Introduction
The increasing world population, along with a higher income, especially in developing countries, is predicted to increase the demand for animal protein. This increased demand and the limits to arable land increase the demand for feed ingredients and their price. As feed represents approximately 50 % of the total production costs in animal farming activities( Reference Seibert and Norwood 1 ), a rise in ingredient price requires a higher efficiency in nutrient utilisation in order to be able to produce animal protein without increasing production costs.
Protein deposition in single-stomached production animals is directly linked to the digestibility of protein in the feed and the adequacy of the amino acid profile to match the animal’s requirements( Reference Conde-Aguilera, Cobo-Ortega and Mercier 2 ). This is evident from the vast amount of research conducted over the last decades to define the nutritional requirements of production animals (to maximise protein deposition and to minimise protein oxidation) and to evaluate the nutritional quality of the ingredients or feeds that are fed to meet these requirements( Reference Conde-Aguilera, Cobo-Ortega and Mercier 2 – Reference Petersen, Liu and Stein 7 ).
Large variation exists in the nutritional quality of protein in feed ingredients, especially in those that received a thermal processing step, such as the most commonly used oilseed co-products (for example, soyabean (Glycine max) meal or rapeseed (Brassica spp.) meal)( Reference Grieshop, Kadzere and Clapper 8 , Reference Messerschmidt, Eklund and Sauer 9 ). Heat used during production of ingredients facilitates the separation of the oil fraction from the full-fat oilseed, inactivates antinutritional factors present, and removes the residual organic solvents used for oil extraction( Reference Classen, Newkirk and Maenz 10 , Reference Paraíso, Cauneto and Zemp 11 ). However, depending on the severity of the conditions employed, thermal processing may negatively affect the digestibility and nutritional value of proteins( Reference Newkirk, Classen and Edney 12 ). As many of the ingredients used for compound feed production have already undergone a processing step, compound feed production is described here as secondary processing. Secondary processing can alter the protein quality of native as well as previously heat-processed protein sources( Reference Almeida, Htoo and Thomson 3 , Reference Stein and Bohlke 13 ). Compound feeds are processed in order to control the physical properties of ingredients and improve nutrient availability, which leads to improvements in performance, and to the reduction of the pathogenic burden in feed( Reference Hancock and Behnke 14 ). It is usual yet unintentional, however, that during processing of ingredients and feed compounding, the factory throughput of material and the physical quality of the feed are of prime importance over the nutritional consequences of the processing parameters employed( Reference Abdollahi, Ravindran and Svihus 15 ). These processes could potentially decrease protein digestibility and amino acid bioavailability.
Most nutritional studies conducted on feed or feed ingredient processing focus on digestibility of nutrients as an end parameter, and fail to identify the underlying mechanisms for the differences found( Reference Stein and Bohlke 13 , Reference Lundblad, Hancock and Behnke 16 – Reference Seneviratne, Beltranena and Newkirk 18 ). For example, extrusion of field peas (Pisum sativum) at 75, 115 and 155°C increased the standardised ileal digestibility of crude protein from 81 % in untreated field peas to 89, 94 and 92 % for the extrusion treatments, respectively( Reference Stein and Bohlke 13 ). These authors suggested that denaturation of either storage proteins or antinutritional factors explain the observed effects, but did not measure this. Furthermore, processing conditions in many studies are poorly described and when complex equipment (for example, pelleting or extrusion) was used, simultaneously involving several processing parameters, such as heat, pressure and shear, it is impossible to disentangle their effects( Reference Chae, Han and Kim 19 – Reference L’Anson, Choct and Brooks 21 ). It is important to understand the effects of each processing parameter individually, as well as interdependency of parameters, on the structural and chemical changes of the protein fraction, which could influence its nutritional value.
Processing, as described in the present review, is defined as any action that results in physical or chemical changes or disrupts the conformation of a native or previously processed ingredient or mixture of ingredients for compound feed production. Here, we address the effects of processing on the physico-chemical changes of proteins and the consequences of these changes for the nutritional quality of protein, linked to the end point of protein digestion. Selection of literature was limited to vegetable ingredients commonly used in swine diets, which reported effects of processing on both protein structure and digestibility. The review starts with a brief summary of the biochemistry of native proteins and its relationship with crude protein digestibility. Although subject to discussion, a protein with a structural conformation equal to its presence in the original source can be considered as native. We continue with an overview of the available literature on the effects of processing at various conditions on protein structure of ingredients used in swine feeds, and the consequences of these changes for protein digestibility in single-stomached production animals. When possible, correlations between studies are evaluated with the CORR procedure of Statistical Analysis System software version 9.3 (SAS Institute, Inc.)( 22 ) using the information also included in the tables and online Supplementary tables. The correlations were used to identify trends on the mechanisms by which protein structure and structural modifications due to processing influence digestibility.
There are shortcomings to the different digestibility techniques reported in literature which might confound the correlations between structural properties of proteins and digestibility. For example, the effects of gut microbiota, antinutritional factors and dietary fibre on digestion are difficult to simulate using in vitro methods( Reference Moughan 23 ). In addition, the standardised ileal digestibility technique does not take into account the specific endogenous losses originating from antinutritional factors and dietary fibre. Finally, the measurement of faecal protein digestibility includes the fermentation of proteins in the large intestine, which might overestimate protein digestibility at the level of the ileum. For this reason, correlations between structural properties and digestibility were performed for studies in which digestibility was analysed using similar techniques (for example, in vitro or faecal).
Biochemistry and bioavailability of protein in vegetable feed ingredients
Structure of native proteins
Proteins are polymers made up of different amino acids linked by peptide bonds. This linear chain of amino acids forms the primary structure of the protein and their sequence, specific for every protein, also determines the secondary, tertiary and quaternary structures of the native form of the protein( Reference Davis and Williams 24 ). The main configurations of the secondary structure of protein are the α-helices and β-sheets( Reference Guan, Marcos and O’Connell 25 , Reference Berman, Westbrook and Feng 26 ) (Fig. 1), although others exist, mostly resulting from variations of these configurations. The α-helices and β-sheets provide strength and rigidity to proteins( Reference Gropper, Smith and Groff 27 ). Regions in the primary structure of a protein that lack structural elements are considered as random coils( Reference Smith, Fiebig and Schwalbe 28 ), which by definition are less stable( Reference Gropper, Smith and Groff 27 ). The secondary structure of proteins can be determined by several techniques, such as Fourier transform IR (FTIR) spectroscopy and circular dichroism, the latter one used solely for soluble proteins. Circular dichroism uses the polarisation angle of polarised light in the far-UV region for the determination of the secondary structure of proteins and the near-UV region for the determination of changes in the tertiary structure. With FTIR spectroscopy, the amide I region (1600–1700/cm) is used to measure the secondary structure of proteins by detecting the vibrations of the carboxyl groups of amino acids( Reference Hu, Kaplan and Cebe 29 , Reference Carbonaro, Maselli and Nucara 30 ). This region of the spectra includes bands for the main secondary structures: α-helices (1650–1660/cm) and β-sheets (1630–1638/cm). Within the amide I region, also bands that indicate modifications to the native structural conformation can be distinguished, such as the intermolecular β-sheets (1620–1630/cm) and A2 bands (1690–1695/cm), indicative for protein aggregation( Reference Carbonaro, Maselli and Nucara 30 ). Intermolecular β-sheet structures originate from intermolecular hydrogen bonds( Reference Shivu, Seshadri and Li 31 ) and A2 regions from intermolecular aggregation of proteins or the vibration of the carboxyl groups of the amino acid side chains( Reference Carbonaro, Maselli and Nucara 30 ).

Fig. 1 Cartoon view of the main secondary structures of proteins. The protein in the cartoon is Protein Data Bank ID: 4R80( Reference Guan, Marcos and O’Connell 25 , Reference Berman, Westbrook and Feng 26 ).
Intramolecular formation of non-covalent and disulfide bonds between side chains of amino acids holds the three-dimensional tertiary structure of protein together. The hydrophobic amino acids are hidden in the interior of the structure. Non-covalent bonds between amino acids can be due to Van der Waals interactions between hydrophobic amino acids (for example, proline, tryptophan), to hydrogen bonding, or to electrostatic interactions between amino acids with opposite charges (for example, lysine and glutamate)( Reference Gropper, Smith and Groff 27 ). Moreover, proteins in their tertiary structure can interact with each other and form non-covalent and covalent (disulfide) intermolecular bonds, which give rise to the quaternary structure of proteins. Most of the storage proteins in vegetable sources are present in a quaternary conformation, which can also be considered their native conformation. Examples of such proteins are napin (albumin) and cruciferin (globulin) in rapeseed, and the globulins β-conglycinin and glycinin in soyabean. Rupture of intermolecular bonds releases the individual proteins that compose the quaternary structure. For example, under reducing conditions, the acidic and basic polypeptides of soyabean glycinin and rapeseed cruciferin are separated as seen by electrophoresis (SDS-PAGE)( Reference Inquello, Raymond and Azanza 32 , Reference Hou and Chang 33 ). The energy required to break disulfide covalent bonds is higher than the energy required to break non-covalent bonds (Table 1). Proteins with large numbers of disulfide bonds are regarded to have a higher resistance to enzymic activity during digestion and also a lower sensitivity to structural changes during processing than proteins with small numbers of disulfide bonds( Reference Clemente, Vioque and Sánchez-Vioque 34 ).
Table 1 Types, strength and amino acids involved in bonds found in proteins and solvents that can dissolve these bondsFootnote *

CHAPS, 3-[(3-cholamidopropyl)dimethylammonio]-1-propanesulfonate; DTT, dithiothreitol.
* Modified after Liu & Hsieh( Reference Liu and Hsieh 81 ).
Proteins from cereals and legumes are usually stored as protein bodies. In general, approximately 80 % of the protein content of legumes can be classified as storage proteins and is stored in protein bodies( Reference Sikorski 35 ). It has been defined that any protein present at least in 5 % of the total protein content can be considered as storage protein( Reference Derbyshire, Wright and Boulter 36 ). The remainder of the proteins, which comprise the proteins with biological activity (for example, enzymes and inhibitors), are not contained within protein bodies.
An overview of the structural properties of the major proteins that are present in important vegetable ingredients used for commercial swine compound feed production is shown in Table 2. The proteins are described based on the most abundant protein types for each ingredient. For example, although classified under the same name (i.e. zein), the prolamins from maize consist of four types of proteins with different secondary structures (i.e. α-, β-, γ- and δ-zein). The secondary structure of α-zein is mostly α-helical, whilst that of β-zein (which is also related to γ-zein) consists mostly of β-sheets and turns( Reference Kim, Woo and Clore 37 ). The difference in the secondary structure of these proteins originates from their different amino acid compositions( Reference Shewry and Tatham 38 ).
Table 2 Biochemical characteristics reported for major proteins of vegetable ingredients frequently used in pig feed

MM, molecular mass; pI, isoelectric point.
* Values were determined from in vitro measurements.
Overall, globulins are the predominant protein types in oil (for example, rapeseed) and legume seeds (for example, soyabean), whilst prolamins and glutelins are the most abundant cereal proteins. In addition, proteins with very different structural characteristics can be found within the same ingredient. For example, cruciferin in rapeseed has a higher molecular weight and α-helix:β-sheet ratio than napin. According to the ‘Osborne’( Reference Osborne 39 ) classification of proteins, albumins are soluble in water, globulins in diluted salt solutions, prolamins in ethanol–water solutions and glutelins in diluted alkali solutions. Although many proteins are classified as being from the same ‘Osborne’ type( Reference Osborne 39 ), proteins from different ingredients usually differ in their amino acid composition. This is reflected in different conformations, thereby making it impossible to generalise on the types of bonds present and their secondary, tertiary or quaternary structures. Globulins and albumins seem to have a higher content of intramolecular β-sheet structures compared with prolamins. This is also reflected in a lower α-helix:β-sheet ratio of the globulins and albumins compared with the prolamins.
Carbonaro et al. ( Reference Carbonaro, Maselli and Nucara 30 ) suggested that the secondary structure of the protein in a (processed) ingredient is a good predictor of digestibility. These authors reported that the proportion of intramolecular β-sheet structures in food sources from vegetable and animal origin is negatively correlated with in vitro crude protein digestibility (r –0·98) using porcine trypsin and peptidase, bovine chymotrypsin and a bacterial protease (the latter added in a subsequent incubation). A higher α-helix:β-sheet ratio has been described to negatively influence in vitro intestinal digestibility of rumen undegraded protein in ruminants( Reference Yu and Nuez-Ortin 40 ), although the mechanism of digestion in ruminants differs from that in single-stomached animals. The relationship between the α-helix:β-sheet ratio and digestibility was also studied in vitro ( Reference Rubio, Pérez and Ruiz 41 ). It was found that the in vitro digestibility of the albumin, globulin and vicilin fractions of peas (41, 63 and 88 %, respectively), using porcine pepsin and pancreatin, corresponded with the α-helix:β-sheet ratio of 0·06, 0·20 and 0·80, respectively. Variation in the secondary structure of proteins, however, does not seem to explain the variation in digestibility completely. Napin and cruciferin, which have very different secondary structures (for example, ratio α-helix:β-sheet is 0·66 in napin and 0·35 in cruciferin), do not differ much in their apparent faecal digestibility in rats( Reference Delisle, Amiot and Goulet 42 ). Also, proteins with similar secondary structures, such as glycinin and β-conglycinin from soyabeans, differ in their apparent faecal digestion coefficients( Reference Delisle, Amiot and Goulet 42 ). The larger number of disulfide bonds in glycinin compared with β-conglycinin has been postulated to provide a further explanation for the differences in the digestibility values between these proteins( Reference Hou and Chang 33 , Reference Hou and Chang 43 ).
There was no correlation (P>0·05) between the secondary structure of isolated native proteins and in vitro or faecal digestibility (Table 2). However, literature on food allergens( Reference Bannon, Goodman and Leach 44 , Reference Bannon, Fu and Kimber 45 ) suggests that the native structure of some proteins (for example, Ara h 1 from groundnuts or β-conglycinin from soyabeans) influences the accessibility of gastric proteases, which might also limit the access of intestinal peptidases for proteolysis. For example, the main globulin from kidney beans (Phaseolus vulgaris), phaseolin, has high resistance to pepsin and pancreatin hydrolysis (19 % in vitro digestibility), which was attributed to its closed tertiary or quaternary structure that restricts enzyme accessibility( Reference Montoya, Leterme and Victoria 46 ).
Structural changes due to protein denaturation
Exposure of plant storage proteins to harsh conditions, like extreme pH values, heat or pressure, causes protein denaturation. For example, a pH higher than 11·5 or lower than 3·0 or pressure at 400 MPa for 10 min cause complete denaturation of soya glycinin( Reference Kim, Kim and Yang 47 , Reference Zhang, Li and Tatsumi 48 ). Denaturation is the unfolding of proteins from their tertiary or secondary structures and will occur when the denaturing influence is sufficiently large to break the non-covalent or covalent bonds that hold the structure together. Conditions for denaturation depend on the type and structure of each native protein( Reference Kilara and Sharkasi 49 ). Refolded proteins with a structural conformation different from their native state can also be considered as denatured. Proteins refold in an attempt to minimise their free energy state, with simultaneous formation of new bonds or interactions( Reference Becker and Yu 50 ). It has been suggested( Reference Marsman, Gruppen and Mul 51 ) that the new bonds formed are not representative of the original conformation of the protein, as this is statistically unlikely to occur. These new bonds result from intramolecular electrostatic and hydrophobic interactions between amino acids, or from intermolecular non-covalent and/or disulfide bonds between two unfolded proteins. The formation of these bonds or interactions between amino acids may lead to protein aggregates, which can be soluble or insoluble depending on the molecular weight of the aggregate. As defined by Wang et al. ( Reference Wang, Nema and Teagarden 52 ), protein aggregates consist of proteins that have lost their native state and are at least twice the size of the native protein. The mechanisms that explain the aggregation of proteins were extensively reviewed by these authors. Protein aggregation can also occur due to crosslinking reactions between proteins, resulting from protein oxidation and the formation of covalent bonds between amino acids, which is favoured at alkaline pH( Reference Schwarzenbolz and Henle 53 ). An example of the latter is the formation of lysinoalanine, which is a crosslink between lysine and alanine and is mediated through the formation of dehydroalanine from β-substituted amino acids (for example, phosphoserine) and the subsequent nucleophilic addition of the ε-amino group of lysine( Reference Schwarzenbolz and Henle 53 ).
During digestion, proteins are partially denatured by the acidic pH conditions in the stomach, which facilitates the accessibility of proteases to the peptide bonds. Pepsin is secreted in the stomach and cleaves peptide bonds between hydrophobic or neutral amino acids, except for proline. Proteins that are resistant to the acidic and proteolytic conditions in the stomach can reach the intestinal mucosa and cause allergic reactions( Reference Bannon, Goodman and Leach 44 ). Trypsin and chymotrypsin are secreted in the pancreatic juices in the duodenum. Trypsin is highly specific to lysine and arginine, whilst chymotrypsin has a preference for large hydrophobic amino acids, such as tryptophan, tyrosine and phenylalanine. This specificity becomes important when the cleavage sites for these enzymes are physically or chemically blocked by structural constrains, such as protein aggregation due to non-covalent or covalent bonds formation or by modification of the amino acid residues due to protein oxidation (for example, dityrosine bonds) or Maillard-type reactions.
Changes in the structure of proteins due to denaturing treatments, such as heat, pH and pressure, can lead to proteins that become either more susceptible or more resistant to proteolysis. Protein denaturation can result in the formation of random coils, which exposes groups that are not usually accessible in the native form, thus becoming more susceptible to enzymic hydrolysis( Reference Carbonaro, Maselli and Nucara 30 , Reference Meade, Reid and Gerrard 54 ). The formation of random coils due to processing results from the breakdown of the tertiary structure and that of the secondary structure, such as α-helices and intramolecular β-sheets. Denaturation, especially at extreme conditions involving high temperatures or pH extremes, promotes protein aggregation( Reference Carbonaro, Maselli and Nucara 30 , Reference Carbonaro, Cappelloni and Nicoli 55 – Reference Tang, Chen and Ma 59 ) and crosslinking between amino acids( Reference Gerrard 60 ). Aggregation and crosslinking reactions could decrease the accessibility of digestive enzymes, thereby reducing protein digestibility( Reference Pinto, Léonil and Henry 61 , Reference Rérat, Calmes and Vaissade 62 ).
The extent of structural changes in proteins depends on the conditions employed during processing, and also on the types of proteins present. Upon thermal processing of rapeseed protein isolates, the globulin fraction (cruciferin) was more affected and formed a larger amount of aggregates than the albumin fraction (napin)( Reference He, He and Chao 63 ). Similar results have been reported for soyabean proteins, in which β-conglycinin is more heat-sensitive than glycinin due to the disulfide bonds present in the latter protein type( Reference Marsman, Gruppen and Mul 51 ). Disulfide bonds can be broken during processing, but depending on the severity of the conditions, the free thiol residues can also react to form new bonds via sulfydryl-disulfide interchange reactions and increase aggregation( Reference Tang, Chen and Ma 59 ). Differences in amino acid composition and structure between different types of the same protein (for example, α-, β- and γ-kafirins) might also influence the response of the proteins to denaturing conditions and digestion( Reference Oria, Hamaker and Shull 64 ).
Furthermore, it has been suggested that the net charge of the protein influences the structural response to thermal processing( Reference Tang, Chen and Ma 59 ). Increasing NaCl concentrations from 0 to 1·5 m at pH 8 increases the denaturation temperature of soya glycinin from 79·1 to 98·5°C( Reference Kim, Kim and Yang 47 ). This is an indication of a protective effect of the salt on the native structure of glycinin from protein denaturation at increasing temperatures. When similar processing conditions were used, a higher content of hydrophobic and uncharged polar amino acids and higher acidic:basic amino acid ratio were linked to a decrease in aggregation( Reference Tang, Chen and Ma 59 ). It has been proposed( Reference Carbonaro, Cappelloni and Nicoli 55 ) that heat-induced aggregation promoted by basic amino acids (lysine, arginine or histidine) could have detrimental effects on protein digestibility.
In addition, proteins are highly susceptible to react with reducing sugars upon heating to produce Maillard reaction products. Some essential amino acid residues, such as lysine and arginine, are more susceptible than other amino acids to these type of reactions. Lysine susceptibility is due to the presence of the additional amino group, whilst in arginine it is due to the additional guanidinium group( Reference van Rooijen, Bosch and van der Poel 65 ). The nature of these reactions and the wide range of compounds formed have been reviewed elsewhere( Reference van Rooijen, Bosch and van der Poel 65 ). It must be considered that structural changes per se (for example, protein aggregation) and chemical changes due to Maillard reactions may occur simultaneously( Reference Gerrard, Lasse and Cottam 66 ) and that the effects of both on proteolysis might be confounded.
Protein digestion is a multifactorial process that is influenced not only by the physico-chemical properties of the proteins, but also by the matrix in which the protein is embedded. Microstructural components of the feed are not often described in the literature and this aspect should be taken into account in future research that links modifications of proteins due to processing to protein digestibility.
Processing, protein structure and protein digestibility
Extrusion and pelleting, which are frequently used agglomeration processes in feed production, involve the simultaneous utilisation of heat, moisture, shear and pressure. These agglomerating technologies can be considered as complex processes, in which process parameters (for example, temperature, moisture, screw configuration) and system parameters (for example, mechanical energy, throughput, residence time) are interrelated and can influence each other( Reference Draganovic, van der Goot and Boom 67 – Reference Meng, Threinen and Hansen 69 ). Both process and system parameters influence the final structural conformation of proteins and enzyme accessibility for proteolysis, and consequently affect protein digestion. Hence, it would be important for further research efforts to disentangle the effects of individual processing factors on protein, which will allow maximisation of dietary protein utilisation in animal production.
Heat-induced changes
Thermal treatments are involved in most common processing technologies to achieve the desired physical state of the ingredients and ingredient mixtures. Other reasons are to improve the digestibility of starch and proteins, to eliminate residual organic solvents after oil extraction and antinutritional factors, and to maintain hygienic levels of the processed feeds( Reference Abdollahi, Ravindran and Svihus 15 , Reference Abdollahi, Ravindran and Wester 70 – Reference Van der Poel 72 ).
The maximum temperature achieved during commercial oil extraction of soyabeans and rapeseeds ranges between 100 and 110°C( Reference Classen, Newkirk and Maenz 10 , Reference Paraíso, Cauneto and Zemp 11 , Reference Newkirk and Classen 73 , Reference Pope, Karr-Lilienthal and Utterback 74 ), a temperature which is held constant for 60–90 min( Reference Newkirk, Classen and Edney 12 ). It must be emphasised that these temperatures are achieved by toasting, which comprises the use of steam, which is a source of heat that involves large amounts of moisture. Large differences with respect to the formation of Maillard reaction products in soyabean meal proteins have been detected between dry (for example, oven-drying) and wet (for example, autoclaving) sources of heat( Reference Gonzalez-Vega, Kim and Htoo 75 ), making it difficult to disentangle the individual effects of heat and moisture.
The temperature applied during compound feed production (secondary processing) depends on the technology employed. Common pelleting temperatures for swine feed production range between 60 and 100°C, whilst higher temperatures are achieved with expander processing (90–130°C) and extrusion (60–160°C)( Reference Chae and Han 76 ).
Changes in the secondary structural conformation of proteins due to thermal processing have been reported (Table 3), although only a limited number of studies have linked these changes to protein digestibility. After autoclaving at 120°C for 20 min, an increase in the relative amount of random coil conformation in different whole ingredients was reported( Reference Carbonaro, Maselli and Nucara 30 ). Heating at 100°C for 15 min also increased the proportion of random coils in rapeseed protein isolate from 23 % in the unheated material to 28 % after heating, whilst increasing simultaneously the proportion of β-sheets from 10 to 33 %( Reference He, He and Chao 63 ). Formation of random coils is an indication of successful protein denaturation, also linked to a positive correlation with in vitro digestibility (r 0·91)( Reference Carbonaro, Maselli and Nucara 30 ). The increase of the in vitro digestibility reported in this study after processing ranges from 2 to 6 %, which can be considered as low. In addition, inactivation of protease inhibitors was not considered in this study. The effect of the formation of random coils on the increase in digestibility after the thermal treatment might be confounded with the inactivation of protease inhibitors. These authors also reported that autoclaving induced the disappearance of the intramolecular β-sheet structures and the appearance of intermolecular β-sheet structures and A2 bands in FTIR spectra. These two bands in the spectra (intermolecular β-sheets and A2 bands) have been related to protein aggregation and the proportion of intermolecular β-sheets was highly predictive (r –0·99) for in vitro protein digestibility. As formation of aggregates and random coils occur simultaneously during processing, the net effect on digestibility is related to the relative frequency of each phenomenon. When taken together (Fig. 2), the total content of intramolecular β-sheet structures from native proteins and the intermolecular β-sheet structures from proteins after thermal treatment has a high negative correlation (r –0·95; P<0·001; n 8) with in vitro crude protein digestibility. The total content of intramolecular and intermolecular β-sheets can be used as a fast predictor for protein digestibility, at least for legume protein sources. As both β-sheet structures within the protein and between proteins correlate well with in vitro crude protein digestibility, it appears that the structure itself (i.e. β-sheets), but not its location, limits proteolysis. The correlation between the α-helix:β-sheet ratio and in vitro crude protein digestibility was not significant, but exhibits a trend (r 0·65; P=0·08). Nevertheless, native legume ingredients tend to have a lower ratio and digestibility compared with thermally treated ingredients. It should be noted, however, that these correlations originate from a single study( Reference Carbonaro, Maselli and Nucara 30 ) and that the ingredients used probably contained protease inhibitors. It is likely that these protease inhibitors were inactivated during thermal treatment and that the increase in digestibility can be partly attributed to the inactivation of protease inhibitors and partly to the modifications of the secondary structure.

Fig. 2 Correlation between the β-sheets content and in vitro crude protein digestibility coefficient of native (- - -) and thermally treated (––) whole legume seeds: ○, Phaseolus vulgaris; ●, Cicer arietinum; ∆, Lens culinaris; ▲, Glycine max. Adapted from Carbonaro et al. ( Reference Carbonaro, Maselli and Nucara 30 ).
Table 3 Relative spectral weights of the secondary structure of proteins and in vitro crude protein digestibility coefficient after processing under different conditions

Although the in vitro digestibility of the most frequent protein fraction present in kidney beans (phaseolin) was improved after thermal processing (Table 4), the digestibility of other protein fractions from the same source (total albumins, protease inhibitor and lectins) was largely reduced( Reference Genovese and Lajolo 77 ). This could explain why minor or no changes in digestibility were reported after thermal treatment of whole kidney beans or protein isolates thereof ( Reference Carbonaro, Maselli and Nucara 30 , Reference Carbonaro, Cappelloni and Nicoli 55 , Reference Tang, Chen and Ma 59 ). The overall effect on total protein in the feedstuff seems to be a combination of the positive and negative effects on each individual protein fraction. This means that for a complete understanding of the underlying mechanisms that explain the effects of processing on protein digestibility in feed ingredients, the effects on each individual protein type needs to be studied. Positive effects of the thermal treatment of kidney beans on apparent ileal crude protein digestibility in pigs have been reported( Reference Van der Poel, Blonk and Huisman 78 ). It was discussed that these effects might be due to inactivation of antinutritional factors and conformational changes of proteins during ‘high temperature short time’ treatments (136°C; 1·5 min), which is in contrast to the lower temperature and longer times (ranging from 95 to 121°C; 15 to 30 min) applied in other studies( Reference Carbonaro, Maselli and Nucara 30 , Reference Carbonaro, Cappelloni and Nicoli 55 , Reference Tang, Chen and Ma 59 , Reference Genovese and Lajolo 77 ).
Table 4 Change in in vitro and in vivo protein digestibility compared with a control of feed ingredients as affected by various processing treatments

* Change (%) within studies with respect to control.
† Data extrapolated from graphs.
‡ Standardised ileal digestibility, rats.
§ Faecal digestibility, mink.
Changes in the solubility of proteins, due to the heat-induced formation of insoluble aggregates, have been reported for a wide range of experimental conditions and were linked to changes in the types of bonds within and between proteins (see online Supplementary Table S1). There is a parallel increase in the amount of insoluble protein aggregates with the severity of the thermal treatments applied. Already at 80°C, 31 % of the protein content in rapeseed protein isolate formed insoluble aggregates, compared with 0 % in the unheated material( Reference He, He and Chao 63 ). In the isolate, cruciferin (globulin) was more susceptible to aggregate formation than napin (albumin)( Reference He, He and Chao 63 ). Most studies agree on a decreased solubility of a protein after a thermal treatment, compared with that of the native protein( Reference Li and Lee 57 , Reference Lin, Huff and Hsieh 79 – Reference Liu and Hsieh 81 ). The reduction in protein solubility is an indication of the formation of insoluble protein aggregates. Many native proteins are soluble as their hydrophobic groups are located on the inside of the molecule. After denaturation, involving reorientation of the hydrophobic groups and refolding, the initial protein aggregates formed are soluble, but they become insoluble when their size exceeds the solubility limit( Reference Shivu, Seshadri and Li 31 ).
Protein solubility studies with agents capable of cleaving different types of bonds (for example, urea, SDS and dithiothreitol (DTT)) have been used in order to study the main causes for protein insolubility (see online Supplementary Table S1). Urea and SDS are good agents to cleave non-covalent bonds, whilst DTT is adequate for the reduction of disulfide bonds into thiols. The solubility of unheated proteins in buffer solutions is similar to the solubility of aggregated proteins with solutions that contain both non-covalent and covalent cleaving agents. Combining these two types of cleaving agents has a synergistic effect on the amount of solubilised protein, compared with the amount of protein solubilised by using them separately( Reference Li and Lee 57 , Reference Lin, Huff and Hsieh 79 – Reference Liu and Hsieh 81 ). This indicates that non-covalent and covalent bonds are mutually important in maintaining the structure of aggregated proteins.
After thermal processing, there is an increase in the disulfide bond content with a simultaneous reduction in the content of thiols (Table 5). For example, thiol content was reduced from 6·6 μmol/g protein in the albumin and the protein inhibitors plus lectins fractions isolated from native kidney beans to 0·2 and 0·6 μmol/g protein, respectively, after heating at 121°C for 15 min( Reference Genovese and Lajolo 77 ). At the same time, the disulfide bond content was increased from 24·8 to 28 μmol/g protein in the albumin fraction and from 22·5 to 25·5 μmol/g protein in the protein inhibitors plus lectins fraction. These changes were more evident with autoclaving at 121°C for 15 min than with cooking at 99°C for 30 min. This could be an indication that the effect of temperature on protein denaturation is more relevant than the effect of time, as reported elsewhere( Reference Van der Poel, Blonk and Huisman 78 ) for the storage protein phaseolin. There was a positive correlation between the in vitro crude protein digestibility and the content of thiol groups in thermally treated isolated albumins from kidney beans (r 0·80; P<0·003; n 11) (Fig. 3). Correlations for the rest of the protein sources analysed were not significant. Some of these sources (Phaseolus beans and soyabeans) probably contained protease inhibitors and lectins. An increase in digestibility that resulted from heat inactivation of protease inhibitors and lectins could mask the decrease in digestibility resulting from heat-induced disulfide bonding. It has been suggested( Reference Selle, Liu and Cai 82 , Reference Selle, Liu and Cai 83 ) that the formation of disulfide bonds after heat treatment of sorghum is responsible for the decreased digestibility of kafirin. However, correlations between disulfide bond content of sorghum after thermal treatments and standardised ileal digestibility were not significant (P>0·05). Oddly, the disulfide bond content in the study of Selle et al. ( Reference Selle, Liu and Cai 83 ) did not increase along with the decrease in the thiol content. With increasing temperatures, there is also an increase in the surface hydrophobicity of the proteins in rapeseed protein isolate, which is a reflection of the exposure to the surface of the hydrophobic groups buried inside the molecules( Reference He, He and Chao 63 ). Surface hydrophobicity (S O ) increased from 600 in the unheated rapeseed protein isolate to 650, 1500 and 1100 after thermal treatments at 60, 80 and 100°C for 15 min, respectively( Reference He, He and Chao 63 ).

Fig. 3 Correlation between the content of disulfide (○) or thiol (●) groups and in vitro protein digestibility coefficient for thermally treated albumins isolated from kidney beans.
Table 5 Free thiol and disulfide bonds content, and protein digestibility coefficient after processing under different conditionsFootnote *
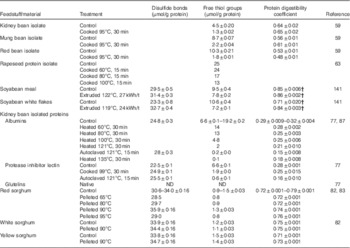
ND, not detected.
* Reported average ± standard error.
† Values correspond to faecal crude protein digestibility in minks.
Overall changes in the structure of protein can be estimated by comparing the denaturation enthalpy of native protein with that of the protein after processing (see online Supplementary Table S2). Denaturation enthalpy is a measure of the amount of energy required to denature a protein. Hence, denatured proteins exhibit a lower or a lack of denaturation enthalpy compared with the native ones. Protein denaturation usually increases with the intensity of the heat treatment and has been suggested as the main reason for improved protein digestibility in thermally heat-treated ingredients( Reference Abdollahi, Ravindran and Wester 70 , Reference Mauron 84 ). According to these authors, unfolding of the native secondary/tertiary structure of the protein could facilitate enzymic attack and their suggestion was based on several proteins of vegetable origin (for example, wheat and soya). The conditions applied during processing probably determine whether proteins become more susceptible (for example, unfolding and random coil formation) or more resistant (for example, protein aggregation and chemical changes to amino acids) to enzymic attack. However, enthalpy of denaturation can also increase after long thermal treatments as reported( Reference Wally-Vallim, Vanier and da Rosa Zavareze 85 ) for soyabean protein isolates prepared from heat-treated soyabeans at 40 and 80°C for 4, 8, 12 and 16 h. The denaturation enthalpy of this material decreased after 4 and 8 h of thermal treatment, but increased again with longer heating times. It is possible that protein refolding occurred at longer heating times, causing the denaturation enthalpy to increase. Most of the denaturation enthalpy observed, ranging from 36 to 70 % of the enthalpy in the original material (2·85 J/g), originated from glycinin, which was less denatured than β-conglycinin under these conditions.
Only a limited number of studies has been performed on the link between denaturation enthalpy and digestibility of processed vegetable protein sources( Reference Wally-Vallim, Vanier and da Rosa Zavareze 85 – Reference Sun, Mu and Sun 88 ). The positive correlation between the relative degree of denaturation (i.e. decrease of enthalpy after thermal treatment with respect to the enthalpy of the native material) and in vitro crude protein digestibility for protein-containing ingredients (i.e. sweet potato protein, cowpeas and soyabeans) was significant (r 0·49; P=0·003; n 34) (Fig. 4). However, these ingredients probably contained protease inhibitors. In that case, the relationship between the degree of protein denaturation and the in vitro crude protein digestibility would be somewhat confounded with increased apparent digestibility due to the heat inactivation of protease inhibitors. The amino acid sequence of sporamin (sweet potato protein) resembles that of Kunitz-type trypsin inhibitors, possibly explaining the increase in digestibility after denaturation( Reference Sun, Mu and Sun 88 ). Proteins from cowpeas and soyabeans can be classified under the same ‘Osborne’ type. However, their amino acid compositions are different, which possibly leads to different structural conformations (for example, secondary and tertiary) and explains the differences in the correlations between the relative degree of denaturation and in vitro digestibility (Fig. 4). In addition, whilst the in vitro digestibility of sweet potato protein and cowpeas was determined using multiple enzymes (for example, porcine pepsin and pancreatin), the digestibility of soyabean proteins was determined after incubation with only pepsin. Incubation with a single enzyme might lead to an underestimation of protein digestibility. For isolated albumins from P. vulgaris there is a trend for a negative correlation between the relative degree of denaturation and the in vitro digestibility (r –0·53; P=0·09; n 11) (Fig. 4). The degree of purity of the isolated albumin fraction is not described in that study( Reference Rocha, Genovese and Lajolo 87 ); thus this fraction might still contain trypsin inhibitors. The limited number of studies available, the inclusion of sources that contain protease inhibitors and the inconsistency in the results do not allow us to draw firm conclusions on the effects of protein denaturation on protein digestibility. The effect of protein denaturation on protein digestibility could be linked to the nature of the protein.

Fig. 4 Correlation between the relative degree of denaturation and in vitro crude protein digestibility coefficient of thermally treated isolated albumins from kidney beans (◊) and other protein sources (■, Ipomoea batatus; ▲, Vigna unguiculata; ●, Glycine max).
Effects of moisture
Water or steam is usually added as cofactors during processing in order to manage the moisture content and the physical properties of the processed material. The moisture content ranges between 12 and 18 % (w/w) for pelleting( Reference Abdollahi, Ravindran and Svihus 15 , Reference Chae and Han 76 ), whereas extrusion processing tolerates higher moisture inclusion ranging from 20 to 35 % (w/w)( Reference Chae and Han 76 ).
Proteins in dry conditions have high glass transition temperatures (Tg), which can be close to their degradation temperature( Reference Verbeek and van den Berg 89 , Reference Bier, Verbeek and Lay 90 ). The Tg can be reduced by the addition of water, which acts as a protein plasticiser, and decreases Tg approximately 10°C for every 1 % increase in moisture content( Reference Bier, Verbeek and Lay 90 ). Due to the small size of its molecules, water can move freely through the small openings of the structure of proteins. Water decreases the Tg by disrupting hydrogen bonds, van der Waals and ionic interactions that hold the structure of protein together, thereby increasing its flexibility( Reference Verbeek and van den Berg 89 ). Increased flexibility and rupture of the bonds also decrease the denaturation temperature of proteins. However, the flexibility of proteins is limited below water contents of 5–9 %. Exposure of hydrophobic amino acid residues due to changes in the conformation of proteins after the addition of water can lead to irreversible protein aggregation( Reference Towns 91 ). The denaturation temperature of sunflower (Helianthus annuus) oil cake globulins decreased from 189·5°C to 154·4, 133·4 and 119·9°C with increasing moisture contents of 0, 10, 20 and 30 %, respectively( Reference Rouilly, Orliac and Silvestre 92 ). The Tg of these proteins showed a similar pattern, decreasing from 180·8°C with no water to 5·3°C at 26 % moisture content. Similar results were also reported for β-conglycinin( Reference Kitabatake and Doi 93 ) and glycinin( Reference Kitabatake and Doi 93 , Reference Huson, Strounina and Kealley 94 ) from soyabean meal. The denaturation temperature of β-conglycinin and glycinin were 76·5°C and 93·3°C, respectively, with a moisture content of 94 %. However, when the moisture content was decreased to 29 %, the denaturation temperature of β-conglycinin was increased to 180°C and no denaturation temperature could be found for glycinin. The addition of water decreases the Tg of both native and denatured glycinin, with a faster rate for the native proteins( Reference Huson, Strounina and Kealley 94 ).
Thus, water addition increases the flexibility of protein structure and in combination with heat, shear or pressure, changes the effects of these factors on the secondary protein structure (Table 3), in vitro crude protein digestibility (Table 4) and protein solubility (see online Supplementary Table S1). Increasing the moisture content during extrusion processing, whilst keeping a constant temperature, increases protein denaturation and refolding, with the formation of new bonds. For example, the percentage of protein solubilised by a solution of 50 mm-DTT in a phosphate buffer after extrusion at 170°C of a soya protein, wheat gluten and wheat starch mix (weight ratio 60:40:5) increased from 10 to 30 when moisture content (w/w) was increased from 60 to 72 %( Reference Liu and Hsieh 81 ). This indicates that during processing the extents of both covalent and non-covalent interactions increase with increasing moisture contents, possibly related to unfolding, which is the rate-limiting step. The increased interaction between proteins could explain the reduction of the in vitro digestibility of soyabean meal with increasing moisture contents at constant temperature and extrusion screw speed (Table 4). Alternatively, inactivation of trypsin inhibitors could have been higher at lower moisture contents, leading to higher in vitro crude protein digestibility.
Changes in the secondary structure of proteins have been reported when moisture-including sources of heat were used (for example, using autoclaving, desolventisation/toasting) in contrast with dry sources of heat (for example, roasting, IR radiation) at similar temperatures (Table 3). This was the case for lentils (Lens culinaris), in which no effects were reported for dry heating at 120°C for 30 min, whilst autoclaving at similar temperatures (120°C for 20 min) increases the α-helices and random coil contributions. The latter treatment also reduces the contribution of the intramolecular β-sheets conformation in exchange for a larger amount of intermolecular β-sheets( Reference Carbonaro, Maselli and Nucara 30 ). However, the response of the secondary structure of proteins to changes in moisture could be source-dependent, as not all the ingredients analysed follow the same pattern. Whilst a dry source of heat at 120°C for 30 min induces the appearance of random coil structures in kidney beans, the same thermal treatment does not induce the formation of these structures in lentils( Reference Carbonaro, Maselli and Nucara 30 , Reference Carbonaro, Maselli and Dore 95 ). These differences could be related to the proportion of the different types of proteins in each ingredient (for example, globulins v. albumins), or to the inherent structural characteristics of the proteins of each source. In these studies( Reference Carbonaro, Maselli and Nucara 30 , Reference Carbonaro, Maselli and Dore 95 ) the seeds were autoclaved, along with the water that was used for soaking them (1:4, w/v) during 2 h.
Shear effects
Multiple definitions have been provided for shear. As defined for extrusion processing( Reference Marsman, Gruppen and van Zuilichem 96 ), shear is the dissipation of the mechanical energy input by friction of the particles inside the extruder barrel. The estimation of shear is based on the torque produced by the engine and on the calculation of specific mechanical energy (SME) input. Different levels of shear are involved in the equipment, which is commonly used for compound feed production. Pelleting consumes a low level of SME, ranging between 23 and 45 kJ/kg, and the levels are highly dependent on the fat level inclusion in the diet( Reference Richardson and Day 97 , Reference Fahrenholz 98 ) and the size of the die. The technology that is considered to cause the highest levels of shear is extrusion, with SME ranging from 839 to 1277 kJ/kg( Reference Fang, Zhang and Wei 68 ). As a system parameter, torque is the result of the combination of several process parameters, such as temperature, moisture content, feeder-screw speed, extruder-screw speed and screw configuration. Thus, any change in the process parameters during extrusion can lead to an alteration of the SME input. Separating the effects of SME input from other process parameters used during processing in complex systems such as extrusion is difficult, and literature describing changes in protein structure due to shear only is scarce.
The effects of simple shear flow on protein structure and function have been reviewed before( Reference Bekard, Asimakis and Bertolini 99 ). However, most of these research has been performed on model systems of proteins in solution( Reference Bekard and Dunstan 100 , Reference Dunstan, Hamilton-Brown and Asimakis 101 ), which do not resemble the conditions used during feed production. Simple shear of proteins can induce conformational changes to the structure of proteins by disrupting the secondary/tertiary structure, even at relatively low temperature, as illustrated by the work of Dunstan and colleagues( Reference Bekard and Dunstan 100 , Reference Dunstan, Hamilton-Brown and Asimakis 101 ).
Protein aggregation in soya isolates has been reported as a consequence of extrusion( Reference Fang, Zhang and Wei 68 ). Vital factors during extrusion, such as a high temperature and pressure, were suggested to be responsible for protein aggregation( Reference Fang, Zhang and Wei 68 ). However, with increasing SME levels, the molecular weight of the protein aggregates was reduced. The molecular weight of the aggregates was reduced by 5, 9 and 17 % at SME inputs of 1050, 1093 and 1277 kJ/kg, respectively, compared with the molecular weight at the 839 kJ/kg extruder setting( Reference Fang, Zhang and Wei 68 ). It was suggested by Meade et al. ( Reference Meade, Reid and Gerrard 54 ) that the mechanical forces during extrusion could break the peptide bonds in the amino acid chain (effects on primary protein structure), thus producing a similar effect as proteolysis.
Increased levels of non-covalent and covalent bonds, which lead to larger amounts of aggregation, were reported( Reference Marsman, Gruppen and De Groot 102 ) in soya proteins with increasing SME. In this experiment, shear contrasts were produced by adaptations to the screw configuration of the extruder. With the screw configurations that produced increased levels of shear, there was also a decrease in the solubility after the extraction of proteins with urea or DTT and the successive extractions with solutions of urea/DTT or DTT/urea. This could indicate that after extrusion at high mechanical energy inputs, proteins may interact by mechanisms other than the formation of disulfide or non-covalent bonds. These mechanisms could be covalent bonds related to crosslinking between amino acids( Reference Marsman, Gruppen and De Groot 102 ).
The formation of Maillard reaction products (and possibly also protein structural modifications) is affected by the interaction between temperature and mechanical energy( Reference Lei, Fulcher and Ruan 103 ). High SME inputs produced large amounts of Maillard reaction products when extrusion of a rice (Oryza spp.)–glucose–lysine model mixture was performed at barrel temperatures of 100 and 130°C, but not at 70°C( Reference Lei, Fulcher and Ruan 103 ). Increasing the screw speed from 80 to 140 rpm had limited effect on the in vitro crude protein digestibility of commercial soyabean meal after extrusion( Reference Marsman, Gruppen and Van der Poel 104 ).
Although there is little evidence that crude protein digestibility might be largely affected after shear-processing, the formation of crosslinked or Maillard-modified amino acids has a large impact on the nutritional value of the feed. The crosslinked and modified amino acids cannot be utilised after absorption( Reference Van Barneveld, Batterham and Norton 105 ), and therefore animal performance is decreased.
Particle size reduction processing
The amount of mechanical energy involved in particle size reduction is related to the type of equipment employed. SME ranges from negligible in roller milling to 104·4–126 kJ/kg for hammer milling( Reference Bitra, Womac and Chevanan 106 ) and 6·5–10·1 kJ/kg for the multicracker system( Reference Thomas, Vrij and Zandstra 107 ). Hammer and roller milling are the most frequently used technologies for particle size reduction of compound feed ingredients, although new technologies, such as the multicracker, have been developed( Reference Thomas, Vrij and Zandstra 107 ).
The effects of particle size reduction on digestibility has been analysed before( Reference L’Anson, Choct and Brooks 21 , Reference Lahaye, Ganier and Thibault 108 – Reference Valencia, Serrano and Lázaro 110 ). During particle size reduction, rupture of protein bodies from grains and legume seeds that contain storage proteins increases protein solubility( Reference Vishwanathan, Singh and Subramanian 111 ), which in turn could facilitate enzyme accessibility. However, only few studies report improved enzyme accessibility due to a larger area of exposure of the substrate( Reference Barakat, de Vries and Rouau 112 ). Few studies have been performed on the effects of particle size reduction on protein structure. This is probably related to the fact that particle size reduction is regarded as only a physical change and, as such, it is assumed not to have major effects on the structural characteristics of proteins.
Some of the technologies that are used to reduce particle size of ingredients involve the use of moisture, whilst others are performed under dry conditions. Wet-milling of maize dried distillers grains with solubles increased in vitro crude protein digestibility( Reference De Vries, Pustjens and Kabel 113 ). It is possible, as suggested by these authors, that the disruption of the cell wall structure leads to increased enzyme accessibility and that the improved in vitro digestion is not linked directly to changes in protein structure, although the latter point was not measured by the authors. Supporting this suggestion, is the observation that there were no changes in the secondary protein structure after wet-milling of silk from Philosomia cynthia ricini and Bombyx mori silkworms( Reference Rajkhowa, Wang and Kanwar 114 ). In contrast, when dry milling was used in the same study, the intramolecular β-sheet structures in these proteins completely disappeared, probably due to the processes involved in dry milling, including shear. The silk in that study was wet (i.e. addition of water) or dry milled using a ball mill followed by air jet milling. In the case of wet milling, water could act as a lubricant, thereby decreasing the amount of shear. It is possible that the disappearance of the intramolecular β-sheets after dry milling could increase protein digestibility as previously reported by Carbonaro et al. ( Reference Carbonaro, Maselli and Nucara 30 ). Furthermore, dry milling could have the additional benefit of the disruption of cell wall structures (as mentioned before for wet-milling), which can increase the access of enzymes for protein hydrolysis.
Conclusions
The structure of proteins influences enzyme accessibility for protein digestion. Some commonly used feed ingredients in swine diets exhibit structural constraints for digestion in their native proteins. Both primary processing of ingredients and compound feed production as a secondary treatment can affect the structure of native and partially denatured proteins. However, the link between the conformational changes and protein digestibility is not clear and further research should help to elucidate these underlying mechanisms. Most literature available that links protein structure to digestibility describes protein sources or ingredients which still contain protease inhibitors. It is necessary for future research to take this aspect into account and study the relationship between the structure of proteins (native or processed) and digestibility in isolated proteins, excluding the effect of protease inhibitors on digestibility. Correlations between structural properties of proteins (for example, free thiol content or degree of denaturation) with protein digestibility after processing seem to be dependent on the protein nature. Heat-induced modifications in the structure of proteins during primary processing render bonds (intra- and intermolecular) and structures, which become irresponsive to secondary processing and allow limited access to proteolytic enzymes. When proteins did not undergo complete denaturation during primary processing, secondary processing might change protein structure, with positive or negative effects on digestibility. Heat seems to be the parameter during secondary processing with the largest influence on structural changes of proteins. Nevertheless, other factors involved during processing (for example, moisture and shear) could also have a decisive role. However, the multifactorial essence of the complex processing technologies used in compound feed production does not allow yet us to disentangle and explain the effects of each separate processing factor. In this sense, model systems could aid in separating the processing factors and explaining their effects on the structural conformation and nutritional value of proteins.
Acknowledgements
The authors gratefully acknowledge the financial support from the Wageningen UR ‘IPOP Customized Nutrition’ programme financed by Wageningen UR, the Dutch Ministry of Economic Affairs, Agriculture & Innovation, WIAS, Agrifirm Innovation Centre, ORFFA Additives BV, Ajinomoto Eurolysine s.a.s and Stichting VICTAM BV.
S. S.-V. is the first co-author of the literature review and was the main writer of the initial version and coordinator of the activities related to planning of the structure and correcting the literature review. He was also in charge of the systematic search for relevant literature.
W. H. H., E. M. A. M. B., H. G. and A. F. B. v. d. P. contributed equally with planning of the structure of the literature review, corrections and proof-reading of the manuscript. They also contributed in suggesting sources of literature to be included in the review. As main supervisor of the project of S. S.-V., A. F. B. v. d. P. is the last co-author mentioned in the list.
There are no conflicts of interest.
Supplementary material
To view supplementary material for this article, please visit http://dx.doi.org/10.1017/S0954422416000056