Introduction
Rodents, mainly mice, have become the model for studies related to human physiology and genetics. However, the differences between rodents and humans in dietary habits, digestive strategies including coprophagy, nutrient requirements and nutrient–nutrient interactions suggest the pig may be a closer match than rodents for studying physiological functions relating to these research areas( Reference Baker 1 – Reference Roura, Humphrey and Klasing 4 ). The suitability of the pig as a model for human research is becoming accepted, particularly related to physiological factors and biomedical applications( Reference Gandarillas and Bas 2 , Reference Spurlock and Gabler 5 – Reference Bendixen, Danielsen and Larsen 7 ). Both humans and pigs are classified as omnivorous mammals and share similarities related to anatomical features of the gastrointestinal tract (GIT), such as the size of the compartments and the predominance of the colon rather than the caecum as the main fermentation site of plant/fibrous dietary components( Reference Gandarillas and Bas 2 , Reference Stephen, Correa-Matos and Donovan 8 ). Pigs have been used to study several areas relevant to human nutritional sciences( Reference Baker 1 , Reference Gandarillas and Bas 2 , Reference Labib, Erb and Kraus 9 – Reference Nielsen, Hartvigsen and Hedemann 11 ), including metabolic syndromes, obesity, bariatric surgery( Reference Gandarillas and Bas 2 , Reference Spurlock and Gabler 5 ), neuroscience, brain imaging( Reference Clouard, Meunier-Salaun and Val-Laillet 3 , Reference Sauleau, Lapouble and Val-Laillet 12 ), food allergies( Reference McClain and Bannon 13 ), alcohol intake( Reference Casani, Segales and Vilahur 14 , Reference Kubotsu, Hu and Carnahan 15 ) and mother–offspring interactions( Reference Guilloteau, Zabielski and Hammon 16 ). In addition, the sequencing of the pig genome has widened support for using the pig as a model for humans by revealing the similarities and differences between pigs and humans at the gene and chromosomal levels( Reference Lunney 17 ).
The present review provides a critical evaluation of the pig as a model for studies related to humans in areas that encompass nutritional chemosensing and hormonal profiles relevant to feed/food intake, the gut barrier function, the host–microbiota interactions and the relationship between nutrition and brain development and metabolism. In addition, the present paper assesses, as quantitatively as possible, how appropriate it would be to use the pig as a model for humans for each of the areas discussed.
Nutritional chemosensing
The chemosensory system has evolved to allow animals to discriminate between foods in their environment, and is believed to reflect species-specific dietary needs( Reference Li and Zhang 18 ). For example, humans have developed trichromatic vision, which was a key event relevant to food recognition and may have contributed to decrease the reliance on olfaction( Reference Gilad, Przeworski and Lancet 19 ). In contrast, pigs appear to have the largest olfactory gene repertoire of any animal studied, with 1,301 potential olfactory receptor genes( Reference Groenen, Archibald and Uenishi 20 ). The potential for pigs to become a model for human research in olfaction is relevant in the case of maternal transfer of dietary cues( Reference Oostindjer, Bolhuis and van den Brand 21 – Reference Langendijk, Bolhuis and Laurenssen 24 ).
Nutritional chemosensing is the science studying the perception of nutrients in biological systems which relates molecular mechanisms to changes in genomic, metabolic, physiological and behavioural parameters. Dietary nutrients are perceived in the oral cavity through the taste system in both pigs and humans( Reference Bachmanov and Beauchamp 25 , Reference Wellendorph, Johansen and Bräuner-Osborne 26 ).
Taste perception and the nutrient chemosensory system
Five basic tastes are accepted in humans: sweet, umami, salty, sour and bitter. In addition, fat perception and other nutrient sensing have been related to taste( Reference Bachmanov and Beauchamp 25 ). Sapid compounds solubilised in saliva are presented to the taste buds in the tongue papillae( Reference Matsuo 27 ). Taste perception involves a large set of taste receptors (referred hereinafter as TR for the receptors and TASR for the corresponding genes), which are expressed in the sensory cells of the taste buds and account for recognition of the tastants triggering a depolarisation of the cell and the subsequent signalling to the gustatory cortex of the brain through dedicated neuronal fibres( Reference Barretto, Gillis-Smith and Chandrashekar 28 ). Salty and sour sensing has been related to ligand-gated, transmembrane-channels: the epithelial Na channels (involving three genes ENaCα, β, γ) and the hydrogen-gated channels (involving two genes PKD1L3 and PKD2L1), respectively( Reference Bachmanov and Beauchamp 25 ). All other taste and nutrient receptors known to date are G-protein-coupled receptors (GPCR), including the family 1 (T1R), family 2 (T2R) and several other receptors related to amino acid (AA) and fatty acid (FA) sensing. In humans, the three genes of the taste receptor type 1 (TAS1R) subfamily have been related to umami and sweet tastes. They sense a limited range of l-AA (TAS1R1 and TAS1R3) and sugars (TAS1R2 and TAS1R3). The taste receptor type 2 (TAS2R) subfamily has evolved to identify potentially toxic compounds and elicits the bitter taste. In addition to the Tas1R family, AA and peptones are sensed in the oral cavity by five other GPCR receptors (mGluR1, mGluR4, GPRC6A, CaSR and GPR92). Medium- and long-chained FA are sensed by another set of five GPCR (GPR40, GPR41, GPR43, GPR84 and GPR120). Detailed reviews of the TR/TASR, including the standard nomenclature, are given for humans and rodents by Bachmanov & Beauchamp( Reference Bachmanov and Beauchamp 25 ), Wellendorph et al. ( Reference Wellendorph, Johansen and Bräuner-Osborne 26 ) and Foster et al. ( Reference Foster, Blank and See Hoe 29 , Reference Foster, Roura and Thomas 30 ).
The pig nutrient-sensing repertoire is less known but several in silico comparisons of taste receptors have been published in the last 3 years, after the appearance of the second annotation of the pig genome( Reference Li and Zhang 18 , Reference Groenen, Archibald and Uenishi 20 , Reference da Silva, de Jager and Burgos-Paz 31 – Reference Herrero-Medrano, Megens and Groenen 33 ). Single gene sequencing in pigs has characterised the porcine TAS1R3( Reference Kiuchi, Yamada and Kiyokawa 34 ). The gene sequences for the porcine umami dimer, TAS1R1/TAS1R3, and the glutamate receptor, mGluR1, have also been published( Reference Roura, Humphrey and Klasing 4 , Reference Humphrey, Tedó and Klasing 35 ). More recently a study including allelic variation and oral expression of the porcine TAS2R family indicated the potential role of the bitter receptors in environmental adaptation( Reference da Silva, de Jager and Burgos-Paz 31 ).
Evidence for expression of TR in the GIT outside the oral cavity has emerged from both pig and human genome studies( Reference Wellendorph, Johansen and Bräuner-Osborne 26 , Reference Foster, Roura and Thomas 30 ). For example, expression of the Tas1r3 gene has been identified in the tongue, heart, lung, stomach, intestine, liver, kidney and testis( Reference Kiuchi, Yamada and Kiyokawa 34 ). Other nutrient receptors have been found along the GIT including T1R in the small intestine and stomach( Reference Moran, Al-Rammahi and Arora 36 – Reference Zhang, Yin and Shu 38 ); AA and peptone receptors, GPRC6A, GPR92 and CaSR, in gastric mucosa( Reference Haid, Jordan-Biegger and Widmayer 39 ); and FA sensors, GPR40, 43 and 120, and seven TAS2R, in five different tissues of the GIT( Reference Colombo, Trevisi and Gandolfi 40 ).
Comparisons between pigs and humans in nutritional chemosensing
Pigs have a high number of taste buds (n 19 904) relative to other animal species. The number recorded for humans (n 6074) is about 30 % that for pigs. Most of these taste buds are on the tongue surface as part of the fungiform papillae or on the circumvallate papillae in pigs and humans( Reference Chamorro, de Paz and Fernandez 41 , Reference Roura, Humphrey and Tedo 42 ). Pigs have a larger number of fungiform papillae than humans and only two circumvallate papillae compared with eight to twelve in humans( Reference Miller and Reedy 43 ). However, the ratio of taste buds to adult body weight is similar for pigs and humans, as it is across many animal species( Reference Roura, Baldwin and Klasing 44 ).
Sensitivity of pigs compared with humans for the basic hedonic human tastes (sweet, umami, salty, sour and bitter) and homology between taste gene sequences are shown in Tables 1 and 2, respectively. Molecular mechanisms and taste sensitivity are generally similar between the two species for sweet, umami, sour and FA tastes. However, similarities are less for salty and low for bitter tastes.
Table 1 Efficacy of the pig model for humans in nutritional chemosensing; endocrine system; microbiota; and brain anatomy, development and imaging
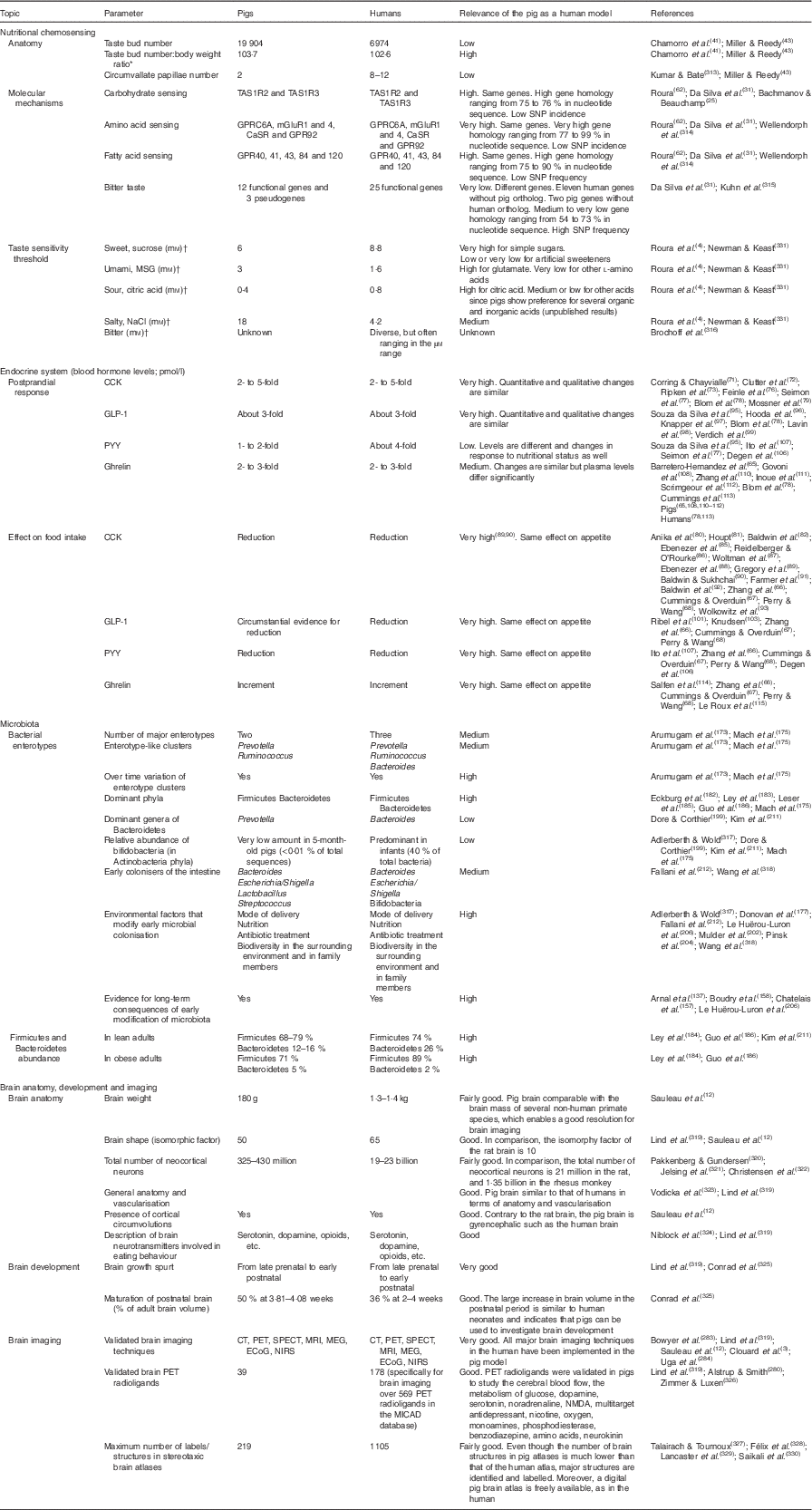
TAS1R, taste receptor type 1; TAS2R, taste receptor type 2; MSG, monosodium glutamate; CCK, cholecystokinin; GLP-1, glucagon-like peptide-1; PYY, peptide YY; CT, computed tomography; PET, positron emission tomography; SPECT, single photon emission computed tomography; MEG, magnetoencephalography; ECoG, electrocorticography; NIRS, near-IR spectroscopy; NMDA, N-methyl-d-aspartate.
* The adult body weights for a pig and for a human used in the calculation were 192 and 68kg, respectively.
† The preference tests in pigs consisted of double-choice tests including two solutions as described in Roura et al.( Reference Roura, Humphrey and Klasing 4 ). The threshold values for pigs refer to the lowest concentration of the taste active compound tested which resulted in significant (P<0·05) preference over water compared with 50 % (neutral value) (adapted from Roura et al. ( Reference Roura, Humphrey and Klasing 4 )). The threshold values for humans refer to the lowest concentration tested following the ascending forced choice triangle test method (Newman & Keast( Reference Newman and Keast 331 )), which resulted in significant (P<0·05) detection (E Roura, unpublished results).
Table 2 Studies on taste receptor and nutrient sensor genes in Sus scrofa compared with Homo sapiens Footnote *

TAS1R, taste receptor type 1; TAS2R, taste receptor type 2; IMP, inosine monophosphate; GMP, guanosine monophosphate; GLP-1, glucagon-like peptide-1.
* The homology percentage for pigs compared with humans was calculated between the amino acid sequences and between the nucleotide sequences for each gene.
† Porcine genes that have been identified as pseudogenes according to Da Silva et al. ( Reference da Silva, de Jager and Burgos-Paz 31 ).
‡ The porcine gene TAS2R134 has no known human homolog (Da Silva et al. ( Reference da Silva, de Jager and Burgos-Paz 31 )).
In relation to sweet tastes, the first studies in pigs from the 1950s were on appetite for sucrose (SUC)( Reference Lewis, Catron and Combs 45 , Reference Salmon-Legagneur and Fevrier 46 ). Kennedy & Baldwin( Reference Kennedy and Baldwin 47 ) also measured preferences by pigs for several sweet compounds and found similar recognition threshold concentrations in the diet for SUC and sodium saccharin (SAC) of about 5 to 10mm. The taste recognition threshold was higher for glucose (GLU) at between 10 and 30mm. Humans show a similar sensitivity to pigs for simple sugars and for two high-intensity sweeteners, sucralose and rebaudioside A( Reference Glaser, Wanner and Tinti 48 , Reference Roura, Shrestha and Diffey 49 ). However, pigs do not respond to aspartame, neohesperidin and thaumatin( Reference Glaser, Wanner and Tinti 48 , Reference Roura, Shrestha and Diffey 49 ), which are recognised as sweet by humans. SAC solutions above 100mm were rejected by pigs( Reference Lewis, Catron and Combs 45 ), suggesting an unpleasant sensation at high concentrations. This response is similar for humans, where high SAC concentrations elicit bitterness( Reference Galindo-Cuspinera, Winnig and Bufe 50 ).
Pigs have a preference for free l-AA including glutamic, aspartic, alanine, glutamine and lysine which have been related to the porcine umami taste receptor( Reference Roura, Humphrey and Klasing 4 ). In addition, preferences for serine, threonine, asparagine and hydroxyproline have also been reported in pigs( Reference Tinti, Glaser and Wanner 51 ). However, pig preferences for AA or sugars seem to increase with dietary deficiencies( Reference Guzmán-Pino, Sola-Oriol and Figueroa 52 , Reference Guzmán-Pino, Solà-Oriol and Figueroa 53 ). In contrast to the AA listed above, pigs have been shown to avoid branched-chained AA and tryptophan, presumably related to the onset of bitter taste( Reference Tinti, Glaser and Wanner 51 , Reference Tedo, Roura and Reina 54 , Reference Danilova, Hellekant and Jean-Marie 55 ). Compounds known to be bitter to humans such as pharmaceuticals, including antibiotics, caffeine, quinine HCl, among others, have also been shown to trigger avoidance in pigs( Reference Danilova, Roberts and Hellekant 56 , Reference Nelson and Sanregret 57 ).
Humans appear to be less sensitive to umami tastants than pigs. Umami taste in humans is stimulated by only two l-AA (glutamic and aspartic), whereas pigs recognise a wider array of l-AA( Reference Tinti, Glaser and Wanner 51 , Reference Tedo, Roura and Reina 54 ). The strong preference for non-glutamic AA in pigs is an important differential feature compared with humans. However, the umami receptor in laboratory rodents responds to an even wider array of l-AA compared with pigs( Reference Roura, Humphrey and Klasing 4 ).
Umami tastants in pigs, for example, monosodium glutamate, either alone or in combination with nucleotides, and some sweet tastants, stimulate nerve fibres identified with umami sensing( Reference Danilova, Roberts and Hellekant 56 ). Umami and sweet compounds are both perceived by dimeric taste receptors (TR) sharing one common receptor T1R3( Reference Li, Staszewski and Xu 58 ).
Characterisation of the umami dimer, TAS1R1/TAS1R3, and the glutamate receptor, mGluR1, revealed higher homologies of these porcine genes with the human orthologs compared with laboratory rodents( Reference Roura, Humphrey and Klasing 4 , Reference Humphrey, Tedó and Klasing 35 ). Studies of divergences and similarities in gene structure and expression between pigs and humans for the whole TASR repertoire showed high homology for the TAS1R subfamily coding for carbohydrates, AA and FA, but low homology for the TAS2R subfamily coding for bitter tastes( Reference da Silva, de Jager and Burgos-Paz 31 ). Recent publications indicate the pig has sixteen and the human twenty-five TAS2R genes( Reference Bachmanov and Beauchamp 25 , Reference da Silva, de Jager and Burgos-Paz 31 ). It may be speculated that the similarity in TAS1R gene structure between pigs and humans is consistent with the theory of parallel evolution, where both species are omnivorous and consumed diets with similar nutrient profiles. The lower number of TAS2R genes in pigs may indicate a higher resilience of pigs to bitter dietary compounds( Reference Groenen, Archibald and Uenishi 20 ). It has been suggested that the TAS2R receptors unique to humans tend to have a narrow specificity and may not have food-related significance particularly in light of their extra-oral expression( Reference Meyerhof, Batram and Kuhn 59 ). Da Silva et al. ( Reference da Silva, de Jager and Burgos-Paz 31 ) also reported a high incidence of non-synonymous polymorphisms in the porcine TAS2R repertoire when comparing fourteen different pig breeds across the globe. These findings are consistent with the view that bitter taste gene diversity is an adaptation within animal species( Reference Li and Zhang 18 , Reference Kosiol, Vinar and da Fonseca 60 ), including humans( Reference Campbell, Ranciaro and Zinshteyn 61 ), to their specific ecosystems. The porcine TAS2R is not a good model for the study of bitter agents in humans. However, the global widespread nature of pig breeds following human expansion may represent a unique model to study the role of taste in the adaptation to specific geo-ecosystems.
One limitation for using the pig as a model for humans to study taste sensitivity is a difference in methodology used between the two species. Threshold nutrient concentrations are determined in pigs using preference tests, often compared with water, whereas recognition thresholds are used in human studies. Compared with a recognition threshold, a preference threshold may require higher doses. Results from these two types of comparisons suggest that pigs are more sensitive to citric acid and SUC, but less sensitive to NaCl than humans( Reference Roura, Humphrey and Klasing 4 , Reference Roura 62 ). In addition, pigs and humans show strong discrepancies in the responses to most high-intensity sweeteners tested to date( Reference Glaser, Wanner and Tinti 48 , Reference Roura, Shrestha and Diffey 49 ).
Conclusion on using the pig as a model for human nutritional chemosensing studies
The chemosensing anatomy appears to be similar for pigs and humans with a similar ratio of taste buds in the mouth to mature body weight and the location of sensors throughout the GIT and other organs. The two species also show similarity in studies related to tasting simple sugars (sweet), glutamate (umami) and citric acid (sour). In contrast to humans, pigs do not have the same ability to taste some high-intensity sweeteners or non-glutamate AA and appear less sensitive to NaCl (salt). Overall, pigs are an excellent model for humans, when based on carbohydrate-, AA- and FA-sensing mechanisms. In contrast, the bitter sensory system of pigs and humans is diverse and characterised by species-specific features evolving from the adaptation to different ecosystems. Studying gene expression in human tissues can be difficult, particularly when using well-controlled nutrition intervention studies. The similarity in nutrient receptors and taste sensitivities between pigs and humans creates the opportunity to use the pig as a model in place of rodents for behavioural studies into chemosensing.
Endocrine regulation of food intake: gut–nutrient sensing and gut–brain communication
Sensory cells expressing TR in the GIT are part of the enteroendocrine system. Signalling of appetite or satiety in the gut can be both GIT hormone and nutrient specific( Reference Maljaars, Symersky and Kee 63 ). The release of GIT hormones is activated by fasting or food intake via intestinal receptors that respond to mechanical and/or chemical stimulation( Reference Ritter 64 ) including TR( Reference Barretero-Hernandez, Galyean and Vizcarra 65 ). Over forty GIT hormones have been identified with specific bioactivity( Reference Zhang, Ning and Handelsman 66 ) of which eight GIT hormones (cholecystokinin (CCK), glucagon-like peptide-1 (GLP-1), oxyntomodulin, peptide YY (PYY), apoA-IV, gastrin-releasing peptide and neuromedin B, gastric leptin and ghrelin) have been implicated in the regulation of food intake in mammals( Reference Cummings and Overduin 67 ). This section focuses on the following four hormones specifically released by the stomach and/or small and large intestine with published results in humans and pigs: CCK, GLP-1, PYY and ghrelin. The importance of these four GIT hormones in appetite regulation has been reviewed by Perry & Wang( Reference Perry and Wang 68 ). In the context of nutrient-induced release of satiety hormones it has been reported by Geraedts et al. ( Reference Geraedts, Troost and Tinnemans 69 ) that the release of CCK and GLP-1 in response to dietary proteins differs substantially between humans and rats, underlining the need for alternative, more human-like, animal models to study food intake regulation.
Once GIT hormones are released, they may exert their action via a neural or an endocrine route. The neural route involves binding of GIT hormones to local GIT receptors and subsequent signal transduction via afferent fibres of the abdominal vagal nerve to the brain. The endocrine route involves systemic transport of GIT hormones to the brain and by binding to brain receptors present in the area postrema, a brain structure serving as an interface between blood and brain, or by crossing the blood–brain barrier (BBB) and subsequent binding to receptors in specific brain regions. For proper translational research from pig to human, it is important that the kinetics of GIT hormones in blood follow a similar profile. For instance, the kinetics of circulating GLP-1 are similar in pigs and humans, but different in rats( Reference Larsen, Fledelius and Knudsen 70 ). This difference between the species is probably caused by the rapid inactivation of GLP-1 by circulating protease dipeptidyl peptidase-4 (DPP-4), which is more active in rats than in pigs and humans.
Similarities and differences of nutrient-induced release of satiety hormones and endocrine gut–brain communication in pigs and humans
Cholecystokinin
Depending on the amount and composition of the diet, CCK is secreted by the I cells in the duodenal and jejunal mucosa and also by neurons in the enteric nervous system. CCK contributes to satiation and satiety effects. The major effects of CCK are stimulation of gall bladder contraction, delay of gastric emptying and stimulation of pancreatic secretion( Reference Maljaars, Symersky and Kee 63 ). In pigs, CCK release is stimulated by a mixed meal from fasting to postprandial (30–120min) concentrations going from about 10pmol/l to about 30pmol/l, respectively, with a faster response when diets are enriched with starch than with fat( Reference Corring and Chayvialle 71 ). Clutter et al. ( Reference Clutter, Jiang and McCann 72 ) measured plasma CCK-8 in pigs, at fasting about 2pmol/l increasing to 10pmol/l at mixed meal feeding whereas Ripken et al. ( Reference Ripken, van der Wielen and van der Meulen 73 ) reported concentrations of 0·5pmol/l and 2pmol/l for fasting and postprandial, respectively. All experiments showed a 2- to 5-fold increase in plasma CCK-8 concentrations after mixed meal feeding. Another study in pigs( Reference Jakob, Mosenthin and Zabielski 74 ), using intraduodenal fat infusion, showed plasma CCK concentrations of approximately 5pmol/l both prior and following fat infusion. CCK release was higher to long-chain FA than to medium-chain TAG. In humans, CCK release is mostly stimulated by the presence of dietary protein, whereas carbohydrate provides a weaker stimulus( Reference Liddle, Goldfine and Rosen 75 ). To our knowledge, the effect of dietary protein on plasma CCK secretion has not been investigated to date in pigs. Plasma CCK concentrations are similar in pigs and humans, on average for humans at fasting 2pmol/l and at feeding 10pmol/l( Reference Feinle, Grundy and Otto 76 – Reference Mossner, Grumann and Zeeh 79 ). In both species the magnitude of the CCK response is dependent on the composition of the meal. The effects of single macronutrients or combinations thereof on CCK release have not been investigated systematically in humans or pigs. The pig is a valid model to investigate these relationships.
Anika et al. ( Reference Anika, Houpt and Houpt 80 ) administered CCK intravenously at a rate of 0·2nmol/kg per min for total doses of 0·4, 0·8, 1·7 and 3·4nmol/kg to pigs. The lowest dose of CCK reduced food intake by 13 % and the highest dose by 94 %. Houpt( Reference Houpt 81 ) showed that intravenous (iv) CCK-8 (the synthetic and bioactive part of the CCK molecule) infusion to pigs of 14, 28 and 56 pmol/kg per min decreased meal size in a dose-dependent manner. A dose of 28pmol/kg per min reduced food intake by 30–40 %. Similar results were found by Baldwin et al. ( Reference Baldwin, Cooper and Parrott 82 ) who studied hungry pigs working for food, thirsty pigs responding for water, and non-deprived pigs working for SUC. In this study CCK produced significant dose-related decreases in response rates for all three conditions.
Nutrients exert much of their effects through the CCK-1 receptor( Reference Holzer, Turkelson and Solomon 83 ). There are two regions where CCK acts to produce satiety: the nucleus tractus solitarius in the hindbrain and the medial-basal hypothalamus( Reference Reidelberger, Hernandez and Fritzsch 84 ). CCK acts both at CCK-1 receptors beyond the BBB and by a CCK-1 receptor-mediated mechanism involving abdominal vagal nerves to inhibit food intake( Reference Reidelberger, Hernandez and Fritzsch 84 ). CCK-1 antagonists, such as devazepide (DVZ), are commonly used in pig appetite and satiety studies( Reference Ebenezer, Vellucci and Parrott 85 ). DVZ easily passes the BBB following iv administration inducing a dose-related increase in food intake at doses ranging from 17·5 to 140µg/kg with maximum increases occurring at about 70µg/kg( Reference Reidelberger and O’Rourke 86 – Reference Ebenezer, de la Riva and Baldwin 88 ). Arterial injection of DVZ (0·1mg/kg) in pigs( Reference Gregory, McFadyen and Rayner 89 ) abolished the inhibition of food intake to duodenal infusion of emulsified fat and monoacylglycerols. However, it did not alter the inhibition of intake in response to oleic acid, to glycerol or to GLU, suggesting that monoacylglycerol-induced CCK secretion is mainly responsible for the satiety resulting from duodenal fat infusion in the pig. Baldwin & Sukhchai( Reference Baldwin and Sukhchai 90 ) reported that intracerebroventricular (icv) injection of DVZ (100µg) before the administration of 1µg CCK abolished the inhibition of GLU intake produced by CCK in pigs. However, DVZ itself had no effect on GLU intake. In a study by Farmer et al. ( Reference Farmer, Roy and Rushen 91 ), pigs were fasted for 24h, injected intravenously with DVZ at 70mg/kg and subsequently subjected to a feed motivation test (operant conditioning). The number of pushes, duration of eating and amount of feed eaten during the feed motivation test were all increased by fasting, and were further increased by DVZ injection, indicating that CCK induces satiation in pigs. An alternative CCK receptor antagonist used in pigs is 2-NAP (2-naphthalenesulfanyl-l-aspartyl-2-(phenethyl) amide), which does not cross the BBB. Baldwin et al. ( Reference Baldwin, de la Riva and Gerskowitch 92 ) revealed that iv administration (20 or 40mg/kg) of NAP injected before iv administration of CCK-8 (1µg/kg) abolished the inhibitory effects of CCK-8 on food intake in hungry pigs. Also, NAP abolished the inhibitory effects of CCK-8 on food intake in hungry pigs after icv injection of both compounds. Overall, these results indicate that endogenous CCK is involved in the regulation of satiety in pigs, in a way which is similar to what is known in humans( Reference Wolkowitz, Gertz and Weingartner 93 ), albeit the pig offers the possibility to conduct invasive mechanistic studies on CCK action.
Glucagon-like peptide-1
GLP-1 is mostly produced by the L cells in the distal small intestine and colon and contributes to satiety. It performs a variety of functions in the body such as inhibiting acid secretion and gastric emptying, while increasing insulin secretion from the pancreas in a GLU-dependent manner( Reference Holst 94 ). The secretion of GLP-1 is mediated by indirect, duodenal activated neurohumoral mechanisms, as well as by direct contact of nutrients with the distal small intestine( Reference Baldwin, Cooper and Parrott 82 ). Souza da Silva et al. ( Reference Souza da Silva, Haenen and Koopmans 95 ) showed that fasting plasma GLP-1 concentrations of 15pmol/l rose to 35pmol/l 1h after the beginning of a complete mash feed. Hooda et al. ( Reference Hooda, Matte and Vasanthan 96 ) showed that fasting plasma GLP-1 concentrations in pigs were approximately 8pmol/l and rose postprandial to 20pmol/l, 1–2h after initiation of the mixed-nutrient meal. The rise of plasma GLP-1 in response to intraduodenal infusion of GLU, fat and GLU–fat in pigs was modest (+5pmol/l) for GLU and intermediate (+10pmol/l) for fat, while the effect of combining GLU and fat was additive (+15pmol/l)( Reference Knapper, Morgan and Fletcher 97 ). To our knowledge, the effect of dietary protein on plasma GLP-1 secretion has not been investigated to date in pigs. In general, the GLP-1 responses to fasting/feeding in pigs are comparable with those in humans, with fasting concentrations of about 13pmol/l rising to 30–40pmol/l postprandial( Reference Blom, Lluch and Stafleu 78 , Reference Lavin, Wittert and Andrews 98 , Reference Verdich, Toubro and Buemann 99 ).
Although GLP-1 can cross the BBB, several studies suggest that peripheral GLP acts to reduce food intake primarily via activation of the vagal afferent nerve( Reference Asmar 100 ). Little information is available on the effect of GLP-1 on food intake in pigs. Ribel et al. ( Reference Ribel, Larsen and Rolin 101 ) showed that administration of a synthetic GLP-1 analogue reduced gastric emptying and therefore may induce satiation in pigs. In general, the pig is recognised as being a good model for studying human conditions( Reference Litten-Brown, Corson and Clarke 102 ), and in the case of GLP-1 analogues, it has found to be predictive of clinical findings in both reduction of hyperglycaemia (through improved insulin secretion and decreased glucagon secretion) and body-weight loss (through reduced gastric emptying and increased satiety)( Reference Knudsen 103 ). The icv administration of GLP-1 inhibited feeding in fasted rats and was counteracted by the icv injection of exendin 9–39, a GLP-1 receptor antagonist( Reference Turton, O’Shea and Gunn 104 ). No data are available in pigs.
Peptide YY
PYY is an anorexigenic hormone. It is a member of the pancreatic polypeptide (PP)-fold family of peptides. It is secreted by the L cells of the distal small-intestinal mucosa. The PYY-containing endocrine cells are found in highest numbers in the lower small intestine and colon. The gastrointestinal functions of PYY include inhibition of gastric acid and pepsin secretion, inhibition of pancreatic exocrine secretion, delay of gastric emptying and inhibition of jejunal and colonic motility( Reference Sheikh, Holst and Orskov 105 ). The secretion of PYY is stimulated by the presence of nutrients in the intestine. In humans, fats provide the strongest stimulus followed by carbohydrates and protein( Reference Degen, Oesch and Casanova 106 ). Little is known on the effects of intestinal nutrient loads on PYY secretion in pigs. Plasma PYY concentrations were higher (500pmol/l) in ad libitum-fed pigs compared with fasted pigs (200pmol/l)( Reference Ito, Thidarmyint and Murata 107 ). On the other hand, Souza da Silva et al. ( Reference Souza da Silva, Haenen and Koopmans 95 ) showed that fasting plasma PYY concentrations were approximately 700–800pmol/l, showing no response to a mixed-nutrient meal. Plasma PYY concentrations are lower in humans, ranging from 30pmol/l at fasting up to 116pmol/l after an intestinal nutrient load( Reference Seimon, Feltrin and Meyer 77 , Reference Degen, Oesch and Casanova 106 ). In the fast–refed condition, both single bolus injection (30mg/kg body weight) and iv infusion (0·25mg/kg per min) of PYY3–36 (the synthetic and bioactive part of the PYY molecule) suppressed feed intake in pigs( Reference Ito, Thidarmyint and Murata 107 ), suggesting that circulating PYY3–36 influences satiety and contributes to the termination of a meal such as in humans( Reference Perry and Wang 68 ).
Ghrelin
Ghrelin is an orexigenic intestinal hormone. In pigs, it is mostly produced in the oxyntic and cardiac gland and less commonly in the pyloric glands of the stomach( Reference Govoni, De Iasio and Cocco 108 ), with a similar pattern to that in humans( Reference Kojima and Kangawa 109 ). Ghrelin functions as a neuropeptide signal that reduces satiety and increases hunger. Govoni et al. ( Reference Govoni, De Iasio and Cocco 108 ) and Zhang et al. ( Reference Zhang, Yin and Li 110 ) showed that fasting increases plasma ghrelin concentrations from 10–25 to 50pmol/l in prepubertal gilts and weanling pigs, respectively. Ghrelin in pigs responded to changes in energy balance and the concentrations increased during fasting from approximately 25 to 75pmol/l( Reference Govoni, De Iasio and Cocco 108 , Reference Inoue, Watanuki and Myint 111 ). Barretero-Hernandez et al. ( Reference Barretero-Hernandez, Galyean and Vizcarra 65 ) reported postprandial plasma ghrelin concentrations of 6pmol/l, increasing to 10pmol/l at fasting in pigs. Scrimgeour et al. ( Reference Scrimgeour, Gresham and Giles 112 ) suggested that the dynamics in plasma ghrelin concentrations in the pig appear to be strongly influenced by prolonged fasting and change from 15pmol/l (feeding) to 100pmol/l (fasting). These ghrelin responses to fasting/feeding are comparable with those observed in humans( Reference Blom, Lluch and Stafleu 78 , Reference Cummings, Purnell and Frayo 113 ). However, the concentrations in humans are higher, being approximately 150pmol/l postprandial and 250pmol/l at fasting.
Salfen et al. ( Reference Salfen, Carroll and Keisler 114 ) showed that there was an increase in body weight of weanling pigs given exogenous ghrelin chronically, suggesting that feeding behaviour was influenced by the treatment. Ghrelin stimulates food intake in humans too. However, in vagotomised patients, ghrelin does not increase food intake, suggesting that an intact vagus nerve is required for exogenous ghrelin to increase appetite in humans( Reference Le Roux, Neary and Halsey 115 ). No pig studies have been conducted addressing the contribution of the vagus nerve to the orexogenic action of ghrelin.
Conclusions on nutrient-induced secretion of satiety hormones and on endocrine gut–brain communication in pigs and humans
Basal or fasting plasma concentrations of CCK and GLP-1 are similar in pigs and humans. The nutrient-induced increase of CCK and GLP-1 concentrations is also similar in both species. Plasma CCK increases postprandial 2- to 5-fold, whereas GLP-1 increases 3-fold in pigs and in humans. Therefore, pigs are a useful model for the investigation of the effects of single (macro) nutrients or combinations of nutrients on CCK- and GLP-1 release. Fasting and postprandial plasma concentrations of PYY in pigs are 10- to 20-fold higher compared with humans. The PYY response of pigs to feeding or intestinal infusion of nutrients (in terms of percentage increase of PYY concentration) seems to be smaller than in humans. For ghrelin the reverse is true; humans show 2- to 5-fold higher plasma concentrations than pigs. The responses of ghrelin to fasting or feeding are comparable in pigs and humans: 2- to 3-fold increases of ghrelin at fasting as compared with fed conditions. The relevance of the pig as a model for human GIT hormone dynamics seems therefore CCK and GLP-1>ghrelin>PYY.
Many pig studies support the human-like action of CCK on food intake regulation, but far fewer studies are available on the actions of GLP-1, PYY and ghrelin. Nevertheless, for GLP-1, PYY and ghrelin, human-like actions on food intake regulation have been reported in pigs( Reference Sternini, Anselmi and Rozengurt 116 ). Therefore, from an endocrine perspective, the pig is a suitable large animal model for the study of the humoral pathways of gut–brain communication as summarised in Table 1.
Gastrointestinal tract permeability and detoxification systems
Current outline of research in nutrition, gut-barrier and defence systems
An important aspect of the GIT function refers to metabolic disorders and obesity, which in humans are partially driven by excessive intake of high-energy diets and may be programmed early in life( Reference Vickers 117 , Reference Portha, Fournier and Kioon 118 ). Unbalanced, high-energy/fat and low-fibre diets may alter GIT permeability, allowing translocation of gut pro-inflammatory microbial-associated molecular patterns (MAMP) such as lipopolysaccharide (LPS) into the body and the development of metabolic inflammation, as demonstrated in mice( Reference Cani and Delzenne 119 ). These MAMP may then promote adipose tissue expansion, insulin resistance, metabolic disturbances and fat deposition.
The GIT is complex, comprising the mucus, the epithelial monolayer and the enteric immune system that includes intestinal epithelial cells( Reference Lallès 120 ). The intestinal epithelium participates in digestion and absorption, while tightly restricting body access of deleterious components. Passage of compounds across the epithelium is mainly regulated by tight paracellular junctions and by macromolecular uptake transcellular mechanisms( Reference Keita and Soderholm 121 ). LPS entry can be transcellular via the chylomicron pathway, following FA absorption, but also paracellular when the transcellular route is altered( Reference Mani, Weber and Baumgard 122 ). These mechanisms appear relevant to stress-related diseases (for example, gut chronic inflammation), the metabolic syndrome and obesity in humans( Reference Miele, Valenza and La Torre 123 – Reference Horton, Wright and Smith 125 ).
Besides permeability, the intestinal mucosa is equipped with defence systems including epithelial intestinal alkaline phosphatase (IAP) and inducible heat shock proteins (HSP). IAP is produced by the enterocyte and acts as a major anti-inflammatory enzyme through two mechanisms: detoxification, by dephosphorylating pro-inflammatory MAMP (for example, LPS), and control of local (and systemic) inflammation through a down-regulation of the Toll-like receptor 4-triggered NF-κB activation and of inflammatory cytokine production( Reference Lallès 126 ). Intestinal epithelial cells are chronically exposed to a harsh environment and toxic substances and they have developed inducible HSP (HSP27, HSP70) as anti-inflammatory and antioxidant cytoprotection mechanisms( Reference Arnal and Lallès 127 ). Inducible HSP are involved in intracellular protein trafficking, with many functional implications, including protection against potentially invasive compounds and organisms( Reference Arnal and Lallès 127 ). Inducible HSP are produced in response to diverse microbial components and related metabolites (for example, LPS, butyrate) in vitro ( Reference Lallès 120 ). However, in vivo data are scarce.
Alterations in the function of the intestinal barrier and defence systems may lead to chronic inflammatory diseases( Reference Pastorelli, De Salvo and Mercado 128 ). Conversely, dietary approaches aimed at reducing intestinal permeability and/or stimulating IAP and inducible HSP may contribute to prevent or treat such diseases.
This section summarises comparisons between the pig and the human for intestinal physiology of permeability and its neuroimmune regulation, detoxification and defence systems, their dietary modulation and their early programming.
Similarities between pigs and humans
The pathophysiology, molecular basis and neuroimmune regulation of the intestinal barrier when under stress have been described for rodent models( Reference Keita and Soderholm 121 ). In summary, the mechanism involves hypothalamic corticotropin-releasing factor (CRF), central and peripheral CRF receptors, degranulation of mucosal mast cells and release of various bioactive mediators. Large quantitative inter-species differences exist for intestinal permeability of small, medium-size and large molecules. Notably, pigs are closer to humans than rodents for both trans- and para-cellular permeability values( Reference Nejdfors, Ekelund and Jeppsson 129 , Reference Wallon, Yang and Keita 130 ). Intestinal permeability regulation in pigs also involves enteric nerve activation, CRF, mast cells and released tryptase and TNF-α( Reference Lallès 120 , Reference Smith, Clark and Overman 131 , Reference Overman, Rivier and Moeser 132 ). Data are limited in humans, but they indicate essentially the same regulations( Reference Wallon, Yang and Keita 130 , Reference Vanuytsel, van Wanrooy and Vanheel 133 ).
MAMP detoxification function by IAP is conserved across species( Reference Yang, Wandler and Postlethwait 134 ). IAP is highly expressed along the villous epithelium of the small intestine in pigs( Reference Lackeyram, Yang and Archbold 135 ) and humans( Reference Tuin, Poelstra and de Jager-Krikken 136 ). IAP activity is 10- to 15-fold higher in the distal ileum compared with proximal colon in both species( Reference Tuin, Poelstra and de Jager-Krikken 136 , Reference Arnal, Zhang and Messori 137 ). In pigs, IAP activity is drastically reduced after weaning and may cause post-weaning intestinal alterations( Reference Lackeyram, Yang and Archbold 135 ). Depressed IAP is also suspected in various inflammatory diseases in humans( Reference Lallès 126 ). Administration of exogenous IAP has strong anti-inflammatory effects in both species( Reference Lallès 126 , Reference Tuin, Poelstra and de Jager-Krikken 136 , Reference Beumer, Wulferink and Raaben 138 , Reference Lukas, Drastich and Konecny 139 ). Plasma LPS is a marker of metabolic inflammation in humans( Reference Laugerette, Vors and Peretti 140 ). Intake of saturated fat is consistently reported to increase plasma LPS in humans( Reference Amar, Burcelin and Ruidavets 141 ) and pigs( Reference Mani, Hollis and Gabler 142 ), intestinal transport of LPS in pigs( Reference Mani, Hollis and Gabler 142 ) and plasma IAP in humans( Reference Domar, Karpe and Hamsten 143 ).
Differences between pigs and humans, or pig studies with no equivalent in humans
Differences exist between humans, pigs and rodents for IAP gene copies (n 1, 2 and 2, respectively) and their chromosome location( Reference Yang, Wandler and Postlethwait 134 ). IAP distribution along the small intestine is opposite between pigs and rodents, the former having higher IAP activity in the ileum and lower in the duodenum compared with rodents( Reference Lallès 126 , Reference Fan, Adeola and Asem 144 ). The distribution of IAP along the human intestine is yet to be elucidated. Human cell line Caco2 and porcine IPEC-I display inducible HSP (for example, HSP70)( Reference Lindemann, Grohs and Stange 145 , Reference Yi, Hou and Wang 146 ). However, data on intestinal HSP25 or HSP70 in human tissues are lacking. As a prototypic example of dietary modulation of intestinal defence systems, l-glutamine supplementation has been shown to improve the morphological integrity and barrier function of the intestines in humans( Reference Benjamin, Makharia and Ahuja 147 ) and pigs( Reference Ewaschuk, Murdoch and Johnson 148 , Reference Zhong, Zhang and Li 149 ). However, these studies are difficult to compare due to many differences in experimental conditions. Zn is a key element for intestinal and body homeostasis. One in vitro study with human (Caco2) and porcine (IPEC-J2) intestinal epithelial cells revealed cell line differences in permeability and Hsp70 responses to Zn, suggesting inter-species differences( Reference Lodemann, Einspanier and Scharfen 150 ). However, in vivo data in humans are lacking.
The concept of ‘developmental origin of health and disease’, linking early-life malnutrition (deficiency or excess) to metabolic diseases was formulated two decades ago( Reference Hales and Barker 151 ). The effects of nutrition in early life on gene expression and potential long-term effects have become a discipline of high interest in human( Reference Nehring, Kostka and von Kries 152 , Reference Mennella 153 ) and animal( Reference Oostindjer, Bolhuis and van den Brand 21 , Reference Hepper, Wells and Millsopp 154 ) models. However, data on how early-life gene programming may affect intestinal function and defence systems are limited in humans( Reference Lallès 155 , Reference Lallès, Michel and Theodorou 156 ). Various dietary components, including protein, fat, methyl donors and fibre, influence gene expression in the intestines( Reference Lallès 155 , Reference Lallès, Michel and Theodorou 156 ). The pig as a model for humans has a high potential value in this area of research, but comparative studies are limited( Reference Arnal, Zhang and Messori 137 , Reference Chatelais, Jamin and Gras-Le Guen 157 – Reference Arnal, Zhang and Erridge 159 ). For example, high-protein milk formula transiently altered ex vivo ileal permeability in piglets and increased responses to LPS challenge in young adults( Reference Chatelais, Jamin and Gras-Le Guen 157 ). Neonatal dietary protein excess also led to long-term alterations in colonic barrier function under oxidant stress in female pigs( Reference Boudry, Jamin and Chatelais 158 ). Finally, alterations in mother-to-offspring transmission of GIT microbiota (for example, using antibiotics) had long-term consequences on IAP and iHSP along the GIT in pigs( Reference Arnal, Zhang and Messori 137 , Reference Arnal, Zhang and Erridge 159 ).
Intra-uterine growth retardation (IUGR) is a risk factor for the metabolic syndrome and obesity, possibly through low-grade inflammation( Reference Lakshmy 160 , Reference Salam, Das and Bhutta 161 ). For example, the prevalence of the metabolic syndrome was found to be 10-fold higher in human subjects weighing less than 3·0kg at birth compared with those with more than 4·3kg of birth weight( Reference Salam, Das and Bhutta 161 ). IUGR in the pig occurs naturally for some pigs in litters( Reference Ferenc, Pietrzak and Godlewski 162 ). IUGR piglets display immature gut, higher HSP70 both in utero and after birth, and altered pro-inflammatory NF-κB signalling pathway( Reference Zhong, Li and Huang 163 – Reference D’Inca, Kloareg and Gras-Le Guen 165 ). The IUGR piglets fed high-protein milk formula had higher ileal permeability and altered neuronal regulation of the gut barrier function later in life( Reference Boudry, Morise and Seve 166 ). Finally, the GIT microbiota is an important modulator of gut development( Reference Lallès, Michel and Theodorou 156 , Reference Weng and Walker 167 ).
Conclusion
The available literature suggests that basic mechanisms of intestinal permeability and defence systems are conserved across species, including pigs and humans. Functional permeability studies ex vivo suggest the pig to be close to the human. However, data are scant for intestinal IAP and inducible HSP defence systems in humans, and only indirect evidence suggests some similarities and differences between pigs and humans. Data on pig nutrition and gut health are numerous( Reference Lallès, Bosi and Smidt 168 – Reference Lallès and Guillou 170 ), making this species valuable to human nutrition research, due to anthropometric, dietary and GIT anatomical and physiological similarities. Moreover, nutritional programming of intestinal gene expression and long-term effects on growth and health can be assessed in this out-bred species and should be relevant to humans.
Host–microbiota interactions
One of the main aspects in the study of GIT function is the microbial population. Animals are associated with a diverse microbial community, primarily consisting of symbiotic and commensal bacteria. Mammalian bacterial diversity has been related to phylogeny and claimed to be influenced by the host diet, increasing in meat eaters compared with non-meat eaters( Reference Ley, Hamady and Lozupone 171 ). The GIT microbiota of modern humans is that of omnivorous primates. Original studies of the GIT microbiota focused on their role in inflammatory diseases, with the view that bacteria were pathogens only. However, the importance of the microbiota has been revisited in the past decade. It is now widely accepted that the GIT microbiota play a crucial role in maintaining homeostasis. The complex and intimate relationship between GIT microbial communities and its host is becoming clearer, due, in part, to large-scale microbial genome-sequencing programs( Reference Del Chierico, Vernocchi and Bonizzi 172 ). Metagenomic sequencing of total community DNA provides information about both the phylogenetic representation as well as functional genes. Interrogation of metagenomic information has revealed three distinct ‘enterotypes’ in the human microbiota that are identifiable by changes in the population of at least one of the three genera: Bacteroides, Prevotella and Ruminococcus ( Reference Arumugam, Raes and Pelletier 173 ). Enterotypes are not limited to humans, but also occur in mice( Reference Hildebrand, Nguyen and Brinkman 174 ) and pigs( Reference Mach, Berri and Estelle 175 ). Advancement in sequencing total RNA (metatranscriptomics), identifying total proteins (metaproteomics) and total metabolites (metametabolomics) has added further knowledge on the GIT ecosystem complexity both in humans and in pigs( Reference Lamendella, VerBerkmoes and Jansson 176 , Reference Donovan, Wang and Li 177 ). The use of an ecosystems biology approach, in association with ‘omics approaches, will lead to a more complete understanding of the complex interactions between the thousands of bacterial species in the GIT and the host( Reference Erickson, Cantarel and Lamendella 178 ).
The physiological similarity between humans and pigs in GIT development, digestive function, and gastrointestinal fermentation profiles (the colon being the main site of bacterial fermentation in pigs and humans) suggests that the pig is preferred over other non-primate models for digestive and metabolic disease studies relating to humans( Reference Guilloteau, Zabielski and Hammon 16 , Reference Le Bourgot, Ferret-Bernard and Le Normand 179 , Reference Heinritz, Mosenthin and Weiss 180 ). Moreover, the pig is a human-sized omnivorous species. The pig has been extensively used as a model for nutritional studies as its protein and lipid metabolism is comparable with humans( Reference Litten-Brown, Corson and Clarke 102 ). Increased knowledge of the pig microbiota composition and structure along the intestinal compartments being similar to humans further supports the pig as an ideal biomedical model for humans( Reference Mach, Berri and Estelle 175 , Reference Zhao, Wang and Liu 181 ). This section outlines the similarities and differences of the pig and human in host–microbiota interactions.
Similarities between pigs and humans in gut microbiota
Dominant phyla
The largest microbiota of the body is located in the GIT and the set of gene products provides a diverse range of biochemical and metabolic activities to complement host physiology. Hundreds of species are present in the GIT lumen, only belonging to a few microbial phyla. Firmicutes and Bacteroidetes are the two dominant bacterial phyla in the human and mouse gut, with the Proteobacteria, Actinobacteria, Fusobacteria and Verrucomicrobia phyla as subdominant phyla( Reference Ley, Hamady and Lozupone 171 , Reference Eckburg, Bik and Bernstein 182 – Reference Ley, Turnbaugh and Klein 184 ). Similarly, the GIT microbiota in pigs, as well as in wild suidae, mainly consists of the Firmicutes and Bacteroidetes phyla( Reference Leser, Amenuvor and Jensen 185 , Reference Guo, Xia and Tang 186 )(Table 3). Recently two different enterotype-like clusters, primarily distinguished by unclassified Ruminococcus and Prevotella, have been identified in pig faeces( Reference Mach, Berri and Estelle 175 ). Their phylogenetic composition was highly similar to two of the enteroptypes described in humans( Reference Arumugam, Raes and Pelletier 173 ). Interestingly, in pigs, as in humans, enterotype-like clustering distribution can vary within an individual over time( Reference Knights, Ward and McKinlay 187 ).
Table 3 Mean values of the amount of total SCFA throughout life in pig and human faeces

* SCFA content in meconium; values adapted from Midtvedt & Midtvedt( Reference Midtvedt and Midtvedt 414 ).
† Commercial pigs (Large White × Landrace × Pietrain breed) weaned 28d of age, data expressed per kg of faecal DM (Montagne et al. ( Reference Montagne, Le Floc’h and Arturo-Schaan 415 )).
‡ 5d post-weaning.
§ Post-pubescent (6·4 and 7·5 months-old Large White × Landrace × Pietrain breed) and gestating sows (1·5–2 years-old Large White × Landrace breed), data expressed per kg of faecal fresh matter (I Le Huerou-Luron, unpublished results).
Postnatal and early life microbial colonisation
During the first few hours after birth (postnatal), contact with environmental and colonising bacteria is essential for healthy intestinal and immune maturation. The major role of microbiota in the development of the neonatal GIT was confirmed in conditions where colonisation was modified early in life through exposure to micro-organisms of maternal and environmental origins, through nutrients consumed and through antibiotic treatments. During infancy, the composition of the intestinal microbiota is unstable and more variable than in older children and adults. Diet-induced adaptation of the microbiota may vary from the proximal to the distal parts of the intestine. The composition that is typically measured from faecal samples does not reflect the large bacterial diversity along the intestinal tract. Animal models, specifically cannulated pigs, are useful for obtaining a better understanding of the interactions between microbiota present in different niches of the intestine and physiology relevant to humans( Reference Saraoui, Parayre and Guernec 188 ). Studies with germ-free piglets clearly show that bacteria are essential for the growth and development of the digestive tract( Reference Chowdhury, King and Willing 189 ). The comparison of gene expression profiles in enterocytes of germ-free compared with conventional piglets has brought insight on the impact of microbiota on the GIT function( Reference Chowdhury, King and Willing 189 ). Bacterial colonisation induces the maturation and function of several components of the mucosal immune system and defence in order to prevent inflammatory responses that would compromise the barrier function( Reference Chowdhury, King and Willing 189 – Reference Hooper 191 ). In humans, neonates compared with older individuals have a decreased innate defence, a low production of IgA and a defective interaction between dendritic cells, T lymphocytes and regulatory T cells( Reference El Aidy, Dinan and Cryan 192 ). Similarly, the mucosal immune system is essentially absent in the neonatal piglet, even though the systemic immune tissue is well developed( Reference Bailey, Haverson and Inman 193 ), and piglets begin to synthetise secretory IgA from the second week of age( Reference Le Huërou-Luron and Ferret-Bernard 194 ). The balance between T lymphocytes helper 1 and helper 2 responses in human and pig neonates is skewed toward the helper 2 profile, resulting in a high susceptibility to intracellular pathogen infection( Reference Le Bourgot, Ferret-Bernard and Apper-Bossard 195 , Reference Adkins, Leclerc and Marshall-Clarke 196 ). The impaired protection of the neonate against infections may be partly attributed to a deficient secretion of interferon( Reference Le Bourgot, Ferret-Bernard and Apper-Bossard 195 , Reference Wilson, Westall and Johnston 197 ).
It only takes a few hours for bacteria to appear in the faeces of mammalian neonates( Reference Thompson, Wang and Holmes 198 , Reference Dore and Corthier 199 ). Facultative anaerobic bacteria, such as Proteobacteria, are the first colonisers. These bacteria reduce oxygen concentration in the GIT and allow strict anaerobes, such as members from the genus Bacteroides and the phyla Actinobacteria and Firmicutes, to colonise the intestine. During the first year of life in humans and the first 6 months in pigs, the intestinal microbiota composition fluctuates widely between individuals and over time, before resembling the adult status (Table 4). The potential use of piglets as a model for human studies is reinforced by the early colonisers, Bacteroides and Escherichia/Shigella being similar in humans. However, the substantial presence of Lactobacillus and Streptococcus in pigs is unparalleled in humans.
Table 4 Comprehensive summary of the existing literature on the relationship between nutrition and brain composition/development in pig models

FA, fatty acids; ARA, arachidonic acid; DPA, docosapentaenoic acid; LA, linoleic acid; ALA, α-linolenic acid; MCT, medium-chain TAG; l-DOPA, l-3,4-dihydroxyphenylalanine; HC, high cholesterol; LC, low cholesterol; ppm, parts per million.
* Swainsonine is a plant toxin.
† Quercetin is a dietary polyphenol with potential health benefits.
Early disturbances of the microbial colonisation process, such as induced by high-hygiene environments or by antibiotic treatment, have major consequences for the developmental sequence of the GIT microbiota and for host metabolism( Reference Cox and Blaser 200 ). An advantage of the porcine model is the flexibility to compare different early environmental-rearing conditions, using, for example, outdoor and indoor sow-reared piglets or isolator-reared neonates. Mulder et al. ( Reference Mulder, Schmidt and Stokes 201 ) showed large differences in composition of ileal-adherent microbiota between outdoor and indoor sow-reared animals, which corresponded to major differences in intestinal immune activation. Excessive hygiene appears to interfere with the normal processes of bacterial stabilisation and alters immune development. Mulder et al. ( Reference Mulder, Schmidt and Lewis 202 ) showed that the succession of events that lead to a stable adult microbiota depends on colonisation during the first 2d of life, and also on continuous exposure to highly diverse microbiota during the early development at least up to weaning at 4 weeks of age. The use of antibiotics, in combination with stressors in early life, was shown to affect adult pig microbiota and intestinal gene expression, including genes involved in immune-related processes( Reference Schokker, Zhang and Vastenhouw 203 ). This observation in pigs corroborates human studies indicating that changing the environmental conditions, and in particular microbial exposure, throughout early life affects the development of immune diseases( Reference Pinsk, Lemberg and Grewal 204 ). Whether inducing early change in immune homeostasis by modifying microbiota would lead to different sensitivity of pigs to infectious or inflammatory challenge, such as recently reported with early spray-dried supplementation( Reference Boyer, D’Costa and Edwards 205 ), warrants further investigation.
Many beneficial strategies have been suggested to strengthen the postnatal development of presumably beneficial microbiota and GIT functions, including: changing the composition of maternal food during gestation and lactation; changing the composition of infant formulas; and favouring breast-feeding over formula-feeding during the suckling period( Reference Heinritz, Mosenthin and Weiss 180 , Reference Le Huërou-Luron, Blat and Boudry 206 ). For example, feeding neonatal piglets with formula supplemented with prebiotics increased the bacterial numbers by 5-fold, the content of folic acid by 53 % and growth of the colon( Reference Aufreiter, Kim and O’Connor 207 ). Similarly, supplementation of the sow diet with prebiotics during gestation and lactation was associated with 50 % greater fermentative activity of the caecal microbiota, accelerated development of the intestinal immunity, and improved intestinal protection by increasing ileal Peyer’s patch production of secretory IgA in the offspring by 46 %( Reference Le Bourgot, Ferret-Bernard and Apper-Bossard 195 ). Faecal secretory IgA also increased by 170 % in healthy infants who receive a prebiotic-supplemented formula( Reference Scholtens, Alliet and Raes 208 ). These results in pigs and humans underline the key role of maternal nutrition during pregnancy in supporting neonatal development of the GIT immune system via modulation of microbiota.
The health benefits of breast-feeding have been recognised for a long time. Breast-feeding is associated with earlier colonisation with bifidobacteria, partly in relation to the presence of oligosaccharides in human maternal milk( Reference Donovan, Wang and Li 177 ). As in humans, breast-fed piglets showed lower intestinal growth and permeability compared with high protein formula-fed ones( Reference Boudry, Morise and Seve 166 , Reference Donovan, Wang and Li 177 ). One major issue in human studies on the effect of breast- v. formula-feeding on gut function is the great number of confounding factors which are difficult to circumvent, including quantification of food intake in breast-fed infants, variable length of exclusive breast-feeding, and variability of the composition of milk formulas. Animal models are used to help control these confounding factors, in particular, use of an automatic milk feeder that provides neonatal piglets with artificial milk as similar as possible to maternal milk( Reference Morise, Seve and Mace 209 ). These studies using a formula-fed piglet model provide strong support for the idea that short dietary changes before weaning associated with a modification of the early intestinal bacterial colonisation can have a long-term impact on the severity of inflammatory responses without changing the basal physiology of the intestinal barrier and cytokine profile in the intestine( Reference Chatelais, Jamin and Gras-Le Guen 157 , Reference Boudry, Jamin and Chatelais 158 ). No similar data are available from human studies due to the invasive procedure required for intestine functionality research. However, breast-feeding is clearly associated with lower incidence of necrotising enterocolitis and diarrhoea in both human and pig neonates( Reference Le Huërou-Luron, Blat and Boudry 206 , Reference Siggers, Siggers and Thymann 210 ).
Differences between pigs and humans in gut microbiota
Dominant phyla
Differences in the most abundant genera exist between the human and the pig intestinal microbiota( Reference Heinritz, Mosenthin and Weiss 180 ). Belonging to the Bacteroidetes phylum, the most abundant genus is Bacteroides in humans, averaging 9 to 42 % of total bacteria( Reference Dore and Corthier 199 ), while the most abundant genus is Prevotella in weaned pigs, accounting for more than 20 % of total bacteria( Reference Mach, Berri and Estelle 175 , Reference Kim, Borewicz and White 211 ). In adult humans, the phylum Actinobacteria may represent up to 15 % of total bacteria. It comprises bifidobacteria, the most predominant group detected in infants (40 % in average in faecal samples of 6-week-old European infants)( Reference Fallani, Young and Scott 212 ). The population of bifidobacteria present in the intestine of pigs is considerably lower, with less than 0·1 % of total sequences in the faecal samples of 22-week-old commercial pigs( Reference Kim, Borewicz and White 211 ). Dietary, environmental and behavioural (such as feeding habits) factors contribute to the species-specificity of the composition of microbiota.
Epigenetic mechanisms
The concept that early developmental dietary insults (poor or inadequate pre- or postnatal nutrition, for example) can have long-term consequences on health later in life has been termed developmental programming, or ‘developmental origins of health and disease’. The GIT microbiota appears to have an important role in the GIT programming as its initial composition creates distinct individuality during the lifespan( Reference Weng and Walker 167 , Reference Palmer, Bik and DiGiulio 213 , Reference Jakobsson, Jernberg and Andersson 214 ). A mechanism leading to these long-term effects may be due to epigenetically active fermentation metabolites such as in histone acetylation( Reference Strasak, Bartova and Harnicarova 215 , Reference Mischke and Plosch 216 ). However, no studies on epigenetic modifications underlying long-term effects on early microbial colonisation and gut function are available in pigs. Despite limited studies, use of the pig as a model for humans to assess the effects of early nutrition on the development of microbiota is gaining acceptance amongst the scientific community.
Conclusion
Although similarities exist between humans and pigs in terms of dynamics of postnatal maturation of microbiota diversity and structure, responses to environmental factors, including dietary factors, and phyla composition, differences in the most abundant genera exist. As reviewed by Heinritz et al. ( Reference Heinritz, Mosenthin and Weiss 180 ), understanding the crucial role and complexity of human microbiota could be improved by the use of human flora associations in pigs. This model has been successfully established taking advantage of the higher similarity between pigs and humans compared with the widely studied rodent models. In addition to the similarities in anatomy, physiology and metabolism between the pig and humans, the pig is more similar to humans than rodents with increased Bacteroides spp. and bifidibacteria( Reference Pang, Hua and Yang 217 , Reference Che, Pang and Hua 218 ). The pig has already successfully been used as a model for humans to study nutritional interventions( Reference Shen, Zhang and Wei 219 , Reference Wen, Tin and Wang 220 ). In addition, the human flora-associated pig can be considered as a useful model for human infants. However, stability of the implanted human microbiota in the gut of pigs during the lifespan remains to be investigated in studies regarding developmental programming.
The relationship between nutrition and the brain in the pig
Current outline on research in nutrition and neurosciences in pigs
Nutrient intake is driven by homeostatic and hedonic signals of peripheral, gastrointestinal, endocrinological and metabolic origin that convey in the central nervous system where they are integrated in a cognitive process referred to as the hunger–satiety cycle. Dietary nutrients also have an impact on brain development and function. Neuroscientific studies in pigs have progressed in recent years, partially to address scientific matters that cannot be studied in humans for ethical reasons.
This section summarises the pig studies, mostly in vivo and the minimally invasive neurocognitive explorations that are paralleled in human studies. Second, we will summarise the pig studies, mostly post-mortem explorations on brain tissues, which present differences or no equivalent in humans.
Similarities between pigs and humans in terms of brain functions
Brain responses to food signals
Describing brain responses to food signals is important to investigate food pleasure and motivation, or to decipher the brain networks underlying sensory and nutrient perception. An extensive literature is available on this topic in the human, describing mostly via functional MRI the brain responses to various food signals, according to different internal states (for example, hungry v. sated) or conditions (for example, lean v. obese), and many review papers are available on this topic (for example, Rolls( Reference Rolls 221 ); Stice et al. ( Reference Stice, Spoor and Ng 222 ); Carnell et al. ( Reference Carnell, Gibson and Benson 223 )). Studies using large animal models have not yet completely made use of the opportunities provided by in vivo brain imaging. The very first studies using functional imaging to describe food-induced brain responses in pigs used 99mTc-HMPAO (technetium hexamethylpropyleneamine oxime) SPECT (single photon emission computed tomography) and [18F]fluorodeoxyglucose positron emission tomography to map cerebral blood flow( Reference Boubaker, Val-Laillet and Guerin 224 – Reference Gaultier, Meunier-Salaun and Malbert 226 ) and brain GLU metabolism( Reference Clouard, Jouhanneau and Meunier-Salaun 227 ), respectively. These studies addressed the use of the pig to study food conditioning, looking at specific modulations of the response of the brain reward circuit after exposure to flavours with positive or negative hedonic values( Reference Gaultier, Meunier-Salaun and Malbert 226 , Reference Clouard, Jouhanneau and Meunier-Salaun 227 ). These studies provide two major outcomes: (1) functional imaging in anaesthetised pigs can be implemented to explore brain responses to different food signals, as in humans; and (2) the brain circuits activated by the perception of food signals are similar to those described in the human (for example, frontostriatal areas, amygdala and insular cortex). Boubaker et al. ( Reference Boubaker, Val-Laillet and Guerin 224 ) showed in pigs that duodenal and portal GLU infusions led to different systemic and brain responses in areas regulating food intake and pleasure. Clouard et al. ( Reference Clouard, Meunier-Salaün and Meurice 225 ) compared congruent v. dissociated oral and duodenal SUC perception, and found different brain responses in the limbic and reward circuits. Studies in human subjects showed that brain responses to energy-providing sugars and sweeteners are not the same in the reward circuit (for reviews, see Low et al. ( Reference Low, Lacy and Keast 228 ) and Ochoa et al. ( Reference Ochoa, Lallès and Malbert 229 )), which resembles the results obtained by Clouard et al. ( Reference Clouard, Meunier-Salaün and Meurice 225 ) in pigs. These studies are important to understand how the human brain correlates sugar cravings, as well as the neurobehavioural changes that could emerge due to the chronic consumption of, for example; sugars or non-energy sweeteners.
Impact of diet on brain activity, neurotransmission and cognition
Minipigs have become a widely accepted model for studying obesity and the metabolic syndrome( Reference Johansen, Hansen and Richelsen 230 – Reference Val-Laillet, Guerin and Malbert 234 ). They can be used to investigate the obesity-induced central modifications in humans, including decreased activity of the prefrontal cortex( Reference Le, Pannacciulli and Chen 235 – Reference Volkow, Wang and Telang 237 ) and altered dopaminergic function( Reference Volkow, Wang and Telang 237 , Reference Wang, Volkow and Logan 238 ). Val-Laillet et al. ( Reference Val-Laillet, Layec and Guerin 239 ) demonstrated in the Göttingen minipig that brain alterations similar to those described in obese humans exist in this model and that they are an acquired feature of obesity correlated to weight gain. In Pitman–Moore minipigs, Val-Laillet et al. ( Reference Val-Laillet, Meurice and Lalles 240 ) also described the effects of three high-lipid diets differing in their lipid sources and found that the basal GLU metabolism of the anterior prefrontal cortex and nucleus accumbens was highest with a diet enriched with sunflower-seed oil, intermediary with a diet enriched with lard, and lowest with a diet enriched with fish oil. These results demonstrate that specific dietary nutrients can modify brain metabolism independently from body weight, and that specific nutrients in excess might favour the onset of brain metabolism anomalies.
In humans, cognitive test scores were positively related to breast milk DHA and negatively related to linoleic acid, suggesting that high levels of dietary linoleic acid may impair cognition( Reference Lassek and Gaulin 241 ). Individual consumption of dietary FA had an impact on cognitive measures in children( Reference Lassek and Gaulin 242 ), with n-3 FA being positively related to cognitive test scores in male and female children, while n-6 showed the reverse relationship. Higher scores in tests of neurodevelopment were found in infants fed formula with DHA than in infants fed formulas without DHA( Reference Willatts, Forsyth and DiModugno 243 ). DHA supplementation in young boys increased the prefrontal cortex activation during sustained attention( Reference McNamara, Able and Jandacek 244 ). These results are consistent with the hypothesis that dietary DHA is assimilated by the brain and has a positive influence on cognition. Autopsy data show lower DHA in brain of human infants fed formula rather than those who were breast-fed( Reference Farquharson, Cockburn and Patrick 245 , Reference Makrides, Neumann and Byard 246 ), which resembles the results obtained in pigs (Table 4).
In pigs, dietary FA significantly made an impact on the frontal cortex and striatum concentrations of neurotransmitters (for example, dopamine, serotonin)( Reference de la Presa Owens and Innis 247 , Reference de la Presa Owens and Innis 248 ). Another study showed fewer arm-entries in a maze in pigs receiving a low-PUFA diet compared with a high-PUFA diet, with the effect being probably dependent on central dopamine metabolism( Reference Ng and Innis 249 ). Epidemiological and clinical studies also suggest a relationship between dietary FA and altered functions of the nervous system, including neurocognitive disorders( Reference Grosso, Galvano and Marventano 250 ). Dietary n-3 FA could also protect against brain disorders on neurotransmission, neuroprotection and neurogenesis( Reference Denis, Potier and Heberden 251 ). However, data are lacking for pigs providing an opportunity to investigate the relationship between diet, brain activity and cognitive functions relevant to humans via in vivo imaging.
Peripheral neuromodulation to regulate eating behaviour in pigs
Vagal nerve stimulation (VNS) is a therapy for refractory epilepsy and psychiatric disorders( Reference Hotujac and Kuzman 252 , Reference Vonck, De Herdt and Boon 253 ), but it has also received attention as a way to modulate food intake. Animal models, including pigs, were used to investigate this question( Reference Val-Laillet, Biraben and Randuineau 254 ). Diaz-Guemes et al. ( Reference Diaz-Guemes, Sanchez and Luis 255 ) found that VNS increased central nervous activity, but no effect was observed on feeding behaviour. In contrast, other authors demonstrated a decreased weight gain, decreased fat gain and plasma insulin-like growth factor I( Reference Sobocki, Krolczyk and Herman 256 ), or decreased food intake and specific activations in several brain areas associated with altered gastric myoelectric activity( Reference Matyja, Thor and Sobocki 257 ) in growing pigs( Reference Biraben, Guérin and Bobillier 258 ). Another on-going study( Reference Malbert, Guérin and Bobillier 259 ) showed VNS-induced metabolism differences in the brain reward circuit only 7d after VNS onset, meaning that quick central neuroplasticity phenomena can be induced by VNS, possibly modulating homeostatic and cognitive processes. In Göttingen minipigs fed a Western diet, VNS prevented further weight gain, decreased food intake and sweet cravings( Reference Val-Laillet, Biraben and Randuineau 254 ), proving the therapeutic potential of this strategy. Similarly, some studies assessing the impact of VNS on eating behaviours and weight in individuals with other psychiatric and neurological disorders showed significant modulation of food cravings and body weight( Reference McClelland, Bozhilova and Campbell 260 , Reference Val-Laillet, Aarts and Weber 261 ), but there are significant discrepancies between studies. Overall, functional imaging in pigs has the potential to help validate and optimise therapies before their application to human patients.
Central neuromodulation to regulate eating behaviour in pigs
Recent development suggests that the deep-brain stimulation (DBS), a procedure for the treatment of Parkinson’s or depression, might also be used to combat obesity( Reference Halpern, Wolf and Bale 262 – Reference Howland 265 ). The minipig has emerged as an ideal model for basic and preclinical studies on DBS( Reference Sorensen, Nielsen and Rosendal 266 ). Hypothalamic DBS was validated in the Göttingen minipig( Reference Bjarkam, Nielsen and Glud 267 , Reference Ettrup, Tornoe and Sorensen 268 ) and resulted in reduced weight gain( Reference Melega, Lacan and Gorgulho 269 ), as well as in behavioural and physiological changes that could be related to the activation of limbic and autonomic brain networks( Reference Ettrup, Sorensen and Rodell 270 ). These results with pigs are similar to those described in human studies (for reviewes, see McClelland et al. ( Reference McClelland, Bozhilova and Campbell 260 ) and Val-Laillet et al. ( Reference Val-Laillet, Aarts and Weber 261 )). Shon et al. ( Reference Shon, Lee and Goerss 271 ) showed that DBS of the subthalamic nuclei in pigs can stimulate striatal dopamine release, which is related to food motivation and obesity( Reference Volkow, Wang and Baler 272 , Reference Narayanaswami, Thompson and Cassis 273 ), whereas Sauleau et al. ( Reference Sauleau, Lapouble and Val-Laillet 12 ) managed to modify food motivation and learning. Knight et al. ( Reference Knight, Min and Hwang 274 ) demonstrated that DBS of the nucleus accumbens, a putative target to combat obesity( Reference Halpern, Wolf and Bale 262 ), modulated the activity of the prefrontal, cingulate and insular cortices, which are brain regions involved in eating behaviour. A similarly low metabolic activity of the prefrontal cortex was observed in obese humans( Reference Le, Pannacciulli and Chen 235 – Reference Volkow, Wang and Telang 237 ) and minipigs( Reference Val-Laillet, Layec and Guerin 239 ), an anomaly that was normalised via DBS of the cortex. The combination of DBS and MRI has been explored in pigs, in terms of image-guided brain navigation( Reference White, Woolley and Bienemann 275 ), network activation( Reference Knight, Min and Hwang 274 , Reference Min, Hwang and Marsh 276 ) and safety( Reference Shrivastava, Abosch and Hanson 277 – Reference Gorny, Presti and Goerss 279 ). These studies show the (mini) pig is a convenient model to study the impact of DBS on eating behaviour and nutritional diseases, and to test medical innovations in preclinical trials before being safely applied to humans.
New imaging approaches in pigs
In addition to the aforementioned neuromodulation studies, there are many innovative methods related to nutrition and brain activity used in humans that could potentially be investigated in pigs. Alstrup & Smith( Reference Alstrup and Smith 280 ) reviewed 10 years of positron emission tomography findings on neuromolecular processes in the living porcine brain and listed all the validated brain radio ligands including several molecules of interest for nutrition studies. Other methods can be used for molecular imaging in pigs, such as the wireless instantaneous neurotransmitter concentration system which allows the measure of neurotransmitter release in specific brain areas( Reference Agnesi, Tye and Bledsoe 281 , Reference Van Gompel, Chang and Goerss 282 ). The non-invasive magnetoencephalography and electrocorticography( Reference Bowyer, Okada and Papuashvili 283 ), as well as the functional near-IR spectroscopy and cortical imaging have been successfully implemented in the pig model( Reference Uga, Saito and Sano 284 ) and could be applied in the future to map brain responses to food and nutrient stimulations.
Differences between pigs and humans, or pig studies with no equivalent in humans
The most important corpus of literature investigating the impact of nutrition on the pig brain has been focused on brain composition and development, and especially on the role of dietary FA. The health consequences of dietary deficits or supplements of n-3 and n-6 FA are still controversial areas of human nutrition due to conflicting results. However, animal models such as the pig have the potential to bring new insight through better-controlled experimental designs( Reference Innis 285 , Reference Innis 286 ). Table 4 provides a comprehensive summary of this literature. Most of these results have no equivalent in human studies (other than exceptional post-mortem case studies), because it is not possible to assess, non-invasively, human brain composition or the administration of specific toxicants.
The BBB is important for the regulation of food intake and the blood-to-brain transport of dietary compounds( Reference Banks 287 ). The passage of xenobiotics( Reference Milbury and Kalt 288 ) or bismuth( Reference Pollet, Albouz and Le Saux 289 ) into the brain has been investigated in pigs via post-mortem tissue analyses. However, the emergence of in vitro models of pig BBB( Reference Goti, Balazs and Panzenboeck 290 – Reference Patabendige, Skinner and Morgan 293 ) have significant advantages for investigating the transport mechanisms of compounds into the brain. BBB transport of glutamate( Reference Kim, Virella and Braunberg 294 ), alkaloids( Reference Mulac, Huwel and Galla 295 ), mycotoxins( Reference Weidner, Huwel and Ebert 296 ) and central nervous system-active drugs( Reference Campbell, Regina and Kharasch 297 ) was assessed with this model, which might help to understand the neural toxicology of dietary compounds and the effectiveness of medicines. An in vivo imaging study in obese minipigs showed increased BBB permeability with a diet enriched with fish oil characterised by an excessive amount of n-3 FA( Reference Val-Laillet, Meurice and Lalles 240 ). Transport of nutrients and their impact on the BBB integrity should receive more attention in the future to understand how the gut–brain axis is altered by nutritional diseases( Reference Buckman, Thompson and Moreno 298 ), and to provide nutritional recommendations in humans. If the increased BBB permeability induced by high doses of n-3 FA is confirmed in humans, this could also have unexpected beneficial outcomes, for example to improve drug delivery to the brain in Alzheimer’s disease( Reference Banks 299 ) or other neuropsychiatric disorders.
In pigs, central concentration of serotonin can modulate operant food intake behaviour( Reference Ebenezer, Vellucci and Parrott 85 ). In addition, brain DHA, which depends on dietary DHA, promotes central dopamine metabolism( Reference Ng and Innis 249 ). Dietary AA can also affect brain neurotransmitters in the hypothalamus( Reference Adeola and Ball 300 – Reference Shen, Voilque and Kim 303 ), suggesting that dietary manipulation of AA precursors of neurotransmitters may offer a practical means of reducing stress responses. Further studies are needed to verify whether these results may be of benefit in human nutrition, for example to help patients with stress disorders. Elmquist et al. ( Reference Elmquist, Ross and Hsu 304 ) suggested that central CCK increases with time in piglets in parallel to the ability to assimilate nutrients from a solid diet. Kenk et al. ( Reference Kenk, Thomas and Lortie 305 ) demonstrated via positron emission tomography imaging of cAMP, a strong region-specific signal in the brain, as well as an impaired cAMP-mediated signalling in obese pigs, giving some insight into pathological progression with potential for directing therapy in humans. Mycotoxin( Reference Prelusky 306 , Reference Swamy, Smith and Karrow 307 ) and feed additives( Reference Poletto, Cheng and Meisel 308 ) were also found to alter behaviour, neurotransmitter activity and metabolism in pigs. The decreased feed intake and increased aggressive behaviour observed in subjects contaminated with mycotoxins might be related to anomalies in brain monoamines, including dopamine and serotonin. Gbore( Reference Gbore 309 ) demonstrated that the acetylcholinesterase activity in the hypophyses, hypothalamus and amygdala decreased with increased mycotoxin concentrations in the diet. These results are important to identify the risk associated with mycotoxin contamination and possible therapeutic interventions in humans.
Pig studies exploring the brain responses to specific diets relying on post-mortem methods have no equivalent human data. Kanitz et al. ( Reference Kanitz, Otten and Tuchscherer 310 ) showed that low protein:carbohydrate dietary ratio during gestation may alter the brain expression of genes encoding key determinants of glucocorticoid activity in the fetus, with potential long-lasting consequences for stress adaptation and health. Also, increased c-Fos (a transcription factor) immunoreactivity in several brain structures was described after oral administration of fungi extracts in pigs( Reference Gaige, Bonnet and Tardivel 311 ). Madsen et al. ( Reference Madsen, Birck and Fredholm 312 ) found that expression of the fat mass and obesity associated gene (FTO) transcript was detected at high levels in brain tissues and that these levels varied through the development and between specific brain areas. These results demonstrate a relationship between the genetic propensity to develop obesity and dietary habits at the cerebral level. Since the FTO gene has recently been associated with increased BMI in several human populations, the pig model might be used to investigate the epigenetic mechanisms that could lead to obesity-related brain anomalies in humans.
Conclusions on the relationship between nutrition and the brain
The general comparison between the brain of pigs and humans (Table 1) shows that, even if there are some differences in terms of size and structure, the overall brain anatomy and development in pigs are similar to those of humans. In addition, similar functional neuroimaging approaches have been transferred from humans to pigs. Understandably, many pig studies do not have any equivalent in humans (especially the invasive and terminal experiments). However, most of the in vivo functional brain explorations and therapies described in pig models are echoed in human studies, which highlight the fantastic potential of pig models for translational research in nutrition and neurosciences. In addition, the pig model is of high value to perform mechanistic, toxicological and epigenetics studies that could not be performed in humans for practical and ethical reasons.
Acknowledgements
The authors would like to thank Dr John L. Black and Dr Anton Pluschke for reviewing the manuscript and for their sound comments.
There are no conflicts of interest.