Introduction
Taste perception plays a significant role in food preference and determines the intake of foods with pleasurable taste while evading those that are unpleasant. Taste perception is mediated through binding of tastants with their specific receptors and this process is altered by the molecules that interrupt their interaction. Processing of taste information is crucial for facilitating food preference and creating familiar dietetic habits(Reference Kral and Rauh1). Taste modalities such as umami and sweet contribute to an evolutionary role in nutrition as a selective supplier of proteins and energy-rich diet, respectively. Sour and bitter tastes are involved in the evasion of detrimental or spoiled foods(Reference Breslin and Spector2). Salty taste ensures proper dietary electrolyte balance. In addition, recent compelling evidence demonstrates that lipids can be perceived by definite receptors in taste cells and, hence, fat taste is slowly attaining the status of a sixth taste modality(Reference Gaillard, Laugerette and Darcel3–Reference Subramaniam, Ozdener and Abdoul-Azize5).
The incidence of obesity and obesity-associated disorders such as type 2 diabetes and the metabolic syndrome has increased pointedly in the previous decades, attained epidemic levels and consequently is becoming a most important worldwide health issue(Reference Ng, Fleming and Robinson6). Increasing concerns regarding the health and quality of life have inspired individuals to prevent the consumption of foods that are rich in fat or sugar(Reference Romagny, Ginon and Salles7, Reference Brug8). Hence, identifying taste in food choice and analysing consumption behaviour will help in understanding the fundamentals involved in body weight maintenance and the development of obesity.
A taste mimetic is a substance that possesses the distinctive organoleptic qualities of a food product. On the other hand, taste modulators are substances that could either enhance or inhibit the perception of a specific taste modality(Reference DeSimone, DuBois, Lyall and Doty9). Modulation of taste responses may occur at numerous phases throughout the course of taste perception, which includes the interaction of tastant molecules with saliva(Reference Matsuo10). Both taste mimetics and taste modulators are being discovered as tools for basic taste research and have some absolute applications in the food industry as well as in the pharmacological industry. Drug discoveries for various medical conditions including asthma and respiratory tract infections(Reference Lee, Xiong and Kofonow11–Reference Deshpande, Wang and McIlmoyle13), CVD(Reference Foster, Porrello and Purdue14) and metabolic disorders(Reference Dotson, Zhang and Xu15) are based on taste modulators. In the present review, we focus on both the taste mimetics and taste modulators that reliably modify various taste responses at the receptor level.
Sweet taste
The taste receptors T1R2 and T1R3 (human taste receptor type 1 members 2 and 3) are the principal receptors for sweet taste. These receptors can sense numerous, chemically varied sweetened compounds including both the natural and artificial non-energetic sweeteners, sweet-tasting proteins, natural sugars and certain specific D-amino acids(Reference Behrens, Meyerhof and Hellfritsch16–Reference Liman, Zhang and Montell18). In the absence of T1R2, homodimers of T1R3 respond to both monosaccharides as well as to disaccharides. These receptors belong to the class C G protein-coupled receptors (GPCR) family that has definite structures including an N-terminal extracellular domain linked to the seventh transmembrane domain through a sharp cysteine-rich domain(Reference Pin, Galvez and Prezeau19, Reference Vigues, Dotson and Munger20). This family of GPCR contain a bilobate domain with two lobes being separated by a cleft(Reference Liu, Ha and Meng21, Reference Assadi-Porter, Maillet and Radek22). The stimulation of sweet taste receptors, irrespective of their expression pattern either in the taste bud cells of the tongue or in other areas of the oral cavity, results in the activation of α-gustducin. Phospholipase C β2 is subsequently stimulated, leading to the release of stored intracellular Ca2+ and activation of transient receptor potential melastatin 5 (TRPM5) (Fig. 1). This sequence results in membrane depolarisation and the release of neurotransmitters, which can then activate afferent sensory neurons that send signals to brain centres involved in taste perception(Reference Zhang, Hoon and Chandrashekar23).
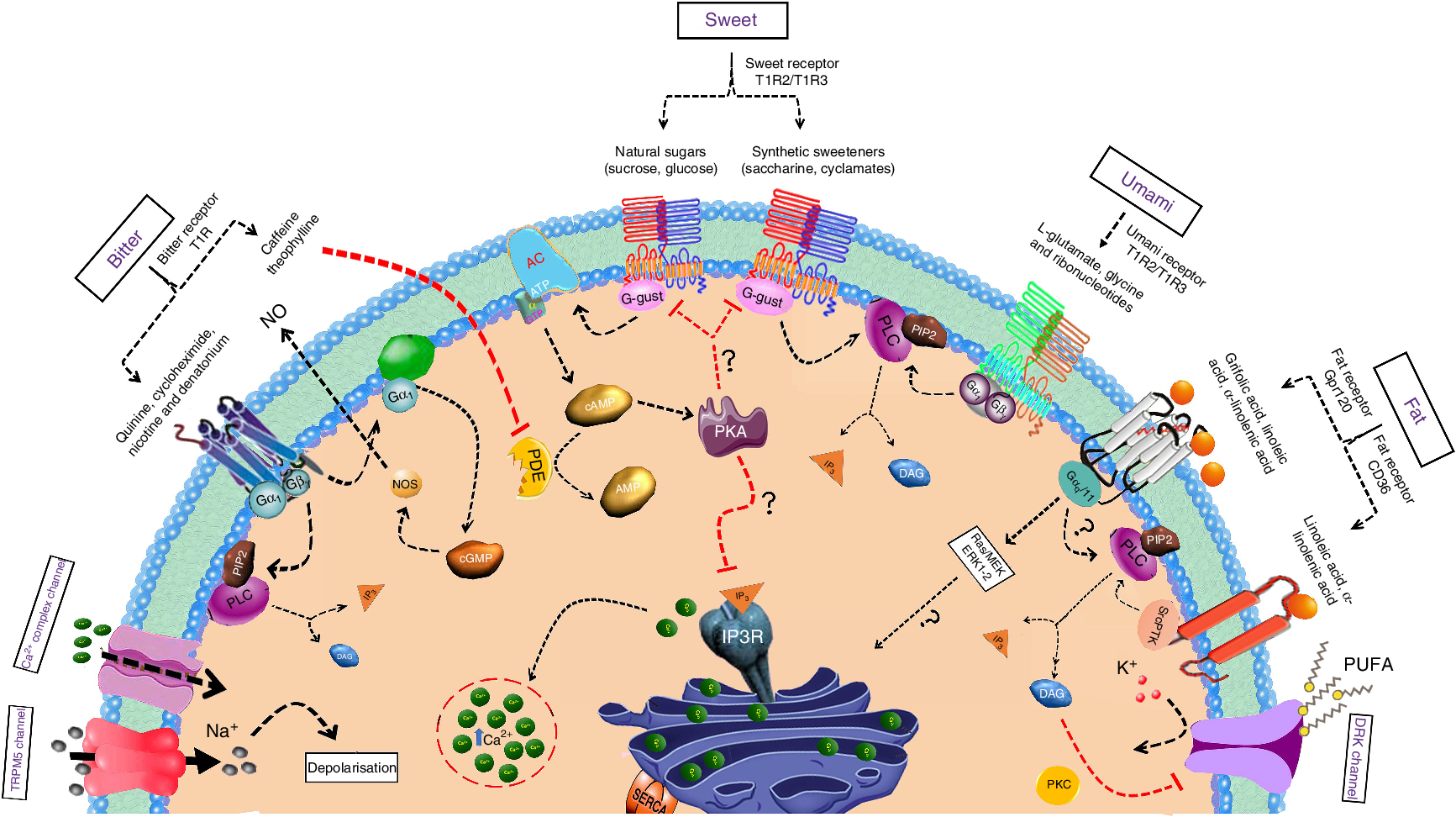
Fig. 1. Mechanisms of sweet, umami, bitter and fat taste perception by taste bud cells. The taste qualities of sweet, umami or bitter are sensed by the G protein-coupled receptor (GPCR) expressed on type II taste bud cells. Whereas, fat taste perception is mediated by both GPCR and cluster of differentiation 36 (CD36). Upon activation of GPCR, the signal is transmitted through βγ subunits Gβγ and α-gustducin to the downstream effectors phospholipase C β2 (PLC) and adenylate cyclase (AC), respectively. PLC β2 activation generates two second messengers, namely, diacylglycerol (DAG) and inositol 1,4,5-trisphosphate (IP3). IP3 acts on IP3 receptors on endoplasmic reticulum (ER) and increase the intracellular calcium levels. Whereas the AC mediates cyclic AMP (cAMP) production, which block the potassium channels on the plasma membrane. Both these pathways ultimately lead to the depolarisation of the taste receptor cells which lead to the opening of the transient receptor potential melastatin 5 (TRPM5) ion channel. Consequently, ATP is released through the Panx1 hemichannel into the extracellular space, which stimulates multiple targets including the gustatory afferent nerve fibres or the adjacent presynaptic cells which releases the neurotransmitters including serotonin (5-hydroxytryptamine; 5-HT) and noradrenaline into the synaptic cleft. PIP2, phosphatidylinositol 4,5-bisphosphate; T1R, taste receptor type 1; PDE, phosphodiesterase; PKA, protein kinase A; MEK, MAPK/ERK; MAPK, mitogen-activated-protein kinase; ERK, extracellular signal–regulated kinase; PKC, protein kinase C; DRK, delayed rectifying potassium; IP3R, IP3 receptor; SERCA, sarcoendoplasmic reticulum calcium transport ATPase. For a colour figure, see the online version of the article.
Sweet tastants and sweet taste modifiers
Sweeteners or sweet inducers are extensively used as dietary supplements, food additives and pharmaceuticals(Reference Mooradian, Smith and Tokuda24). Sweeteners can be classified into distinctive types according to their intrinsic properties, origin (artificial or natural), nutritional value (energy-containing or non-energy-containing), sweetness potency and their stability(Reference Carocho, Morales and Ferreira25).
The most common natural sweeteners are neohesperidine, steviol glycosides, thaumatin and dihydrochalcone. A bioengineered synthesis pathway concerning glucosyltransferase optimisation using Saccharomyces cerevisiae has led to a new generation of stevia sweeteners(Reference Olsson, Carlsen and Semmler26). Among steviol glucosides, two molecules, namely, rebaudioside A and stevioside, have been established as standard sweeteners(Reference Aranda-Gonzalez, Barbosa-Martin and Toraya-Aviles27, Reference Samuel, Ayoob and Magnuson28). In addition, cycloartane-type saponins, namely, abrusosides from the leaves of Camellia sinensis and Abrus precatorius L., could serve as effective sweet tastants(Reference Stavrianidi, Stekolshchikova and Rodin29). Accordingly, the sweet-tasting proteins can also be perceived as promising natural sweeteners due to their high sweet effectiveness and sensory properties. The plant proteins, namely, brazzein, thaumatin, neoculin, monellin and miraculin, have been recently identified as sweet-tasting molecules(Reference Kant30). Among these, miraculin and neoculin have the uncommon, rare property of adapting sourness into sweetness. Moreover, sensory analysis has revealed that miraculin could represent a natural sugar mimetic used in ancillary beverages(Reference Rodrigues, Andrade and Bastos31). However, the leading problem deterring in the use of sweet-tasting proteins in diet applications is the obstacle of gaining proteins from their natural source.
In addition to the natural sweeteners, artificial sweeteners are also currently in use and the prominent ones are acesulfame K, cyclamates, aspartame, saccharin, neotame and sucralose(Reference Godshall32). Among these, sucralose and neotame are chlorinated derivatives of sucrose and aspartame, respectively (Table 1). In addition, a recently developed dipeptide, advantame, is an excellent heat-stable sweetener that is 20 000 times sweeter than sucrose(Reference Bishay, Bursey and Nabors33).
Table 1. Characteristics of candidate sweet and bitter taste modifiers/tastants and their associated mechanism of chemoreception

TRPM5, transient receptor potential melastatin 5; MCL, miraculin; T1R2, taste receptor type 1 member 2; NCL, neoculin; ACK, acesulfame K; ASP, aspartame; GPCR, G protein-coupled receptor; GIV3727, 4-(2,2,3-trimethylcyclopentyl) butanoic acid; T2R, taste receptor type 2 (bitter taste receptor); GABA, γ-aminobutyric acid; BCML, Nα, Nα-bis (carboxymethyl)-l-lysine; ABA, abscisic acid.
Sweet taste receptors are subjected to both positive and negative allosteric modulation. Screening of the compound library for molecules that positively regulate the in vitro responses of the heterologous sweet taste receptors led to the identification of a sweet taste-enhancing molecule(Reference Servant, Tachdjian and Tang34, Reference Zhang, Klebansky and Fine35). This substance, termed as ‘SE-1’, predominantly enhanced the stimulation of the sweet taste receptor if intensified with sucralose; however, it displayed inadequate or negative modulation when tested with other sweet taste substances(Reference Servant, Tachdjian and Tang34). The structural variation of SE-1 developed by molecular modelling and site-directed mutagenesis gave rise to strong derivatives, which are labelled as SE-2 and SE-3, that are slightly increased in sweetness compared with sucrose(Reference Zhang, Klebansky and Fine35). In addition, polyols are the larger consumed fraction of sweet enhancers due to their lack of cariogenic properties(Reference O’Brien Nabors and Nabors36, Reference Evrendilek, Varzakas, Labropoulos and Anestis37). Therefore, polyols have been used as food additives that effect the hydrogenation of reducing sugars which are found naturally in vegetables and fruits. Many of the polyols including sorbitol, maltitol, mannitol, isomaltose, lactitol, erythritol and xylitol act as effective sweet modifiers.
In contrast, some other compounds inhibit the activity of sweet taste receptors (Table 1). Lactisole is one such compound that inhibits the human sweet taste receptor but not that of the rodent(Reference Sclafani and Pérez38). It was shown that this substance does not bind to the sweet taste receptor subunit T1R2, but instead interacts with T1R3(Reference Jiang, Cui and Zhao39). In addition, compounds isolated from the leaves of the plant Gymnema sylvestre, namely, gymnemic acid and gurmarin peptide, are also sweetness-suppressing molecules that act specifically on rodent sweet taste receptors(Reference Kamei, Takano and Miyasaka40, Reference Glaser, Hellekant and Brouwer41).
Bitter taste
Bitter taste is normally considered to be an unfavourable taste attribute in most food products and elicits a stereotypical innate response by mammals to avert consumption of harmful food constituents. Bitter taste is identified by the receptors that are encoded by the Tas2r gene family expressed in type II taste bud cells and have a sequence length of about 300–330 amino acids with a short extracellular N-terminus. Bitter taste receptors (taste receptors type 2; T2R) belong to class A GPCR and have ligand-binding sites in their transmembrane sections(Reference Adler, Hoon and Mueller42). In taste bud cells, T1R (which sense umami and sweet tastes) and T2R are generally expressed in a non-overlapping array(Reference Meyerhof, Batram and Kuhn43), implying a partition of receptor cells that detect appetitive v. aversive stimuli. In contrast to T1R, the T2R are commonly believed to act as monomers; nevertheless, current evidence proposes that they may also form heterodimers(Reference Kuhn, Bufe and Batram44). The broad and overlapping range of ligand sensitivities of T2R assures that this family of receptors responds to an enormous range of bitter-tasting chemicals.
Bitter taste signalling is initiated by the interaction of the ligand with its cognate T2R that brings a conformational change in the receptor and triggers the heterotrimeric intracellular G-protein complex(Reference Chaudhari and Roper45). When activated, Gα-gustducin becomes relieved from the complex and activates phospholipase C β2 (PLC β2)(Reference Caicedo, Pereira and Margolskee46) (Fig. 1). PLC β2 further acts on phosphatidylinositol-4,5-diphosphate to generate diacylglycerol and inositol 1,4,5-trisphosphate (IP3). The generated IP3 releases Ca2+ ions from the endoplasmic reticulum and opens the TRPM5 which leads to membrane depolarisation and subsequent neurotransmitter release(Reference Chaudhari and Roper45, Reference Hofmann, Chubanov and Gudermann47).
Bitter tastants/modifiers
A widespread variety of structurally diverse compounds can trigger T2R; however, the efficacy of most of them remains to be pharmacologically determined(Reference Devillier, Naline and Grassin-Delyle48, Reference Wiener, Shudler and Levit49) (Table 1). A recent assessment of a bitter compound library comprising both synthetic and natural bitter substances gave rise to the classification of twenty-eight T2R46 agonists, thirty-three T2R14 agonists, and thirty-two T2R10 agonists. The collective activity of all the three receptors is sufficient to perceive half of the tested substances, signifying that these receptors work as ‘generalists’ in the detection of most bitter substances(Reference Meyerhof, Batram and Kuhn43).
A recent study analysed both the promiscuity and selectivity of bitter ligands for human T2R(Reference Di Pizio and Niv50). In this report, the authors proposed that promiscuous bitter compounds activate all the selective T2R while both selective and promiscuous compounds can activate promiscuous T2R. However, no compound is known to activate all twenty-five T2R or no unique compound towards a selective T2R(Reference Meyerhof, Batram and Kuhn43, Reference Di Pizio and Niv50).
4-(2,2,3-Trimethylcyclopentyl) butanoic acid (GIV3727) was the first T2R inhibitor discovered by employing the high-throughput screening approach of 17 854 compounds, which specifically acts as a competitive inhibitor for T2R31 against acesulfame K(Reference Slack, Brockhoff and Batram51). The site-directed mutagenesis and molecular modelling revealed that the binding site of the bitter taste receptors is overlaid with that of the bitter agonists and therefore, this finding describes the competitive mechanism of action and the perceived selectivity of GIV3727 for an individual subset of the human bitter taste receptors(Reference Brockhoff, Behrens and Roudnitzky52). Additionally, probenecid, which was formerly known as an anion transporter channel inhibitor, mostly used as a uricosuric drug(Reference Stamp, O’Donnell and Chapman53), was suggested as a bitter taste blocker(Reference Brockhoff, Behrens and Roudnitzky52, Reference Greene, Alarcon and Thomas54). Moreover, enterodiol, a T2R inhibitor that has appeared to conceal the bitter taste of caffeine, inhibits numerous human bitter taste receptors(Reference Ley, Dessoy and Paetz55). This molecule was recognised by the examination of compounds structurally related to the known bitter masking molecule homoeriodictyol. In addition, 3β-hydroxydihydrocostunolide (3HDC) and 3β-hydroxypelenolide (3HP), natural sesquiterpene lactones from edible plants, were recognised as bitter taste receptor blockers which block the responses of T2R46(Reference Brockhoff, Behrens and Roudnitzky52). Ley et al. (Reference Ley, Dessoy and Paetz55) in their in silico docking experimentations proposed that the attachment of enterodiol to T2R10 may make an impact on the observed bitter masking effect. Recently, Nα,Nα-bis (carboxymethyl)-l-lysine (BCML), γ-aminobutyric acid and (+)-S-abscisic acid are suggested as competitive inhibitors of activated T2R4(Reference Pydi, Sobotkiewicz and Billakanti56). Among them, BCML was considered as a highly effective T2R antagonist reported until now. Besides, studies classified various effective bitter taste inhibitors such as 5’-AMP, sodium acetate, monosodium glutamate (MSG), and sodium gluconate to inhibit whey protein hydrolysate and offer insights on potential bitter taste inhibitors for the product applications associated with whey protein hydrolysate.
More recently, Kim et al. (Reference Kim, Kim and Jin Son57) reported on the active umami fraction (F05) of modernised Korean soya sauce and its bitter masking effect on human bitter-taste sensory receptor-expressing cells. This active umami fraction (F05) reduced the human-perceived bitterness along with efficient repression of the intracellular Ca2+ response induced by caffeine in the hT2R46 and hT2R43 bitter taste receptor-expressing cells. Both Glu-enriched oligopeptides and free amino acids are proposed to be vital in the effect of F05 on bitter taste receptors; F05 was also mixed with other bitter components like magnesium chloride and Gly-Leu that partly modulate the action of human bitter taste receptors.
In contrast, bitter-tasting tri-peptides are shown to be more effective in T2R1 stimulation. Among the peptides examined, the bitter tri-peptide Phe-Phe-Phe is the most potent in activating T2R1 with a half maximal effective concentration (EC50) value in the micromolar range(Reference Upadhyaya, Pydi and Singh58). In addition, Melis & Tomassini Barbarossa(Reference Melis and Tomassini Barbarossa59) examined the taste perception of bitter, sweet and umami with the modifications triggered by l-arginine (l-Arg) supplementation. The outcome proposes that l-Arg could be used as a strategic tool to modify taste responses that are related to eating behaviours. l-Arg can enrich the bitterness intensity of 6-n-propylthiouracil (PROP), whereas other reports(Reference Ahijevych, Tepper and Graham60, Reference Leksrisompong, Gerard and Lopetcharat61) have established a suppression of quinine’s bitterness.
Umami taste
The term ‘umami’ was coined in 1909 by Japanese chemist Kikunae Ikeda, and means ‘delicious savoury taste’(Reference Ikeda62). Binding of the umami tastant, such as free glutamate, in foods to the oral umami taste receptor triggers the umami taste sensation. The typical model of the umami receptor T1R1+T1R3 was reported by Temussi(Reference Temussi63) and Chandrashekar et al. (Reference Chandrashekar, Hoon and Ryba17); it was stated that T1R1 is considered critical for sensing umami taste(Reference Mouritsen and Khandelia64). The heterodimeric GPCR complex of T1R1 and T1R3 elicits the umami taste when interacting with amino acids, typically MSG, and this interaction occurs synergistically with the 5′-ribonucleotides GMP, IMP and AMP(Reference Nelson, Chandrashekar and Hoon65). Furthermore, mGluR1 and mGluR4 were also be identified as the probable receptor candidates for umami taste.
Ligand binding to the T1R1/T1R3 receptor activates Gβ3γ13, which in turn activates PLC β2 that catalyses the production of the second messengers IP3 and diacylglycerol. IP3 binds with IP3 receptor IP3R3 to induce the release of Ca2+ from intracellular stores (Fig. 1). The increase in intracellular Ca2+ subsequently activates TRPM5, which results in taste cell depolarisation and release of ATP that activates ionotropic purinergic receptors on gustatory afferent nerve fibres(Reference Roper66).
Brain mechanisms underlying the oral perception of umami taste have been well documented. Animal studies revealed that the facial (chorda tympani and greater superficial petrosal), glossopharyngeal and vagus (superior laryngeal) nerves, which make synapses with taste cells, convey umami taste information to the first relay nucleus, the rostral part of the nucleus of the solitary tract, and then the taste information is finally transferred to the insular cortex(Reference Sakai, Uneyama, Chavasit and Grumezescu67). The umami tastants such as MSG and IMP activate the same regions of the insular cortex that is known as a primary taste cortex in humans, suggesting that both umami tastants could be similarly recognised(Reference de Araujo, Rolls and Kringelbach68).
Umami tastant/modifiers
Umami tastants are very important for food seasoning and are widely used in food production. They show many health benefits, including reduction in fat deposition, inhibition of weight gain, and decrease in plasma leptin levels in rats(Reference Kondoh and Torii69, Reference Nakamura, Sanematsu and Ohta70). Umami tastants were also found to regulate gastrointestinal functions and to decrease the risk of stroke and CHD in adults by reducing Na intake in their diets(Reference Aburto, Hanson and Gutierrez71, Reference Bera, Kar and Yadav72). The umami taste preference is native and associated with protein-rich food uptake(Reference Chandrashekar, Hoon and Ryba17, Reference Chaudhari and Roper45). Several recent efforts motivated further studies to evaluate the taste properties of umami ingredients and to find new umami substances(Reference Kunishima, Shimada and Tsuji73, Reference Masic and Yeomans74) (Table 2).
Table 2. Characteristics of candidate umami and salt taste modifiers/tastants and their associated mechanism of chemoreception

MSG, monosodium glutamate; T1R, taste receptor type 1; TMD, transmembrane domain; NGCC, N-geranylcyclo propylcarboxamide; TRPV1t, transient receptor potential vanilloid 1; CNS, central nervous system; ENaC, epithelial Na channel.
MSG was the first molecule reported to have umami taste(Reference Ault75). Later, certain ribonucleotides such as IMP and GMP were discovered to have synergistic properties with MSG(Reference Yamaguchi76, Reference Zhang, Venkitasamy and Pan77). In addition, theanine, gallic acid, theogallin(Reference Kaneko, Kumazawa and Masuda78), N-acetylglycine(Reference Amado and Schlichtherle-Cerny79), pyroglutamyl peptides(Reference Martin, Hedwig and Michael80), glycopeptides(Reference Frerot and Benzi81) and succinoyl amides of amino acids(Reference Iwasaki, Miyamura and Kuroda82) were all reported to have umami taste. Alapyridaine(Reference Soldo, Blank and Hofmann83), which is a product of the Maillard reaction, and morelid(Reference Rotzoll, Dunkel and Hofmann84) found in morel mushrooms were also found to enhance umami taste.
Cairoli et al. (Reference Cairoli, Pieraccini and Sironi85) evaluated umami taste enhancement by the positive effect of sulfur substitution, where the umami taste enrichment was amplified if the methylene function of the alkyl chain linked to the exocyclic amino function of 5’-GMP was replaced by a sulfur atom. Furthermore, the umami-enhancing potential was declined if there was an oxidation of sulfur atoms to consequent sulfoxides(Reference Morelli, Manitto and Speranza86).
Moreover, along with these natural and synthesised umami taste-enhancing compounds(Reference Kondoh and Torii69, Reference Nakamura, Sanematsu and Ohta70), several other investigations demonstrated that a few peptide molecules produced from hydrolysates of fish protein, beef bouillon, or other foods, have umami taste(Reference Arai, Yamashita and Noguchi87–Reference Winkel, de Klerk and Visser89). Recently, Rhyu & Kim(Reference Rhyu and Kim90) found that low-molecular-weight acidic peptides (F-IV; 1000> MWP500) were the constituent that contributed to the umami taste of doenjang water extract. Further, Su et al. (Reference Su, Cui and Zheng91) found two novel umami taste-enhancing peptides, an octapeptide and an undecapeptide, from groundnut hydrolysate. Bagnasco et al. (Reference Bagnasco, Pappalardo and Meregaglia92) reported that medium-to-small size polypeptides contributed to the umami taste of hydrolysate of rice middlings.
Fat taste
Recently, there has been a massive upsurge of information and evidence on the oro-gustatory perception of fat taste. Reports indicate that improper oral fat detection may be associated with several complications, including obesity-induced lipotoxicity, diabetes, arterial hypertension, atherosclerosis, etc.(Reference Gurevich-Panigrahi, Panigrahi and Wiechec93). The detection of fat stimuli was thought to depend mostly on olfactory, textural and post-ingestive cues(Reference Besnard, Passilly-Degrace and Khan94). However, recently, research conducted predominantly on rodent models exposed an additional gustatory element for the detection of long-chain fatty acids(Reference Besnard, Passilly-Degrace and Khan94, Reference Gilbertson and Khan95). In mammals, oro-gustatory sensing of dietary fat is facilitated by fat taste receptors, namely, cluster of differentiation 36 (CD36) and GPR120/40, which are expressed in taste bud cells on circumvallate papillae, fungiform papillae and foliate papillae of the tongue epithelium(Reference Ozdener, Subramaniam and Sundaresan4, Reference Subramaniam, Ozdener and Abdoul-Azize5). Fat taste perception involves Ca signalling downstream to the activation of CD36 and GPR120/40, stromal interaction molecule 1-mediated opening of store-operated Ca channels, the release of neurotransmitters from taste bud cells and, finally, stimulation of afferent nerve fibres that transmit the signals to the brain(Reference Ozdener, Subramaniam and Sundaresan4, Reference Abdoul-Azize, Selvakumar and Sadou96). Our recent report suggests that the activation of extracellular signal-regulated kinase signalling cascade downstream to the activation of CD36 by fatty acids regulates Ca homeostasis modulator 1 (CALHM1)-mediated Ca signalling in both human and mouse taste bud cells(Reference Subramaniam, Ozdener and Abdoul-Azize5). TRPM5, a monovalent, non-selective cation channel, is reported to be a probable contributor of fat taste signalling(Reference Liu, Shah and Croasdell97). In addition, the delayed rectifying K channels expressed in taste bud cells are inhibited by PUFA in the diet and this supports their involvement in fat taste perception (Fig. 1). Moreover, toll-like receptor 4 signalling has recently been reported to stimulate consumption of obesogenic foods that are rich in fat and sugar(Reference Camandola and Mattson98).
Fat tastants/modifiers
Like sweet tastants, fat tastants are molecules that may be synthesised in the laboratory or purified from plants that imitate the purpose of fat taste by binding to fat taste receptors (online Supplementary Table S1). Recent research had foreseen that the fatty acid-activated CD36 and GPCR might be the potential target of plant-driven tastants eliciting the fat taste sensation devoid of having any energy value(Reference Dramane, Akpona and Simonin99). CD36 and GPR120 agonism with grifolic acid (GA) has been shown to elicit intracellular Ca signalling in both human and mouse taste bud cells(Reference Ozdener, Subramaniam and Sundaresan4). Several selective ligands for free fatty acid (FFA) receptors have subsequently advanced as plausible remedies for type 2 diabetes(Reference Vangaveti, Shashidhar and Jarrod100–Reference Zhang and Leung102). Therefore, many enduring academic programmes and industries are driven by the aim of improving selective and potent agonists for FFA receptors. Godinot et al.(Reference Godinot, Yasumatsu and Barcos103) synthesised effective agonists for the fat taste receptors GPR120 and GPR40 in mice, which trigger the glossopharyngeal nerve through binding to the receptor. In humans, various reports derived from triangle tests and two-alternative forced choice, and sensory profiling demonstrate that GPR40 agonists were perceived in sip-and-spit tests and bring out a taste similar to that of linoleic acid(Reference Godinot, Yasumatsu and Barcos103). A vastly convincing FFA1 agonist TUG-770, with its promising pharmacokinetic and physico-chemical properties, displayed increased glucose tolerance in diet-induced obese mice(Reference Christiansen, Hansen and Urban104). Further, through mutational and modelling efforts, the dual synthetic agonists of GPR120/40, including GW9508, NCG21 and NCG46, are emerging as novel ligands with improved pharmacological properties for the fat receptors.
In 2018, Melis et al.(Reference Melis, Mastinu and Arca105) showed that the alterations of oleic acid perception stimulated by the administration of l-Arg are associated with the PROP taster status of subjects and common variants in CD36. Moreover, the low concentration supplementation of l-Arg governed an upsurge in perceived intensity of oleic acid, mostly in medium tasters and PROP non-tasters. Sihag & Jones(Reference Sihag and Jones106) found that oleoylethanolamide, which acts as an effective agonist of PPAR-α, potentially modifies the expression of CD36 and thereby alters fat taste perception. Consequently, a certain group of hybrid composites of thiazolidinedione PPARγ agonists also revealed therapeutic prospective beyond antidiabetic activity(Reference Thangavel, Al Bratty and Akhtar Javed107). In addition, Sasaki et al. (Reference Sasaki, Yasoshima and Matsui108) identified that both intraperitoneal (IP) and orally administered d-serine affect feeding behaviour; especially, IP-injected d-serine prevented the acquisition of a preference for high-fat diets. Furthermore, Murtaza et al.(Reference Murtaza, Berrichi and Bennamar109) presented the first evidence for the modulation of fat taste perception in human taste bud cells (hTBC) by ziziphin, purified from the edible fruit of Zizyphus lotus, indicating the possibility of using these compounds as a fat taste modifier/fat taste mimetic.
Salt taste
Salty taste in mammals is triggered by two different pathways termed as the amiloride-sensitive (AS) pathway and the amiloride-insensitive (AI), or high-salt, pathway. The former selectively reacts to Na and Li salts, which is facilitated by the epithelial Na channel (ENaC)(Reference Heck, Mierson and DeSimone110–Reference Roper112). However, the latter reacts to a wide scale of Na and non-Na salts(Reference Ninomiya and Funakoshi113, Reference Halpern114). In rodents, about 65 % of fungiform papillae cells and 35 % of foliate papillae cells displayed efficient amiloride-sensitive Na+ currents, whereas the circumvallate papillae cells are absolutely insensitive to amiloride even though amiloride-sensitive Na+ channel proteins and ENaCα mRNA have been spotted in those cells(Reference Shigemura, Islam and Sadamitsu115). The AI pathway responses have been described in both a subpopulation of type II bitter taste cells and polycystic kidney disease 2-like 1 protein (PKD2L1)-expressing type III taste cells, which is essential for sour taste perception(Reference Oka, Butnaru and von Buchholtz116).
The degree of co-occurrence between sour and AI salt responses in type III taste cells is still undefined due to the frequency of cell-to-cell signal transmission in the taste bud(Reference Roper117). Earlier reports have shown that the type III taste cells are essential for both AI salt taste and sour taste(Reference Oka, Butnaru and von Buchholtz116, Reference Huang, Spielmeyer and Lagudah118–Reference Yoshida, Miyauchi and Yasuo120); nevertheless, it was uncertain whether the expression of these taste receptors was exhibited by distinct or similar populations of taste cells. Furthermore, previous studies have shown that the AI salt taste responses originated in a subset of both sour-responsive taste cells and bitter-sensitive type II cells(Reference Oka, Butnaru and von Buchholtz116, Reference Lewandowski, Sukumaran and Margolskee121). These findings suggest that the AI salt taste response perception depends mostly on the collective stimulus of different taste cell populations which determine further distinct bitter or sour taste qualities.
Several reports indicated that NaCl-mediated induction and its relative contributions depend on the concentration of Na(Reference Tordoff, Aleman and McCaughey122, Reference Taruno, Vingtdeux and Ohmoto123). Accordingly, at lower Na concentrations, there is no direct association of CALHM1 but there is an infusion of Na within ENaC located in taste bud cells(Reference Ishiwatari and Bachmanov124, Reference Cherukuri, Bachmanov and McCaughey125) (Fig. 2). At higher Na concentrations, CALHM1 participates in an even more vital role in the neural response. However, further studies will be required to ascertain whether this is due to the stimulation of elevated thresholds of salt receptors in type II cells or due to other Na-receptive cell types that cooperate with type II cells.

Fig. 2. Mechanisms of sour and salt taste perception by taste bud cells. Sour taste is triggered in type III cells by the intracellular proton concentration change triggered by protonated acids. In addition, several channels, including polycystin 2 like 1 (PKD2L1; transient receptor potential cation channel) and PKD1L3 have also been associated with sour taste. For salt taste, the putative candidate is the epithelial-type sodium channel (ENaC). The principal salt stimulus (sodium ion; Na+) can permeate through these cation channels on the apical surface of taste bud cells and trigger depolarisation. ΔVm, membrane potential change; TRPP3, transient receptor potential polycystic 3. For a colour figure, see the online version of the article.
Salt tastants/modifiers
The extreme consumption of salt in the diet is a universal health issue. Various efforts have been made to focus on this issue, involving the evolution of salt substitutes (Table 2) and mounting strategies to lower salt intake(Reference Henney, Taylor and Boon126). Salty taste is chiefly prompted by Na+, which is the simple cation known to elicit a pure salt taste transduction in humans. Other than sodium chloride, various mineral and organic salts provoke a salty taste but to a minor extent(Reference van der Klaauw and Smith127). Potassium chloride is a promising candidate that acts as a substitute for sodium chloride in low-salt foods(Reference Barat, Allende and Aliño128–Reference Toldrá and Barat130). However, it has a vulnerable salty taste compared with sodium chloride and, in addition, when used in excessive quantities, it is often linked with bitterness. Other substitutes such as sodium gluconate and ammonium chloride are also recommended but reveal the similar complication of association with bitterness when reacting with KCl, which limits their usage.
Nakagawa et al. (Reference Nakagawa, Kohori and Koike131) reported that sodium aspartate is a potent enhancer of salt taste perception. They proposed a research model based on their study results that the sodium aspartate-induced conformational change on ionic channels enhance NaCl and KCl perception. The enhancement of salt taste transduction by sodium aspartate was also confirmed by human sensory assessments.
The compound (+)-(S)-alapyridaine has universal taste-enhancing properties. When alapyridaine was introduced in the sensory triangle test, the threshold concentrations for the umami taste of MSG and GMP, for the sweet taste of sucrose and glucose, along with the salty taste of NaCl, were considerably decreased. However, on the other hand, the bitter taste perception of l-phenylalanine and caffeine, along with the sour taste of citric acid, was unaltered(Reference Soldo, Blank and Hofmann83). Hence, the taste-enhancing properties of alapyridaine in umami and saltiness prompt developments in the production of low-Na foods for hypertensive patients. Furthermore, Kim et al. (Reference Kim, Son and Kim132) proposed a unique synthetic compound (N-geranylcyclopropylcarboxamide), which modulates amiloride-insensitive Na+-opening pathways, thereby characterised as a salt taste enhancer. More importantly, as per their report, the N-geranylcyclopropylcarboxamide concentration at which it greatly enhanced the benzamil-insensitive Na+ chorda tympani response in rodents also improved the taste perception of NaCl solutions (60–80 mm) in human subjects. Additionally, choline chloride is also proposed to be a salt taste enhancer(Reference Locke and Fielding133) and several choline-containing compounds were synthesised to be used as salt taste enhancers(Reference Fielding and Locke134). Nevertheless, chlorhexidin (an antiseptic compound) has been revealed to impede salt taste perception generated by the chloride salts of Na, K, ammonium and Li.
Taste-enriching peptides are also used to enhance salt taste perception and hence diminish the usage of sodium chloride content in foods. Kino & Kino(Reference Kino and Kino135) synthesised an effective salty taste-enriching dipeptide, Met-Gly, using l-amino acid ligase (Lal) of BL00235 (Lal from Bacillus licheniformis) or TabS (Lal from Pseudomonas syringae) by site-directed mutagenesis based on the perceived crystal structure.
Sour taste
Weak organic acids tend to diffuse through the plasma membrane as neutral molecules in sour taste transduction and dissociate inside the cytoplasm, which causes intracellular acidification(Reference Lyall, Alam and Phan136). However, strong acids depolarise the sour taste receptor cells either by the inhibition of K+ channels or by the stimulation of voltage-gated Na+ channels(Reference Chaudhari and Roper45) (Fig. 2). However, both these mechanisms might result in the opening of voltage-gated Ca2+ channels and stimulate the discharge of neurotransmitters against adjacent afferent nerves.
Sour tastants/modifiers
In sour taste transduction, several ion channels have been predicted to function as mediators despite the fact that the genetics of sour taste perception are inadequately recognised. Although protons signify a dominant part of the sour stimulus, the precise nature of the sour taste stimulus is still under dispute. It was established that weak organic acids in their undissociated form can competently pervade the taste cell plasma membrane and possibly will reduce the intracellular pH values near the cell surface(Reference Huang, Maruyama and Stimac137). Recently, Ohishi et al. (Reference Ohishi, Nishida and Miyamoto138) examined the potentials of the bortezomib-induced taste condition in mice and reported that the sensitivity of sour taste was drastically increased by the administration of bortezomib (online Supplementary Table S1). Moreover, PKD2L1 expression was increased in bortezomib-administered mice, and on cessation of its administration its effect reverted to the control level. Hence, these effects propose that the increase in PKD2L1 protein expression develops sour taste sensitivity in bortezomib-administered mice, and this modification is reversed on termination of its administration. Alternatively, Ishii et al.(Reference Ishii, Kurokawa and Kishi139) suggested that capsaicin can be exploited as an inhibitor of PKD2L1 and PKD1L3, which signifies that in a medical condition, pre-treatment with capsaicin will probably lessen sour taste sensitivity.
Conclusions and future perspectives
Our diets differ based on numerous influences such as environment, our culture and health. At the molecular level, individuals perceive different taste modalities with the help of a range of specified tissues that direct sensory receptors to control nutritious value. In general, the interplay between trigeminal, olfactory and gustatory sensation is interpreted as taste perception like sweet, bitter, umami, salty and sour. Apart from these five principal taste behaviours, the taste system perceives certain non-canonical senses of orosensory taste stimuli such as fat, kokumi, complex carbohydrates and water that prove the existence of additional taste modalities. Current investigations foretold the plant-driven taste modulators which possibly target fatty acid taste receptors (CD36 and GPCR) and provoke a taste sensation devoid of any energy value that may be used against dietary-induced obesity.
Moreover, while the molecular characterisation of modulators and activators for the umami and sweet taste receptors has been carried out, the precise relationship among the T1R heteromeric subunits has yet to be characterised. In the near future, to combat against obesity and other related metabolic syndromes, rigorous strategies should be developed to construct libraries of plant-derived or chemical taste modifiers that would adhere to taste receptors and prompt a façade gustatory sense. In order to achieve this goal, we should attain a comprehensive understanding of the entire oro-gustatory receptors and their respective plant-derived/chemical compounds that are able to activate the gustatory system at the cellular and neurological levels. Likewise, the further understanding of combined biochemical properties of tastants and their inter-species transformations in chemosensory signal detection could be improved.
Furthermore, there is a need for modern contemporary analytical technologies to accelerate the detection of unidentified active chemosensory molecules present in nature and to recognise their physico-chemical relations on a molecular level with food matrix ingredients. The existing complication to gain sensors with suitable sensitivity and selectivity for the examination of taste modulators is directed to the concept of electronic tongues. For example, the electronic bio-mimetic tongue is capable of validating the projected taste intensity of unidentified taste modulators.
Advancement in examination and screening of specific taste-modulating compounds may offer novel platforms for checking the taste of drug candidates and in food quality control. Nowadays, a major challenge in taste research is to overcome the flavour defects in nutritious food products and production of natural or biosynthetic, non-energy-containing fat/sugar mimetics as well as bitter maskers. Moreover, thorough understanding is needed in distinctive age-dependent chemosensory genotypic and phenotypic variances in sensory preference for aversion against food flavours. Further research is essential to enhance our understanding towards the molecular adaptations of salivary composition upon stimulation with tastants along with the receptor proteins included in perceiving tastants. This knowledge will help in developing methodological questions regarding the sequential profile of taste modulators and their biased agonism, which may lead to recognise broadly adjusted enhancers like positive allosteric modulators or inhibitors such as negative allosteric modulators for industrial or commercial applications.
Author ORCIDs
Shanmugamprema Deepankumar 0000-0002-0043-3864, Subramaniam Selvakumar 0000-0003-3798-2899
Supplementary material
The supplementary material for this article can be found at https://doi.org/10.1017/S0954422419000118
Acknowledgements
Financial support provided by the DST-SERB (ECR/20l6/001101) and UGC (BSR startup grant no. F.30-354/2017) India is greatly acknowledged.
S. D. and S. S. planned the graphical abstract and wrote the article. M. K. and K. V. wrote selected parts of the review and facilitated in the formulation of table.
The authors declare no conflicts of interests.