Kitamura86), particularly below pH 4·5.
Luminal down-regulation of lipolytic secretions comes from intestinal tryptic and proteolytic activity(Reference Owyang, Louie and Tatum87, Reference Ihse, Lilja and Lundquist88). This feedback regulation was shown to only affect CCK-mediated pathways, not cholinergic pathways, in human studies(Reference Owyang, May and Louie89). Tryptic activity has also been shown to reduce pancreatic lipase activity in vitro, due to proteolysis of the active enzyme(Reference Layer, Jansen and Cherian90).
Dietary inhibitors of lipolytic activity
More recently, a number of other naturally derived compounds have been noted to have an impact on pancreatic and gastric lipase activity in vitro or in animal studies, including those from seaweed(Reference Ben Rebah, Smaoui and Frikha91, Reference Bitou, Ninomiya and Tsujita92), green tea(Reference Koo and Noh93), berries(Reference McDougall, Kulkarni and Stewart94) and wheat flour(Reference Tani, Ohishi and Watanabe95). A recent review has also highlighted a number of other plant products that inhibit pancreatic lipase action, including saponins and polyphenols(Reference Birari and Bhutani96). It must be noted that such compounds are likely to have similar unwanted gastrointestinal side effects to orlistat unless consumed at lower amounts or in the presence of bulking agents(Reference Cavaliere, Floriano and Medeiros-Neto26).
Summary
Intestinal lipolysis is perhaps the most complex endogenous enzyme-driven process that occurs within the human gut. Three major factors (pancreatic lipase, colipase and bile acids) are released in response to various luminal conditions that drive the rate of dietary lipid digestion. The formation of lipid micelles and their potential to bind to lipase–colipase complexes are important rate-determining steps in this catalytic process.
The present review highlights the cascade of luminal, local and neurohumoral factors associated with the rate of lipid hydrolysis in the small intestine. Novel treatments that aim to target lipolytic pathways to treat obesity should aim to do so in a way that does not produce unwanted gastrointestinal side effects in order to increase patient compliance.
Acknowledgements
I. A. B.'s salary was paid through a Biotechnology and Biological Sciences Research Council (BBSRC) Diet and Health Research Industry Club (DRINC) project grant (no. BB/G00563X/1 held by J. P. P. and C. J. S.), while M. D. W. was sponsored by a BBSRC Collaborative Awards in Science and Engineering (CASE) studentship (no. BBS/S/M/2006/13 035 held by J. P. P.) during the preparation of this paper.
All authors contributed equally to the preparation of this paper.
The authors report no conflicts of interest arising from the publication of this review.