Introduction
The prevalence of obesity has doubled in the last 50 years, with a third of the global population being considered either overweight or obese. Reference Driscoll and Gregory1 Obesity is a high priority health concern as it is associated with reduced glycemic control and dyslipidemia including altered distribution of lipoprotein particle number and size. Further, individuals with obesity are more likely to develop type 2 diabetes, cardiovascular disease, and some types of cancers. Reference Waddell and Orfila2
The pathogenesis of obesity is complex and multi-factorial, involving genetic predisposition and lifestyle factors including limited exercise and poor nutrition. Reference Chooi, Ding and Magkos3 In addition, the more recent rise in pediatric obesity suggests that maternal health status during pregnancy may influence fetal metabolic exposures in utero or in early postnatal life to promote longer-term obesity and metabolic disease risk. Reference Di Gesu, Matz and Buffington4 Thus, the high incidence of maternal overweight and obesity in women of childbearing age is of particular concern Reference Ogunwole, Zera and Stanford5 as this environment has been shown to program metabolic maladaptation in offspring, increasing the risk of childhood obesity and metabolic dysfunction. Reference Catalano and Shankar6,Reference Howell and Powell7
There is currently an unmet and urgent need to identify maternal interventions for obesity management that improves maternal pregnancy health and reduces the transgenerational impact of maternal obesity in offspring. In non-pregnancy populations, current recommended treatment approaches for obesity are primarily centered around lifestyle changes where patients are encouraged to improve their diet (i.e. focus on nutrient quality, lower-energy foods, etc.) and exercise regularly, though medication and surgery may be recommended in cases where behavioral modification is not effective. Reference Siega-Riz, Bodnar, Stotland and Stang8 However, obesity management in women may require special considerations, particularly during pregnancy. Reference Johnson, Anekwe, Washington, Chhabria, Tu and Stanford9 As weight loss and the use of anti-obesity pharmacotherapies are not recommended during pregnancy, the importance of achieving a healthy body weight and improving obesity-associated metabolic issues prior to pregnancy are encouraged to support both maternal and future offspring health. Reference Siega-Riz, Bodnar, Stotland and Stang8,Reference Johnson, Anekwe, Washington, Chhabria, Tu and Stanford9 However, a recent meta-analysis reported that maternal diet and lifestyle interventions among overweight and obese pregnant women had little effect on the risk of early childhood obesity, Reference Louise, Poprzeczny and Deussen10 re-emphasizing the importance of lifestyle interventions that are initiated before pregnancy and continue throughout the pregnancy and early postpartum periods.
One potentially feasible dietary approach to help achieve a healthy body weight is increased consumption of dietary fiber. As plant-based carbohydrates that are not digested or absorbed in the small intestine, dietary fibers may assist in body weight regulation by reducing dietary energy density, increasing satiety, and promoting a healthy gut microbiome. Reference Benítez-Páez, Gómez Del Pulgar and Kjølbæk11,Reference Haenen, Zhang and Souza da Silva12 In non-pregnant adults, high fiber dietary intake has been shown to improve weight loss often without the need for calorie-restriction, Reference Lambert, Parnell, Tunnicliffe, Han, Sturzenegger and Reimer13,Reference Kim, de Souza and Choo14 decrease waist circumference, Reference Venn, Perry and Green15,Reference Mollard, Luhovyy, Panahi, Nunez, Hanley and Anderson16 and improve a range of obesity-associated metabolic perturbations including dyslipidemia Reference Ghavami, Ziaei and Talebi17 and reduced glycemic control. Reference Xu, Fu and Qiao18 Despite these findings, there has been limited research to assess the health benefits of maternal fiber consumption in obese pregnancies on the metabolic health of offspring. Therefore, using a diet-induced obese Sprague-Dawley rat model, we aimed to examine the protective effects of maternal yellow-pea fiber supplementation on offspring metabolic function as adults.
Materials and methods
Animal’s diets, and design
The rats used in this experiment were cared for in accordance with the guidelines established by the Institutional Animal Care and Use Committee. All procedures were reviewed and approved by the Animal Care Committee at the University at Buffalo. Sixty newly-weaned postnatal day (PND) 21 female Sprague-Dawley (Charles River, obese prone, Crl:OP-CD) rats were housed in the Laboratory Animal Facility at the University at Buffalo under controlled environmental conditions (12 h light:12 h dark, 20°C, and 50% humidity). All animals had free access to food and water throughout the experiment. During the initial 6-weeks of prepregnancy, rats were randomized to a low-calorie control diet (CON; n = 10; total energy 3.8 kcal/g; % energy from fat, 10; protein, 20; and carbohydrate, 70) (Research Diets, D12450K) or a high caloric obesity-inducing diet (HC; n = 50; total energy 4.8 kcal/g; % energy from fat, 44; protein, 20; and carbohydrate, 35) (Research Diets, D12451). Following this 6-week period, HC animals demonstrating a body weight ≥20% over the CON animals were considered “obese” and were then randomized to either remain on the HC diet (n = 20) or provided the HC diet supplemented with yellow-pea fiber (HC + FBR, n = 15) (25%, Roquette® Pea Fiber I 50 m; total energy 3.8 kcal/g; % energy from fat, 50; protein, 22; and carbohydrate, 30) for an additional 4-weeks prior to mating (Table 1). Macronutrient and fiber content of the pea fiber supplement was assessed by third party analyses (Table 1) (Anresco Laboratories, San Francisco, Ca, USA). At end of the prepregnancy period (a total of 10 weeks) the rats were bred with CON-fed male breeders to establish a timed pregnancy, Reference Heyne, Plisch, Melberg, Sandgren, Peter and Lipinski19 confirmed by the presence of a vaginal plug. Maternal body weights and food intake were collected weekly throughout gestation and lactation. Following delivery, litter size and weights were recorded, and the litters were adjusted to 8 pups per dam (4 males and 4 females) within 24 hours of birth. At weaning on PND 21, 6 offspring from each group (3 males and 3 females) were randomly selected for metabolic phenotyping in the non-fasted state. Following weaning, one male and one female offspring from each litter were weaned onto the CON diet until PND 120. Food intake (ad libitum) and body weights were monitored weekly throughout the post-weaning period. On PND 120, adult offspring were anesthetized for terminal non-fasting blood collection by cardiac puncture. The rats used in this experiment were cared for in accordance with the guidelines established by the Institutional Animal Care and Use Committee. All procedures were reviewed and approved by the Animal Care Committee at the University at Buffalo. Livers were quickly excised, weighed, flash frozen in liquid nitrogen, and stored at -80˚C for further processing and analyses.
Table 1. Experimental diet formulation (% composition)

1 CON, low-calorie control diet based on D12450 formulation (Research Diets); HC, high calorie obese-inducing diet based on D12451 (Research Diets); HC + FBR, HC diet supplemented with yellow-pea fiber (25%, Roquette® Pea Fiber I 50 m; total energy 3.8 kcal/g; % energy from fat, 50; protein, 22; and carbohydrate, 30; insoluble fiber content, 43.99%; soluble fiber content, 4.45%); HC + FBR diet was formulated to be matched for energy and macronutrient contribution based on proximate nutrient analyses of pea fiber.
Blood biochemistry
Serum analyses included glucose by colorimetric detection (Invitrogen, EIAGLUC), insulin by ELISA (Millipore, EZRMI-13K), cholesterol (TC, LDL/VLDL-C, and HDL-C) by enzymatic analysis (BioAssay, EHDL-100), and triglyceride (TG) by enzyme assay (Zenbio, STG-1-NC). Direct assessment of lipoprotein subclass distribution, including particle number (nM) and size (nm), for triglyceride-rich lipoproteins (very low-density lipoprotein /chylomicron), low-density lipoprotein (LDL), and high-density lipoprotein (HDL), was conducted by nuclear magnetic resonance spectroscopy (NMR) using automated signal acquisition followed by computational analysis and proprietary signal processing algorithms (LabCorp Inc., Raleigh, NC). Reference Jeyarajah, Cromwell and Otvos20 Lipoprotein distribution was assessed in male offspring only, given the lack of treatment effects in serum lipids amongst female offspring. Hepatic TG was extracted from frozen tissue and analyzed with a commercial kit (Zenbio, STG-1-NC) according to previously published work. Reference Rideout, Andreani and Pembroke21
Data analyses
All statistical analyses were conducted using SPSS 16 (SPSS Inc., Chicago, IL). Data were checked for normality using the Shapiro-Wilk test. Maternal outcomes were measured with a one-way ANOVA with a least significant difference (LSD) post-hoc test. Litters from each dam were considered as a single observation. Main effects of maternal exposure (CON, HC, and HC + FBR) and sex (male and female from same maternal exposure), and interaction-related effects were analyzed by two-way ANOVA. If a significant main effect or interaction was detected, a one-way ANOVA with an LSD post-hoc test was conducted to assess programing responses. Data are presented as means ± SE. Differences were considered significant at p < 0.05.
Results
Compared with CON, rats consuming the HC diet in prepregnancy demonstrated increased (p < 0.05) body weight and caloric intake (Fig. 1a, b), which persisted throughout the gestation and lactation phases. Initiation of the HC + FBR diet at week 7 did not (p > 0.05) protect against body weight gain or reduce feed intake throughout gestation and lactation compared with the HC group.

Figure 1. Body weight trajectory and food intake in mothers and offspring. [ a ] Body weight (g) in prepregnancy, gestation, and lactation in mothers consuming a control [CON], high calorie [HC], and HC + yellow-pea fiber [HC + FBR] diet; [ b ] Maternal caloric intake (kcal/g) in mothers in prepregnancy, gestation, and lactation; [ c ] Postnatal body weight in male and female offspring from CON, HC, and HC + FBR mothers; [ d ] Average postnatal daily caloric intake (kcal/d) in male and female offspring. Data are means ± SE; n = 8-10 per group; ab groups that do not share a superscript are significantly different (p < 0.05).
Adult male, but not female offspring, from obese mothers (HC) demonstrated increased (p < 0.05) body weight and caloric intake (Fig. 1 c, d) compared with offspring from CON-fed mothers, however, maternal supplementation of HC + FBR throughout prepregnancy, gestation, and lactation did not protect against this response.
Compared to CON, adult male and female offspring from HC-fed mothers showed an increase (p < 0.05) in serum glucose and HOMA-IR (Fig. 2 a, c). Male HC offspring showed an additional increase (p < 0.05) in serum insulin (vs. CON) that was not observed in females (Fig. 2 b). Maternal supplementation of pea fiber improved (p < 0.05) serum glucose, insulin, and HOMA-IR in adult male offspring compared with HC offspring, but no protective effect was observed in females.
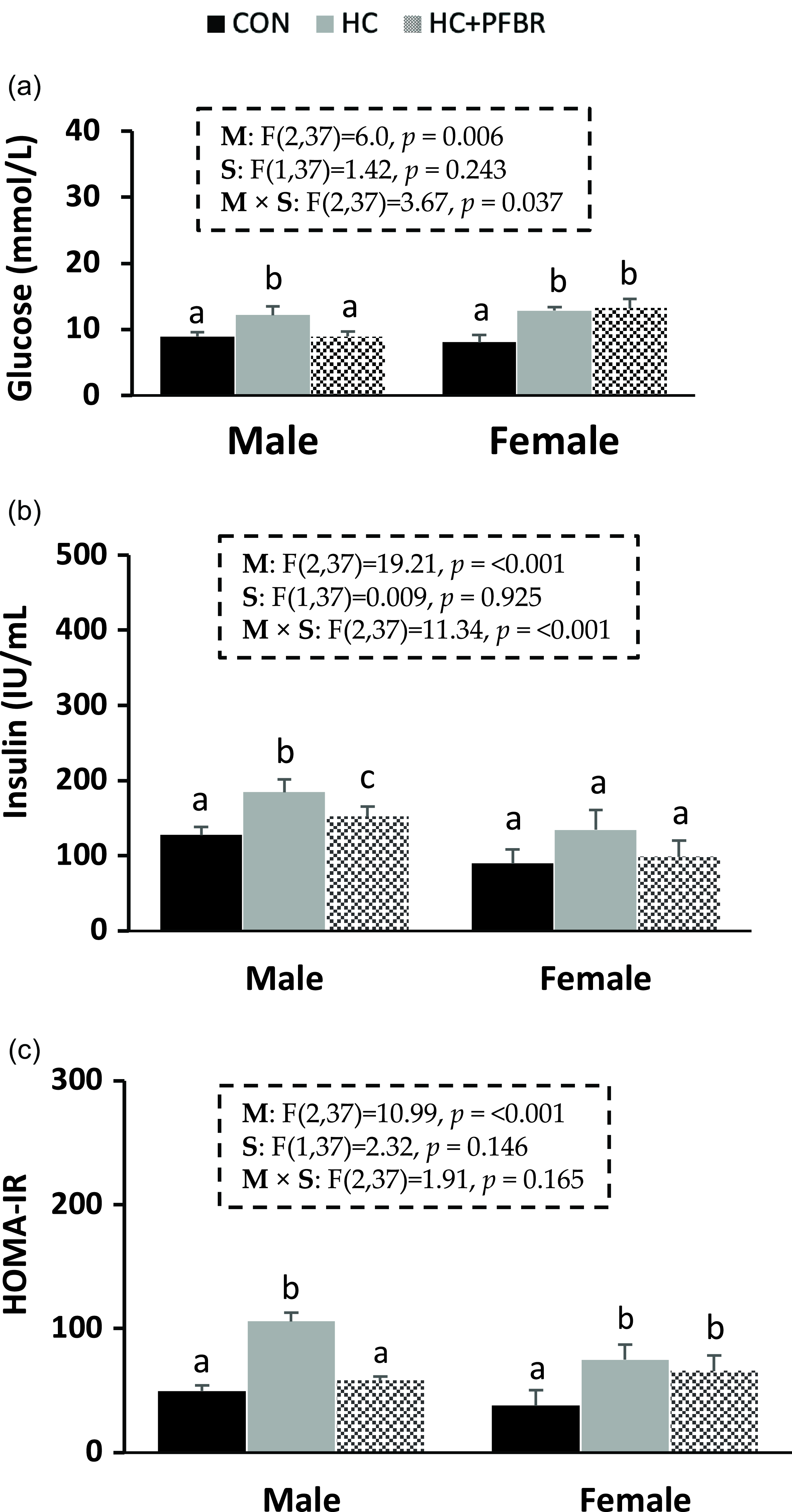
Figure 2. Glycemic control markers in adult male and female offspring from mothers consuming a control [CON], high calorie [HC], and HC + yellow-pea fiber [HC + FBR] diet. [ a ] Serum glucose (mg/dL); [ b ] Serum insulin (IU/ml); [ c ] HOMA-IR. Data are means ± SE; n = 8-10 per group; ab groups that do not share a superscript are significantly different (p < 0.05).
Compared to CON, adult males from HC-fed mothers had higher (p < 0.05) serum total-C and LDL/VLDL-C (Fig. 3a,c), but no difference (p > 0.05) in HDL-C or TG (Fig. 3 b, d). Maternal yellow-pea fiber supplementation reduced (p < 0.05) serum LDL/VLDL-C (vs. HC) and TG (vs. CON) concentrations in adult male offspring (Fig. 3c, d), but did not influence total-C and HDL-C concentrations. No between group differences in serum lipids were observed in females.
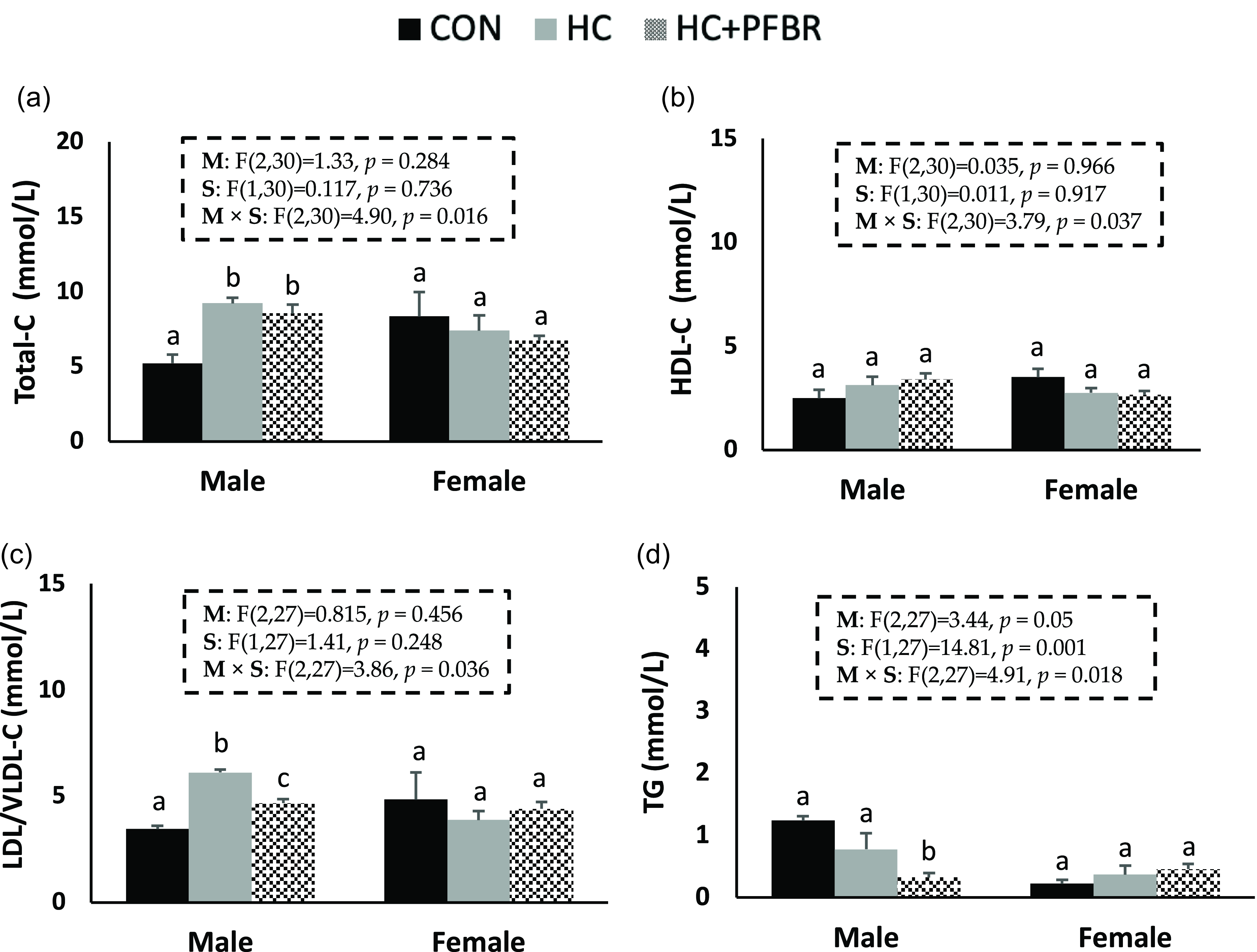
Figure 3. Lipid profile in adult male and female offspring from mothers consuming a control [CON], high calorie [HC], and HC + yellow-pea fiber [HC + FBR] diet including [ a ] Serum total cholesterol (Total-C) (mg/dL); [ b ] Serum HDL-C (mg/dL); [ c ] Serum LDL/VLDL-C (mg/dL); and [ d ] Serum triglycerides (TG) (mg/dL) in male and female offspring. Data are means ± SE; n = 8-10 per group; ab groups that do not share a superscript are significantly different (p < 0.05).
Males from HC mothers demonstrated an altered lipoprotein distribution pattern with increased (p < 0.05) number of TG-rich lipoproteins particles and smaller LDL particles compared with offspring from CON mothers (Table 2). Maternal supplementation of yellow-pea fiber normalized LDL size to CON levels and reduced (p < 0.05) the size of TG-rich lipoproteins lower than both the CON and HC groups.
Table 2. Lipoprotein distribution in adult male offspring

CON, low-calorie control diet; HC, high-calorie obese-inducing diet; HC + FBR, HC high calorie diet with supplemental yellow-pea fiber (25%).
a,b,cTreatment groups that do not share a superscript are significantly different (p < 0.05). Data are means ± SE, n = 8–10 mothers per group.
Compared with CON, maternal obesity programed an increase (p < 0.05) in hepatic TG in adult male offspring and maternal yellow-pea fiber supplementation tended (p = 0.07) to protect against this response, however, it was not significant (Fig. 4). No between group differences in liver TG were observed in female offspring.

Figure 4. Hepatic triglycerides (μmol/g) in adult male and female offspring from mothers consuming a control [CON], high calorie [HC], and HC + yellow-pea fiber [HC + FBR] diet. Data are means ± SE; n = 8-10 per group; ab groups that do not share a superscript are significantly different (p < 0.05).
Discussion
In this study, maternal diet-induced obesity programed hyperphagia, increased body weight, reduced glycemic control, and dyslipidemia, including altered lipoprotein distribution and increased hepatic fat accumulation, in adult male offspring compared with offspring from lean mothers. Although maternal yellow-pea fiber supplementation throughout prepregnancy, pregnancy, and lactation did not influence maternal obesity or offspring body weight status, several metabolic health benefits were evident in adult male, but not female, offspring including improved glycemic control and dyslipidemia.
We observed that adult male offspring from obese mothers had higher body weight and food intake than offspring from CON mothers, confirming that maternal obesity can increase the risk of obesity in offspring, at least in males. This sex-specific response has been observed in some, Reference Tajaddini, Kendig, Prates, Westbrook and Morris22,Reference Kulhanek, Abrahante Llorens, Buckley, Tkac, Rao and Paulsen23 but not all, Reference Kirk, Samuelsson and Argenton24,Reference Samuelsson, Matthews and Argenton25 previous rodent model studies investigating the transgenerational impact of maternal obesity. Previous work in rodent models suggests this hyperphagic/obesogenic response may be mediated, at least partially, by altered expression of central appetite reward systems including preferential enhancement of orexigenic (NPY/AgRP) versus anorexigenic neural peptide signaling in the hypothalamus. Reference Desai, Ferrini, Han, Narwani and Ross26,Reference Desai, Han and Ross27
Our findings do not support a protective effect of maternal dietary fiber supplementation on gestational weight gain Reference Hallam and Reimer28,Reference Mohammed, Qadri, Mir, Kondapalli, Basak and Rajkumar29 or offspring adulthood obesity Reference Mohammed, Qadri, Mir, Kondapalli, Basak and Rajkumar29,Reference Kimura, Miyamoto and Ohue-Kitano30 that has been reported in other previous rodent model studies. Hallam et al. Reference Hallam and Reimer28 reported that maternal consumption of a high prebiotic fiber diet (21.6% w/w mix of oligofructose and inulin) in a Wistar rat model throughout pregnancy and lactation reduced dam weight gain during pregnancy and lowered body fat mass in female, but not male, offspring compared with a high protein diet. A previous human study also supports a negative association between maternal dietary fiber intake and adiposity in female offspring in the first two years of life. Reference Heard-Lipsmeyer, Diaz and Sims31 Unfortunately, as we assessed obesity by weekly body weight measures only, we are not able to evaluate potential changes in body composition between treatment groups. However, a previous study in mice lends support for the possibility that maternal fiber supplementation throughout pregnancy and lactation could influence body weight by selectively influencing organ weight (intestinal and muscle mass) in male offspring (Desbuards et al. 2012). These contradictory sex-specific findings are likely due to many experimental design factors that influence the outcomes of rodent model studies assessing the transgenerational impact of maternal obesity, Reference Preguica, Alves and Nunes32 including the source and amount of dietary fiber. The limited amount of work that has been conducted with maternal dietary fiber intervention during pregnancy in rodents has been conducted with a variety of fiber sources including galacto-oligosaccharides, Reference Desbuards, Gourbeyre, Haure-Mirande, Darmaun, Champ and Bodinier33 fructooligiosacccharides, Reference Mohammed, Qadri, Mir, Kondapalli, Basak and Rajkumar29 inulin, Reference Kimura, Miyamoto and Ohue-Kitano30,Reference Hsu, Lin, Hou and Tain34 and oligofructose and inulin. Reference Hallam and Reimer28 Dietary fibers are known to elicit differential metabolic responses based on their physiochemical properties (i.e., viscosity, fermentability, soluble to insoluble ratio, etc.), Reference Fak, Jakobsdottir and Kulcinskaja35,Reference Weitkunat, Stuhlmann and Postel36 thus conflicting findings between studies may be expected. For instance, the pea fiber supplement used in the present study had a total fiber content of 48%, composed of insoluble (44%) and soluble (4.5%) fiber fractions, likely making it difficult to compare with other studies using different amounts and types of dietary fiber sources.
Similar to a recent mouse model study, Reference Nicholas, Nagao, Kusinski, Fernandez-Twinn, Eliasson and Ozanne37 we observed reduced glycemic control in males and females from HC-fed mothers compared to offspring from lean (CON) mothers, as demonstrated by increased serum glucose, increased serum insulin (males only), and reduced HOMA-IR. Further, in a previous human study, Mingrone et al. reported reduced glucose clearance in female offspring (∼23 years old) from obese vs lean mothers. Reference Mingrone, Manco and Mora38 Reduced insulin sensitivity in offspring from obese pregnancies may be due to structural remodeling of pancreatic islets, including reduced β-cell mass Reference Bringhenti, Ornellas, Martins, Mandarim-de-Lacerda and Aguila39 and increased α-cells, Reference Gregorio, Souza-Mello, Mandarim-de-Lacerda and Aguila40,Reference Bringhenti, Ornellas, Mandarim-de-Lacerda and Aguila41 and decreased expression of genes involved in insulin secretion. Reference Yokomizo, Inoguchi and Sonoda42,Reference Han, Xu, Epstein and Liu43
Dietary pea fiber intake has been reported to improve glycemic control in diet-induced obese male rats, an effect which may be related to changes in the gut microbiome community Reference Eslinger, Eller and Reimer44,Reference Hashemi, Fouhse, Im, Chan and Willing45 as well as increased expression of genes involved in gut epithelial function and short chain fatty acid production. Reference Hashemi, Fouhse, Im, Chan and Willing45 To our knowledge, maternal pea fiber intake has not been previously assessed in pregnancy, however, other types of dietary fibers have shown promise as a maternal intervention to prevent the programing of metabolic dysfunction function in offspring. In a diet-induced Golden Syrian hamster model, Mohammed et al. 2022 reported that maternal dietary supplementation of fructooligiosacccharides from preconception throughout lactation was effective at improving glycemic control in offspring from obese pregnancies. Reference Mohammed, Qadri, Mir, Kondapalli, Basak and Rajkumar29 Similarly, compared with offspring from low fiber-fed mothers, mouse offspring born to mothers consuming a high fiber inulin-supplemented diet during pregnancy reduced blood glucose and conferred resistance to adult-onset obesity by beneficially shifting maternal gut microbiota composition. Reference Kimura, Miyamoto and Ohue-Kitano30
Consistent with other studies in similar obese rat models, Reference Bariani, Correa and Dominguez Rubio46,Reference Ribaroff, Wastnedge, Drake, Sharpe and Chambers47 we observed a range of blood lipid abnormalities in adult male offspring from obese vs. lean mothers including higher serum concentration of total-C and LDL/VLDL-C. In addition to changes in the serum cholesterol profile, maternal obesity altered lipoprotein distribution in male offspring with evidence of a higher concentration of TG-rich lipoproteins and smaller LDL particles. The mechanism(s) underlying these responses are not completely understood but are likely associated with the reduction in insulin sensitivity observed in HC offspring compared with offspring from lean (CON) mothers. Insulin influences both postprandial and fasting lipid metabolism by regulating the activity of peripheral lipases and lipid transfer proteins, lipid uptake and efflux transporters, intracellular lipid synthesis and oxidation pathways, and the production and secretion of lipoproteins. Reference Klop, Elte and Cabezas48,Reference Titchenell, Lazar and Birnbaum49 Consequently, the blood lipid and lipoprotein profile in type 2 diabetes is exemplified by elevated TG and LDL-C concentrations, large TG-rich VLDL particles, and small LDL particles that are prone to oxidation. Reference Singh, Rainwater and Haffner50–Reference Woodman, Watts, Playford, Best and Chan52 Although we are not aware of previous studies that have assessed potential perturbations in lipoprotein distribution in offspring from obese pregnancies, obese mothers have been reported to have an increase in small, dense LDL particles in the third trimester, Reference Meyer, Stewart and Brown53 indicating the potential fetal exposure to an atherogenic lipoprotein profile in an obese gestational environment. The observed lipoprotein profile in HC offspring may signal potential longer-term disease risk complications as LDL particle number has been reported to be more strongly associated with CVD than LDL-C alone. Reference Cromwell, Otvos and Keyes54,Reference Aday, Lawler, Cook, Ridker, Mora and Pradhan55 Further, several reports suggest that offspring from obese pregnancies have increased risk for cardiovascular events, Reference Reynolds, Allan and Raja56,Reference Cooper and Power57 however, the specific contribution of dyslipidemia to CVD incidence in offspring from obese pregnancies is not known.
As a maternal intervention, maternal pea fiber supplementation throughout prepregnancy, gestation, and lactation was protective against dyslipidemia induced by maternal obesity by largely normalizing serum LDL/VLDL-C concentration and LDL particle size and by reducing serum TG and the size of TG-rich lipoproteins (vs. both CON and HC). Outside of pregnancy, dietary fiber supplementation, including yellow-pea fiber, Reference Liu, Xiao and Fang58 has been shown to have numerous beneficial effects on serum lipid profiles in high fat-fed male animals. Reference Han, Zhang, Muheyati, Lv, Aikebaier and Peng59 However, few studies have assessed blood lipids in offspring from obese mothers supplemented with dietary fiber during pregnancy. Mohamed et al. (2022) reported that daily gavage of mung bean fructooligiosacccharides to high fat-fed (60% energy from fat) mothers from preconception to the end of lactation reduced serum triglycerides (6 months of age) and increased serum HDL concentration (at 6 and 12 months of age) in offspring. Reference Mohammed, Qadri, Mir, Kondapalli, Basak and Rajkumar29
With higher hepatic TG concentration in HC vs. CON male offspring, our findings support the association between maternal obesity and nonalcoholic fatty liver disease in offspring reported in previous rodent model Reference Mouralidarane, Soeda and Visconti-Pugmire60,Reference Han, Zhu and Huang61 and human investigations. Reference Hagstrom, Simon, Roelstraete, Stephansson, Soderling and Ludvigsson62,Reference Zeng, Shen and Zou63 A similar sex-specific predisposition to nonalcoholic fatty liver disease was reported in F1 male offspring from obese mothers in a Wistar rat model. Reference Lomas-Soria, Reyes-Castro and Rodriguez-Gonzalez64 Previous work suggests that this connection may be mediated through a variety of mechanisms including reduction in the autophagy-mediated degradation of cytoplasmic lipid droplets, Reference Han, Zhu and Huang61 liver transcriptome changes in genes that regulate liver lipid metabolism, Reference Lomas-Soria, Reyes-Castro and Rodriguez-Gonzalez64 innate immune dysfunction, Reference Mouralidarane, Soeda and Visconti-Pugmire60 and persistent shifts in hepatic DNA methylation and cecal microbiome populations. Reference Wankhade, Zhong and Kang65,Reference Paul, Collins and Nicolucci66 Maternal yellow-pea fiber consumption offered only partial protection against steatosis in male adult offspring and did not reach statistical significance (p = 0.07). In a previous study in male rats, consumption of yellow-pea flour, but not fiber, resulted in a lower hepatic TG concentration. Reference Eslinger, Eller and Reimer44 Additionally, we recently reported that maternal consumption of a yellow-pea protein-supplemented diet throughout pregnancy and lactation protected against hepatic TG accumulation in male offspring. Reference Rideout, Andreani and Pembroke21 Hence, the benefits of yellow peas with respect to hepatic steatosis are likely fraction-specific and the greatest potential benefits may be realized through consumption of whole yellow peas or by combining different yellow-pea fractions to obtain a specific desired metabolic effect. Interestingly, a recent longitudinal study in humans using multiple 24-h maternal pregnancy diet recalls and MRI assessment of hepatic fat in children (4–8 years old) reported that higher maternal fiber intake was associated with lower hepatic fat in offspring. This beneficial association was observed for total dietary fiber intake, however, the potential effects of different fiber types (i.e., soluble vs. insoluble) or food fiber source were not assessed. Reference Cohen, Perng and Sauder67
In summary, our findings suggest that maternal obesity programs a sexual dimorphic obesogenic response in adult male offspring that cannot be rescued through the postnatal consumption of a low-calorie diet. Additionally, offspring from obese pregnancies showed evidence of reduced glycemic control (both males and females) and dyslipidemia (males only). Supplementation of a maternal high calorie diet with yellow-pea fiber in prepregnancy and throughout gestation and lactation protects male offspring from metabolic dysfunction in the absence of any change in body weight status in adulthood.
Acknowledgments
We would like to thank the staff of the Laboratory Animal Facility at the University at Buffalo for their assistance in the care and maintenance of the animals involved in this study.
Financial support
This research was supported by the United States Department of Agriculture, USDA Pulse Crop Health Initiative (58-3060-0-049, to TCR).
Competing interests
None.