Gut microbiota and its relevance for health
Until recently the human organism has been treated as an exclusively eukaryotic metazoan, whereby the studies of nutrition, health and non-infectious disease were challenging but well defined in terms of cells and external factors. A technological revolution spurred by innovations in DNA sequencing technology after the Human Genome Project ironically provided insights into the complex community of bacteria that lives on and in the human body. It has been estimated that these bacteria outnumber human cells by a factor of 10 and human genes by a factor of 100( Reference Ley, Peterson and Gordon 1 ), and it is now widely accepted that these bacterial communities co-evolved with human subjects and other metazoans in a mutualistic if not truly symbiotic way. The evidence for this stems from the linkage of perturbed microbial communities with a range of symptoms, pathophysiologies and diseases. A microbial mechanism that might be involved in multiple such conditions is the documented ability of bacteria to convert dietary ingredients into bioavailable and/or bioactive metabolites, whose lack, due to altered microbiota, leads to symptomology. This paradigm can have more subtle long-term effects, including CVD, colorectal cancer and malnutrition. Expressed most simply, health studies restricted to diet and host alone have missed a major player in a holistic understanding of how diet affects health and disease. This review briefly summarises what we know about the composition and function of the gut microbiota, how it changes over the lifespan, how it relates to health and disease, and how realistic it might be to accomplish large-scale remodelling of the microbiota by dietary supplementation, in order to improve health.
Gut microbiota and its development throughout life
The collection of bacteria in a particular environment or site is termed the microbiota; the related and often confused term ‘microbiome’ refers to the collection of genes or coding capacity that a particular microbiota harbours. As noted earlier, our knowledge of the human microbiome and gut microbiome has expanded dramatically in the past decade because of culture-independent methodologies, whose main features and appropriateness for different research questions were recently reviewed( Reference Fraher, O'Toole and Quigley 2 ). Although it is well established that the mucosal and faecal microbiota differ in composition( Reference Eckburg, Bik and Bernstein 3 – Reference Araujo-Perez, McCoy and Okechukwu 5 ), most of our knowledge of gut microbiota is actually derived from studying faecal samples, for pragmatic reasons: colonoscopic biopsy sampling of healthy individuals is not ethical, whereas faecal samples are readily available. Estimates of the complexity of the faecal microbiota vary somewhat depending on the analytical method used, and the bioinformatic processing of the data, but the community is dominated by ten major divisions or phyla( Reference Rajilic-Stojanovic, Heilig and Molenaar 6 ), among which phylum Bacteroidetes and phylum Firmicutes together often constitute more than 90 % of the total population( 7 , Reference Qin, Li and Raes 8 ). The other major phyla are Proteobacteria, Actinobacteria, Verrucomicobia and Lentisphaera ( Reference Rajilic-Stojanovic, Heilig and Molenaar 6 – Reference Claesson, Cusack and O'Sullivan 9 ) (see Fig. 1). Two archaeal species, Methanobrevibacter smithii, predominantly, and Methanosphaera stadtmanae, are present in proportions not usually exceeding 1 % in total( Reference Mihajlovski, Dore and Levenez 10 ). Development of lower error amplicon sequencing strategies for ribosomal RNA genes has recently estimated that there are about 195 bacterial strains in the faecal gut microbiota, representing about 101 species( Reference Faith, Guruge and Charbonneau 11 ). It has been proposed that the gut microbiota of an individual can be assigned to one of the three so-called enterotypes, Bacteroides, Prevotella or Ruminococcus, depending on the abundance of these microbial genera( Reference Arumugam, Raes and Pelletier 12 ). There is some evidence that rather than a single dominating microbial type, microbiota datasets from large cohorts form gradients rather than discrete clusters( Reference Huse, Ye and Zhou 13 , Reference Jeffery, Claesson and O'Toole 14 ).
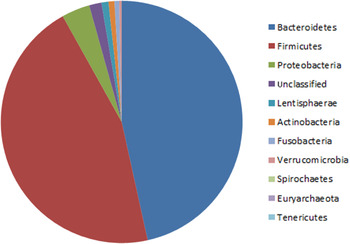
Fig. 1. (colour online) Phylum-level composition of the human adult faecal microbiota, derived from aggregated data of sixteen healthy controls. Phylogenetic assignments were made using the Ribosomal Database Project classifier as previously described( Reference Claesson, Cusack and O'Sullivan 9 ).
Initial studies of the faecal microbiota focused primarily on healthy adults and suggested that the dominant organisms were stably maintained in individuals( Reference Zoetendal, Akkermans and De Vos 15 ), an observation that has recently been confirmed( Reference Faith, Guruge and Charbonneau 11 ). This stability only occurred if the subjects were not receiving antibiotic treatment, and independent studies have amply demonstrated the significant alteration to microbiota composition caused by antibiotic therapy( Reference Dethlefsen, Huse and Sogin 16 – Reference Cho, Yamanishi and Cox 18 ). Although the faecal microbiota composition is stable in healthy adults, the two extremes of life, infancy and old age, are characterised by a gut microbiota in flux (reviewed also in( Reference O'Toole and Claesson 19 )). Infants are born essentially devoid of microbes, but are colonised by a series of displacements and successions for the first 2 years of life, until an adult-like microbiota has formed( Reference Adlerberth and Wold 20 – Reference Yatsunenko, Rey and Manary 23 ). A distinguishing feature in the infant gut microbiota is the predominance of phylum Actinobacteria, specifically Bifidobacterium spp.( Reference Turroni, Peano and Pass 24 ). However, the neonatal gut microbiota is very influenced by the mode of delivery, whereby infants delivered by a caesarean section initially have a gut microbiota more similar to the maternal skin and physical surroundings than a vaginal microbiota( Reference Dominguez-Bello, Costello and Contreras 25 ). There is considerable interest in the connection between the composition of the infant gut microbiota and late-life risk for asthma, allergy and autoimmune-type diseases (reviewed in( Reference Walker 26 )), particularly because of the soaring rate of births by caesarean section in many countries( Reference Niino 27 ). Also of particular interest is feeding modality, because human milk oligosaccharides are optimally degraded and metabolised by particular bifidobacteria present in greater levels in healthy vaginally delivered infants( Reference Sela and Mills 28 ), supporting the link between breast-feeding and particular bifidobacterial species and strains.
In old age, a process occurs that superficially resembles a mirror image of neonatal gut colonisation. Culture-dependent studies had suggested that the faecal microbiota of older subjects was different from that of younger adults (summarised in( Reference Woodmansey 29 )) but unifying trends in different studies, in different countries, were not identified( Reference Mueller, Saunier and Hanisch 30 ). Although the proportion of Bacteroidetes appeared elevated in some studies( Reference Hopkins and Macfarlane 31 ), it was reduced, and Bacteroides species diversity was reduced, in others( Reference Woodmansey, McMurdo and Macfarlane 32 ). Advancing age in human subjects is often accompanied by frailty, but also by persistent activation of the innate immune system, the so-called inflammaging, that may contribute to frailty( Reference Franceschi, Bonafe and Valensin 33 ). It was therefore interesting that altered microbiota was reported in frail older subjects( Reference van Tongeren, Slaets and Harmsen 34 ), although the number of subjects was relatively small. Proportions of lactobacilli were significantly depleted (twenty-sixfold), while the abundance was also reduced for the Bacteroides/Prevotella group (threefold) and the Faecalibacterium prausnitzii group (fourfold)( Reference van Tongeren, Slaets and Harmsen 34 ). Testing the hypothesis that altered gut microbiota in the elderly might be associated with health loss including frailty and inflammation was one of the aims of the ELDERMET project in Cork, Ireland (see later). Other groups using other culture-independent methods have also identified differences between the gut microbiota of younger v. older subjects. Rajilic-Stojanovic et al. reported increases in proportions of Actinobacteria (Actinomycetes and Atopobium), streptococci and lactobacilli in ten older subjects compared with ten younger, and reductions in proteobacteria and Bacteroides ( Reference Rajilic-Stojanovic, Heilig and Molenaar 6 ). The same phylogenetic microarray was used by Biagi et al. to study microbiota changes in older subjects and centenarians( Reference Biagi, Nylund and Candela 35 ). In the cluster containing the highest number of centenarians, the microbiota was characterised by higher proportions of Proteobacteria and Bacilli, and decreased levels of Clostridium cluster XIVa bacteria. Decreased Bacteroides abundance was not associated with ageing, and centenarians tended to harbour Archaea more frequently than the elderly or young adults( Reference Biagi, Nylund and Candela 35 ).
Gut microbiota alterations in disease
One of the drivers for research into gut microbiota is the fact that alterations in the normal composition or function have been linked to a wide range of diseases (reviewed in( Reference de Vos and de Vos 36 , Reference Sekirov, Russell and Antunes 37 )). It is beyond the scope of this review to describe in detail the evidence for these linkages. The diseases with the strongest evidence for microbiota linkages include obesity (see later), inflammatory bowel disease (IBD), type-2 diabetes, disease-related malnutrition, and there is increasing evidence for a colorectal cancer linkage. IBD can be described as an abnormal immune response to normal gut bacteria, in which pre-disposing genetic factors combine with environmental factors( Reference Manichanh, Borruel and Casellas 38 , Reference Jostins, Ripke and Weersma 39 ). The microbiota is different in IBD patients compared with controls( Reference Frank, St Amand and Feldman 40 , Reference Manichanh, Rigottier-Gois and Bonnaud 41 ), and loss of F. prausnitzii, which ameliorates symptoms in animal models of colitis( Reference Sokol, Pigneur and Watterlot 42 ), is a feature of the altered microbiota of some IBD patients. Microbiota alterations have been reported in type-2 diabetes( Reference Karlsson, Tremaroli and Nookaew 43 , Reference Qin, Li and Cai 44 ), and although mechanistic links have yet to be determined, it is relevant that faecal microbiota transplantation has proven effective for treating glucose insensitivity in patients with metabolic syndrome( Reference Vrieze, Van Nood and Holleman 45 ). Gordon and co-workers reported an intriguing synergy between a particular microbiota (associated with the severe malnutritional wasting disease Kwashiorkor) and environmental factors (nutrition and disease burden) to produce full symptoms of the disease( Reference Smith, Yatsunenko and Manary 46 ). Colon cancer is the number two ranking cancer diagnosis globally( Reference Jemal, Bray and Center 47 ), and there is an emerging literature linking the gut microbiota as a risk factor for colorectal cancer, particularly Fusobacterium nucelatum ( Reference Wu, Rhee and Albesiano 48 – Reference Kostic, Gevers and Pedamallu 52 ). For this and most of the other disease linkages, the precise microbial species and mechanisms are unknown. Once they are identified, the potential for nutrition to modulate lifetime risk for these diseases will be an exciting research endeavour.
The interaction of gut microbes with nutrition
The gut microbiota influences a number of nutrition-related functions, from digestion and energy provision, fermentation, lipid storage, to the production of specific micronutrients (Fig. 2)( Reference O'Connor 53 ). The preponderance of research focus has concentrated on dietary carbohydrate and lipid metabolism and their direct effects on the gut microbiota, but the gut microbiota also contributes to total host micronutrient production and status including a number of essential B vitamins (including folate, riboflavin, vitamin B12, niacin and pyridoxine( Reference LeBlanc, Milani and de Giori 54 )) and vitamin K( Reference Collins, Fernandez and Howarth 55 , Reference Mathers, Fernandez and Hill 56 ). In addition, other activities of the gut microbiota may have indirect diet-dependent interactions. For example, recent evidence suggests that differences in host genotype that affect the mucus carbohydrate composition of the distal gut interact with diet to alter the composition and function of resident microbes in a diet-dependent manner( Reference Kashyap, Marcobal and Ursell 57 ).
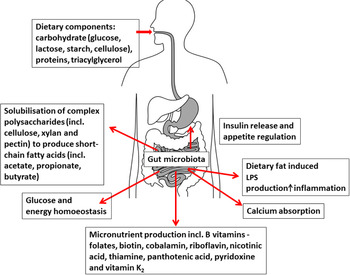
Fig. 2. (colour online) Interaction between diet, gut microbiota and nutritional status (taken with permission from( Reference O'Connor 53 )). LPS, lipopolysaccharide.
The gut microbiota and its metabolic activities respond to and alter according to habitual diet; this has been shown across a number of population subgroups, including the elderly. Age-related dietary changes can occur for several reasons, including loss of oral mechanical function, taste and appetite. These changes may result in an increased consumption of low-nutrient dense/high-fat/sugar foods and a reduction in dietary intakes of plant-based foods. The ELDERMET study recently reported different intestinal microbial populations among those aged over 65 years who consume a diet enriched in animal products and food with high glycaemic index. These individuals contained a greater abundance of Bacteroides and Alistipes species in their microbiota while those consuming a healthier diet (high in plant-based foods) harboured an abundance of bacteria from the genus Prevotella ( Reference Claesson, Jeffery and Conde 58 ).
These findings are in agreement with other findings, albeit in different population groups( Reference De Filippo, Cavalieri and Di Paola 59 , Reference Wu, Chen and Hoffmann 60 ). In the ELDERMET cohort, change of living environment from community to long-term residential care was associated with a dramatic change in dietary quality and diversity (indicated by a low Healthy Food Diversity index) which correlated with a subsequent reduction in gut microbiota diversity. In addition, those with poorer dietary and microbiota diversity had a concomitant decrease in functional independence (i.e. they were more frail), they had increased levels of serum cytokine markers of inflammation, lowered concentrations of SCFA in their faeces, as well as negative correlations with other clinical parameters( Reference Claesson, Jeffery and Conde 58 ).
Gut microbiota and lipid storage/metabolism
The gut microbiota is thought to influence lipid storage by affecting nutrient acquisition and energy regulation of the host, thereby playing a role in the development of obesity, insulin resistance and diabetes (for a detailed review, see( Reference Tremaroli and Backhed 61 )). As a product of fermentation, some gut microbes produce SCFA, which are important energy sources for the host. SCFA have been shown to regulate glucose homoeostasis by regulating the secretion of a number of gastrointestinal hormones including glucose-dependent insulinotropic polypeptide, glucagon-like peptide 1 (an incretin hormone, responsible for increased insulin secretion)( Reference Tolhurst, Heffron and Lam 62 ) and glucagon-like peptide 2, and peptide YY( Reference Lin, Frassetto and Kowalik 63 ). However, the molecular pathways underpinning these beneficial effects of SCFA are largely unknown. In addition, free fatty-acid receptors, located on the colonic enteroendocrine L-cells known as free fatty-acid receptor 2 and free fatty-acid receptor 3 (also known as G-protein-coupled receptor (GPR)43 and GPR41), are responsible for the release of glucagon-like peptide 1 among other anorectic hormones( Reference Ford and Frost 64 ). In a recent study, Kimura et al.( Reference Kimura, Ozawa and Inoue 65 ) showed that the SCFA receptor GPR43 links the metabolic activity of the gut microbiota with host body energy homoeostasis. GPR43-deficient mice were obese on a normal diet, whereas mice overexpressing GPR43 (and specifically in adipose tissue) remained lean even when fed a high-fat diet. A normal phenotype prevailed in those raised under germ-free conditions or after antibiotic treatment; however, SCFA-mediated activation of GPR43-suppressed insulin signalling in adipocytes, which inhibited lipid accumulation in adipose tissue and promoted the metabolism of unincorporated lipids and glucose in other tissues. These findings establish GPR43 as a sensor for excessive dietary energy, thereby controlling body energy utilisation while maintaining metabolic homoeostasis.
There are three main theories as to how the microbiota might be a determining factor for obesity: energy harvest, signalling and inflammation–adipogenesis. The earliest propounded of these theories was that the obesogenic microbiota was characterised by an altered Firmicutes: Bacteroidetes ratio. In fact, the contribution of various bacterial groups to the development of obesity in human subjects remains controversial with conflicting evidence regarding the representation of Bacteroidetes in obese and non-obese individuals. A study conducted with obese and non-obese adults on low-energy diets revealed that the relative proportion of Bacteroidetes was lower in obese individuals compared with lean subjects( Reference Ley, Turnbaugh and Klein 66 ). However, a subsequent study by Duncan et al.( Reference Duncan, Lobley and Holtrop 67 ) detected no difference in the proportion of Bacteroidetes measured in faecal samples from obese and lean individuals on weight-loss diets but did note a reduction in butyrate producing Firmicutes in obese subjects. In contrast, Schwiertz et al.( Reference Schwiertz, Taras and Schafer 68 ) found that the ratio of Firmicutes to Bacteroidetes changed in favour of the Bacteroidetes in overweight and obese subjects while recently Simoes et al.( Reference Simoes, Maukonen and Kaprio 69 ) showed that intakes of energy, MUFA, n-3 PUFA, n-6 PUFA and soluble fibre had significant associations with the stool bacterial numbers. In particular, increased energy intake was associated with reduced faecal proportions of Bacteroides spp. in monozygous twins. Finally, a recent interrogation of data from the Human Microbiome Project and MetaHIT (an eight-country cooperation between Europe and China investigating the role of the metagenome in nutritional status and chronic disease risk) from healthy and obese individuals confirmed differences in phylum-level taxonomic composition variability between lean and obese subjects but no association was found between BMI and taxonomic composition( Reference Finucane, Sharpton and Laurent 70 ). The authors concluded that the effect of the microbiome on obesity may not, in fact be mediated through its taxonomic composition but rather its function, because a discrepancy can exist between closely and distant-related taxa, at a functional level. It is also important to note that the gut microbiota is potentially variable in composition and function and modifiable, depending on diet at the time of analysis; therefore, transient changes in diet affect the gut microbiota; however, longer term effects (of dietary modulation on the gut microbiota) are largely unknown. A 10-d dietary intervention caused alteration in the superficial configuration of the microbiota but not in enterotypes( Reference Wu, Chen and Hoffmann 60 ), and short-term switch to solely plant or animal diets caused similar general trends in Bacteroidetes and Firmicute abundance( Reference David, Maurice and Carmody 71 ).
While the role of the intestinal microbiota in the aetiology of obesity has been elegantly demonstrated in animal models, human studies as yet have failed to concomitantly support the notion of significant phylum-level differences between obese and non-obese individuals and conclude that microbiota variation alone is not likely to cause obesity but overeating and disproportionate consumption of certain food items has the potential to alter the microbiota to one associated with increased energy harvest and reduced diversity. Although distinct taxonomic profiles have been established for lean and obese human in some populations, the relative contributions of the microbiota and diet to lean and obese phenotypes are most likely multi-faceted and regulated by a number of processes( Reference Turnbaugh, Hamady and Yatsunenko 72 , Reference Jumpertz, Le and Turnbaugh 73 ).
It is important to note that the comparison of microbial communities between seemingly disparate phenotype groups merely provides a descriptive platform and cannot, at this point, infer causality. Notwithstanding the primary influencing factors in relation to obesity (diet, lifestyle and genetics), human metagenomic biomarkers have shown much better discriminatory potential between lean and obese individuals compared to human genome markers, which only show modest variability( Reference Russo, Lauria and Siani 74 ). In fact, the specific metagenomic species have been identified which are associated with lower weight gain( Reference Le Chatelier, Nielsen and Qin 75 ). Furthermore, individuals with low bacterial gene counts are associated with reduced bacterial diversity, and increased inflammation and disease incidence (including type-2 diabetes and IBD)( Reference Cotillard, Kennedy and Kong 76 ). Therefore, low bacterial gene richness may determine the efficacy of dietary intervention in altering clinical phenotypes.
Gut microbiota and obesity/energy harvest
Evidence from animal models suggests that the microbiota is an important environmental factor effecting energy harvest and storage. The aetiology of microbiota-induced obesity was established by Backhed et al.( Reference Backhed, Manchester and Semenkovich 77 ) who found that inoculation of germ-free mice with conventional-raised mouse microbiota resulted in insulin resistance and increased body fat despite reduced food intake; this appeared to occur by promoting monosaccharide absorption and inducing de novo hepatic lipogenesis. Turnbaugh et al.( Reference Turnbaugh, Ridaura and Faith 78 ) confirmed this obesity-induced effect of transplantation of obese microbiota in germ-free animals and concluded that the microbiota is a significant contributing factor in the pathophysiology of obesity. However, a later study investigating the influence of different diets (low-fat, high-fat and Western diets) on body composition in germ-free and conventional mice concluded that the absence of gut microbiota does not provide a general protection from diet-induced obesity and that diet composition affects gut microbial composition to a larger extent than previously thought( Reference Fleissner, Huebel and Abd El-Bary 79 ).
Furthermore, evidence that diet plays a pivotal role in the maintenance of a lean-type microbiota has previously been established. Ridaur et al.( Reference Ridaura, Faith and Rey 80 ) showed that the microbiota of obese mice could be transformed to that of lean-counterparts by cohousing, resulting in phenotypic rescue. Transformation was primarily associated with invasion by members of the Bacteroides species; a decreased abundance of which has been associated with increased energy harvest in human subjects( Reference Jumpertz, Le and Turnbaugh 73 ) and obesity( Reference Turnbaugh, Hamady and Yatsunenko 72 , Reference Turnbaugh, Ley and Mahowald 81 , Reference Turnbaugh, Backhed and Fulton 82 ). However, only a diet low in saturated fat and high in fruit and vegetables was responsible for the maintenance of the lean phenotype, whereas those consuming a diet high in saturated fat and low in fruit and vegetables did not maintain the lean phenotype( Reference Ridaura, Faith and Rey 80 ). Fleissner et al.( Reference Fleissner, Huebel and Abd El-Bary 79 ) also demonstrated alteration in the faecal microbiota composition of conventional mice fed high-fat and Western diets, with the proportion of Firmicutes increasing at the expense of the Bacteroidetes with both diets. This infers a diet-dependent effect of successful microbiota transformation and establishes the transmissible and modifiable interactions between diet and intestinal microbiota and their influence on the host biology.
Gut microbiota, inflammation and adipogenesis
Low-grade, systemic inflammation, initiated by high-fat feeding has been shown to alter the gut microbiota. The first animal study to investigate the effect of high-fat feeding on systemic endotoxemia was conducted by Cani et al.( Reference Cani, Amar and Iglesias 83 ) and identified plasma lipopolysaccharide (LPS) as a major causative factor of systemic inflammation. In addition, this diet-induced increase in LPS appeared to increase the proportion of LPS-containing bacterial species in the gut. These findings suggest that the bacterial LPS production could trigger metabolic diseases characterised by insulin resistance and chronic low-grade inflammation, including obesity and diabetes.
Endotoxemia, namely activation of host-derived inflammatory mediators that induce systemic low-grade inflammation, occurs in obesity, the source and mechanism of which remains controversial. The gut microbiota has been proposed as a source of endotoxin or LPS which by virtue of being a major component of the Gram-negative bacterial cell wall could potentially influence intestinal permeability with changes in gut bacterial composition( Reference Pendyala, Walker and Holt 84 ). In addition, obese or diabetic subjects, or individuals consuming a high-fat diet, have double the circulating levels of serum LPS due to decreased alkaline phosphatase activity (an enzyme involved in intestinal LPS cleavage), increased chylomicron formation and decreased gut barrier integrity( Reference Delzenne, Neyrinck and Cani 85 ). Such changes are thought to activate the endocannabinoid system, which consists of bioactive lipids that bind to cannabinoid receptors and promote cell signalling (and are widely expressed in tissues that control the energy balance, i.e. pancreas, muscle, gut, adipose tissue, liver and hypothalamus)( Reference Matias and Di Marzo 86 ). This system is thought to regulate gut barrier function and energy homoeostasis( Reference Muccioli, Naslain and Backhed 87 ) and is particularly sensitive to dietary fat levels; dysregulation is thought to promote adipogenesis and obesity in pathological situations( Reference Pagotto, Marsicano and Cota 88 , Reference Bluher, Engeli and Kloting 89 ) and may be directly associated with specific changes in the composition of the gut microbiota( Reference Muccioli, Naslain and Backhed 87 , Reference Geurts, Lazarevic and Derrien 90 ).
Prebiotic interventions in older people
Functional foods such as probiotics and prebiotics are beneficial to the host by promoting health and preventing disease( Reference Vitali, Ndagijimana and Maccaferri 91 ). Probiotics are live microorganisms while prebiotics are non-digestible food ingredients which selectively stimulate the growth and/or activity of one or a limited number of bacteria in the colon( Reference Gibson and Roberfroid 92 ). Prebiotics, given their non-viable nature, offer some technological advantages and may be simpler to exploit than ‘living’ probiotics, allowing them to be readily incorporated into drinks, confectionary and a range of food products where they are also used to replace sugar, and improve texture and palatability( Reference Walton, Swann, Gibson, Rosenberg, DeLong, Lory, Stackebrandt and Thompson 93 ). Their inclusion can also improve the nutritional status of a product, e.g. the inclusion of inulin in the manufacturing of ice creams and spreads results in a reduced fat content in the finished product( Reference Gibson and Roberfroid 92 ). These factors coupled with their health benefits make prebiotics ideal components to incorporate into diets of target populations, from infants to the elderly.
There has been a significant increase in the annual publication rate of studies describing prebiotics and their potential to improve health over the last 20 years, investigating the effects on inflammation, immune system, infections, blood lipids and mineral absorption( Reference Lomax and Calder 94 , Reference Manning and Gibson 95 ). Many prebiotics have been investigated because of their ability to cause a bifidogenic effect in human subjects( Reference Davis, Martinez and Walter 96 ). Fermentation of prebiotics by some microorganisms that are considered beneficial, such as bifidobacteria and lactobacilli, indirectly leads to the production of SCFA by other gut bacteria; these SCFA have been shown to have both immunomodulatory and anti-inflammatory effects( Reference Macfarlane, Steed and Macfarlane 97 ).
Most prebiotic studies in human subjects have focused on oligosaccharides and inulin, and so the effects reported in elderly subjects are generally concerned with these compounds. Inulin supplementation in a study of constipated elderly subjects led to an increase in faecal Bifidobacterium proportions, and also resulted in a laxative effect when compared to lactose( Reference Kleessen, Sykura and Zunft 98 ). A study in France( Reference Bouhnik, Achour and Paineau 99 ) observed increased bifidobacteria and cholesterol excretion (in the stool) in an elderly population receiving fructo-oligosaccharide; baseline levels returned 4 weeks after the wash-out period. Another study with fructo-oligosaccharide ingestion in elderly nursing home patients confirmed its bifidogenic effect and also reported a decrease in IL-6 mRNA in peripheral blood monocytes, indicating its potential to influence the immune system( Reference Guigoz, Rochat and Perruisseau-Carrier 100 ). Effects in elderly subjects receiving a galacto-oligosaccharide mixture showed an increase in bifidobacteria levels, a decrease in pro-inflammatory cytokine levels (IL-1b, IL-6 and TNFα) and an increase in IL-10, an anti-inflammatory cytokine( Reference Vulevic, Drakoularakou and Yaqoob 101 ). Some studies have indicated that prebiotics such as fructo-oligosaccharide may also influence the antibody response in healthy elderly subjects vaccinated against influenza, but results are conflicting at present( Reference Lomax and Calder 94 ).
The potential positive effects of these prebiotics have also been demonstrated in particular study populations. For example, galacto-oligosaccharide has been shown to relieve symptoms of people suffering from irritable bowel syndrome,( Reference Silk, Davis and Vulevic 102 ) while also positively influencing the microbiota and immune function in overweight adults( Reference Vulevic, Juric and Tzortzis 103 ).
Dietary supplementation with wheat dextrin in critically ill jejunal-fed patients (including some elderly subjects) was shown to modulate the microbiota and increase SCFA even in the presence of antibiotics and other medications( Reference O'Keefe, Ou and Delany 104 ). Although further studies need to be carried out, this offers huge potential in reducing the risk of diarrhoea and counteracting the effects of antibiotics.
There are a number of other potential prebiotic compounds that have recently been studied in non-elderly subjects which may have potential in the older age group. Novel fibres such as polydextrose and soluble maize fibre can modulate the gut microbiota with increases in the populations of Faecalibacteria for both fibres, and with an increase in Lactobacilli in human subjects who consumed soluble maize fibre ( Reference Hooda, Boler and Serao 105 ). Animal studies have identified candidates for further investigation, e.g. arabinoxylan that was shown to increase proportions of Bifidobacteria, Bacteroides–Prevotella and Roseburia in obese mice on a high-fat diet, thus conferring both anti-obesity and health benefits( Reference Neyrinck, Possemiers and Druart 106 ). Resistant starch has been shown to modulate the microbiota in human subjects (including some elderly subjects)( Reference Walker, Ince and Duncan 107 ) and in pigs, with an increase in faecal SCFA levels also being observed in the latter( Reference Haenen, Zhang and Souza da Silva 108 ). Potential anti-cancer effects have been reported in a number of animal studies including rats with colorectal cancer which received a resistant starch type 3 (Novelose 330)( Reference Geier, Butler and Howarth 109 ). While trials using animal models are an acceptable first step towards identifying dietary candidates for further investigation, it must be acknowledged that this may not necessarily translate in a (young or old) human situation( Reference Walton, Swann, Gibson, Rosenberg, DeLong, Lory, Stackebrandt and Thompson 93 ).The above ingredients all require further investigation to confirm their beneficial effects and suitability in an elderly population. Furthermore, the extensive inter-individual variation in gut microbiota composition which exists between similar population sub-groups( Reference Frank, St Amand and Feldman 40 , Reference Finucane, Sharpton and Laurent 70 ), including the elderly( Reference Claesson, Cusack and O'Sullivan 9 , Reference Claesson, Jeffery and Conde 58 ) suggests that the response to prebiotic supplementation may be equally varied. The initial gut microbiota composition is evidently important as certain dominant bacterial groups are known to be more nutritionally versatile( Reference Ramirez-Farias, Slezak and Fuller 110 ) and individuals who harbour such bacteria have previously been reported as ‘non-responders’( Reference Davis, Martinez and Walter 96 , Reference Kleessen, Schwarz and Boehm 111 ). Therefore, studies to establish whether a reduced diversity microbiota can be rescued by dietary intervention and thus impact health are warranted. In addition, because individual prebiotics promote various specific individual bacterial species, the effect of combined prebiotic intervention on ‘health-promoting’ plyla are required. Furthermore, the timeframe required to modulate key health-related bacterial species, at an individual level, is unknown and unlikely to be uniform.
Knowledge gaps/challenges for dietary modulation of the microbiota in older people
Although several studies (( Reference Mueller, Saunier and Hanisch 30 , Reference Claesson, Jeffery and Conde 58 )) have examined the gut microbiota in elderly populations, defining it is still a challenge given the difficulty in comparing individual studies, country effect, inter-individuality and the variation in age of which the elderly study population is set( Reference Biagi, Candela and Fairweather-Tait 112 ). The influence of ageing on bacterial populations, e.g. Bacteroides is difficult to determine given the effect of other factors such as antibiotics treatment and hospitalisation which need to be considered( Reference Bartosch, Fite and Macfarlane 113 ). In fact, disruption of the gut microbiota with oral antibiotics often precedes the emergence of several enteric pathogens. Recent evidence suggests that gut mucosal carbohydrates (e.g. fructose and sialic acid) can be released and catabolised by the resident microbiota in the presence of antibiotic-associated pathogens (including Salmonella and Clostridium difficile) and can influence their competitiveness and expansion within the gut( Reference Ng, Ferreyra and Higginbottom 114 ). These findings may have implications for designing new therapies for the prevention of antibiotic-associated pathogenic infection.
Understanding the relationship between ageing and gut microbiota will require longitudinal, multicentre studies with larger populations using standardised procedures and methods of analysis to allow direct comparison, eliminate discrepancies or identify true variation. This is also the case for identifying future nutritional products which can promote healthy ageing. While a number of studies examining the effect of dietary interventions have been carried out in human subjects (with a limited number in elderly subjects), interpreting the outcome is difficult for a number of reasons including: use of a small study population, lack of a placebo, duration of the intervention and study design( Reference Simren, Barbara and Flint 115 ). The dose-effect and establishing a minimum effective dose is difficult when both variation between studies and dose rates exist( Reference Roberfroid, Gibson and Hoyles 116 ). Inter-individual variability of a response can also occur, e.g. increased Bifidobacterium abundance and decreased bacteroides abundance were not observed following a galacto-oligosaccharide treatment which included elderly subjects( Reference Walton, van den Heuvel and Kosters 117 ). The choice of a particular form of prebiotic could be crucial in certain instances if it is to be effective, e.g. the chain length of arabinoxylan determines the intestinal site of fermentation, and thus the influence on bacterial populations and resulting SCFA levels is modulated( Reference Sanchez, Marzorati and Grootaert 118 ). Resistant starch types 2 and 4 have different effects on microbiota composition because of their accessibility to the bacteria due to their chemical structures( Reference Martinez, Kim and Duffy 119 ). In addition, nutrient stores available to the gut microbiota that may influence the effects of dietary interventions designed to modulate the gut microbial profile warrant further investigation.
As prebiotics are classed as food substances rather than medications by the Food and Drugs Authority (USA), the requirements for manufacturers to conduct large-scale, placebo-controlled trials is small compared to clinical trials of a medicinal product where hundreds of subjects are recruited at a huge cost. However, it is important to note that if a functional claim is to be made for supposedly beneficial prebiotic foods/ingredients, they must demonstrate a positive health effect and not just alter the microbiota composition to be considered therapeutic( Reference Salminen and van Loveren 120 ). To date the majority of research on functional foods has mainly focused on the probiotics Bifidobacteria and Lactobacilli ( Reference Toward, Montandon and Walton 121 ) and prebiotics oligosaccharides and inulin( Reference Laparra and Sanz 122 ). However, considering our increasing knowledge of the gut microbiota (and the range of species present) other potential contenders such as F. prausnitzii (e.g. as a treatment for Crohn's disease( Reference Sokol, Pigneur and Watterlot 42 )) are emerging. The development of these functional food ingredients is a personalised healthcare strategy where the effectiveness of the product is consumer dependent, e.g. a prebiotic that promotes a particular bacterium is useless unless the organism is present in the host. It is also important for elderly and unwell populations in particular that frailty, diseases and medications are considered to prevent any adverse effects, highlighting the need for well designed, intervention trials.
Conclusions
While research indicates reduced intestinal microbiota diversity particularly with advancing years and with reduced dietary diversity, it is important that future research should focus not only on establishing the core gut microbiota in elderly subjects, but also on the impact of long-term dietary intervention on microbiota modification. While we await clarification from intervention studies as to whether a reduced diversity microbiota can be rescued and modified such that it can promote and support better health outcomes in elderly populations, further studies on the elderly gut metagenomic capacity and function are required to identify important bacterial species to target for health promotion. Our expanding knowledge of gut microbiota, its influence on health and ageing is of global importance given that 22 % (2 billion) of the population will be aged 60 years and over by 2050 according to the WHO. The development of nutritional products (e.g. prebiotics) which can improve overall health through influencing the gut microbiota and its effects such as disease prevention and improved immune function will lead to a reduction in hospitalisation, drug intake and financial savings in the healthcare sector. This is an exciting and challenging time for all involved; however, as research continues to examine the interaction between dietary ingredients, the microbiota and influence on health, the key elements leading to the successful development of such nutritional products lie in comprehensive research which is soundly designed and executed thereby removing any ambiguity in the interpretation of results.
Acknowledgement
We thank Dr Denise Lynch for preparing Figure 1.
Financial Support
Work in P. W. O'T.'s laboratory was supported by Science Foundation Ireland through a Principal Investigator award and a CSET award to the Alimentary Pharmabiotic Centre, and by an FHRI award to the ELDERMET project by the Department of Agriculture, Fisheries and Marine of the Government of Ireland. The work in E. M. O'C.'s laboratory was supported by the Allen Foundation Inc., Michigan, USA.
Conflicts of Interest
None.
Authorship
All authors contributed to the planning, scoping and writing of this review.