Introduction
Cardiovascular diseases (CVD) are a major cause of mortality and disability in the UK (Reference Bhatnagar, Wickramasinghe and Williams1). Since diet and low-density lipoprotein (LDL)-cholesterol (LDL-C) are both important modifiable CVD risk markers, there is considerable interest in dietary strategies which can reduce LDL-C levels for disease prevention (Reference Lewington, Whitlock and Clarke2). Probiotic, prebiotic and polyphenol-rich interventions have reported beneficial effects on the fasting lipid profile, with changes in the gut microbiota composition thought to play an important role in lipid regulation. Emerging evidence suggests that bile acids may act as a link between changes in the gut microbiota and cardiovascular health, via effects on circulating lipids, inflammation and glycaemia, with the profile and concentration of circulating bile acids considered to be a potential novel biomarker of disease risk (Reference Long, Gahan and Joyce3).
Bile acids are synthesised in the liver from cholesterol and form the major component of bile (Reference Long, Gahan and Joyce3). They are stored in the gallbladder before being released into the gut in response to food intake, where they emulsify dietary fats to facilitate adsorption of lipids and lipid-soluble vitamins (Reference Bauer, Jakob and Mosenthin4). Bile acids are significantly modified in the gut by bacterial enzymes (Reference Joyce and Gahan5). Bile salt hydrolase (BSH), bile acid inducible (BAI) and bile acid dehydratase enzymes, expressed by certain species of gut bacteria can modify bile acids to generate unconjugated and secondary bile acids (Reference Joyce, MacSharry and Casey6). These converted bile acids may be less well absorbed in the intestine, and as such, the proportion of bile acids being excreted may be affected by the microbial population in the gut (Reference Begley, Hill and Gahan7). The vast majority (95 %) of bile acids are recycled back to the liver via the hepatic portal circulation in what is known as the enterohepatic cycle (Reference Chiang8). However, a small percentage will escape recycling and are excreted in the faeces. Bile acid neo-synthesis is an effective system for cholesterol regulation in the body, taking cholesterol out of the circulation to be used for synthesis of new bile acids, replacing those lost in the faeces. Certain foods including probiotics, prebiotics and those rich in polyphenols have been shown to alter the gut microbiome, enhancing growth of probiotic bacteria with bile acid metabolising activity. Since high circulating LDL-C levels are a significant risk factor for CVD (Reference Grover, Dorais and Coupal9), the ability of probiotic bacteria to reduce cholesterol via bile acid modification in the gut could be key for dietary interventions in reducing disease risk and improving population health. This narrative review will provide an overview of bile acid metabolism and determine the role of probiotics, prebiotics and polyphenol-rich foods in modulating circulating bile acids and lipid risk markers for CVD.
Bile acids and modulation of CVD risk markers
Bile acids are synthesised through two distinct pathways, the classical and alternative pathways (see Fig. 1 for an overview). For an in-depth discussion on the synthesis of bile acids, the reader is directed to an excellent review by Li and Chiang(Reference Li and Chiang10). Within the liver, classic bile acid synthesis is initiated in the hepatocytes via cholesterol hydroxylation, catalysed by the cholesterol 7α-hydroxylase (CYP7A1) enzyme, part of the cytochrome P450 enzyme family (Reference Staels and Fonseca11). This is the rate-limiting step in bile acid synthesis and leads to conversion of cholesterol to 7α-hydroxycholesterol. CYP7A1 expression is regulated by farnesoid X receptor (FXR), via a feedback mechanism, by an increase in bile acids following food intake. FXR can be directly activated in hepatocytes or by bile acids in the gastro-intestinal (GI) tract. In the intestine, FXR activation leads to expression of fibroblast growth factor (FGF) 19, which travels to the liver to activate FGF receptor 4 (FGFR4) and ultimately suppresses expression of CYP7A1, inhibiting bile acid synthesis (Reference Li and Chiang10). The alternative bile acid pathway occurs in the mitochondria and produces mainly chenodeoxycholic acid, and can therefore theoretically occur in all cell/tissue types. Evidence of alternative bile acid synthesis has been shown to occur in the brain, macrophages and liver (Reference Ogundare, Theofilopoulos and Lockhart12–Reference Chiang14). In this pathway, hydroxylation of cholesterol leads to its conversion to oxysterols catalysed by sterol 27-hydroxylase. 7α-hydroxylated oxysterols are eventually converted to primary bile acids in enzymatic pathways common to both the classic and alternate bile acid pathways (Fig. 1) (Reference Li and Chiang15). In the final step of bile acid synthesis, bile acids are conjugated to an amino acid, either glycine or taurine, via formation of a thioester intermediate, which is catalysed by the bile acid cholyl-CoA synthetase and bile acid CoA:amino acid N-acyltransferase enzymes (Reference Falany, Johnson and Barnes16,Reference Pircher, Kitto and Petrowski17) . Conjugated bile acids, known as bile salts, have greater water solubility and are secreted into bile which is stored in the gallbladder. The hormone cholecystokinin is the main regulator of gallbladder contraction and sphincter of Oddi relaxation which facilitates the release of bile through the bile duct and duodenal papilla to the duodenum during the postprandial phase (Reference Portincasa, Di Ciaula and Wang18,Reference Russell19) . Following food intake, nutrients, including fatty acids and some amino acids, are sensed in the small intestine (the duodenum), resulting in secretion of cholecystokinin by enteroendocrine cells and promoting absorption in the intestine (Reference Costarelli and Sanders20).

Fig. 1. Metabolism of bile acids: bile acid synthesis occurring in the liver from cholesterol via host cytochrome P450 enzymes, through the classic and alternative bile acid synthetic pathways. Conjugation of bile acids occurs in the liver, with further modification of BA moieties by microbes in the GI tract which can de-conjugate using bile salt hydrolases (BSH). This results in free primary bile acids which can be further modified by microbes for example dehydroxylation via bile acid-inducible (BAI) enzymes to yield a variety of secondary bile acids (such as deoxycholic and lithocholic acid). Reprinted from Molecular Aspects of Medicine, 56, Sarah L. Long, Cormac G.M. Gahan, Susan A. Joyce, Interactions between gut bacteria and bile in health and disease, Pages No. 54–65, Copyright (2017), with permission from Elsevier.
Sterol regulatory element-binding proteins (SREBP) belong to a family of basic helix-loop-helix leucine-zipper transcription factors which are regulated by intracellular levels of cholesterol/oxysterols and are vital in the regulation of fatty acid and cholesterol synthesis (Reference Sato21). SREBP-1c (produced from the SREBF-1 gene) is mainly involved in fatty acid synthesis, while SREBP-2 (encoded by the SREBF-2 gene) is implicated in the synthesis of cholesterol (Reference Li and Chiang10,Reference Janowski, Shan and Russell22) . SREBP-1c expression is induced by insulin as well as activation of liver X receptor (LXR)-α by oxysterols (Reference Eberlé, Hegarty and Bossard23) required to generate fatty acids for the synthesis of cholesterol esters (Reference Tontonoz and Mangelsdorf24). Transcription factors for SREBP2 are preserved as an inactive precursor in the endoplasmic reticulum membranes when oxysterol levels are high, for example during synthesis of bile acids. However, conversion of intracellular oxysterols to primary bile acids leads to transportation of the SREBP2 precursor to the Golgi apparatus where it is cleaved by proteases to release the amino-terminal portion of the protein from its membrane-bound precursor. This protein then migrates to the nucleus where it can activate the transcription of the LDL-receptor gene, increasing the expression of receptors on the surface of the hepatocytes and leading to an increase in intracellular cholesterol levels (Reference Janowski, Shan and Russell22). Interestingly, studies in mice have shown that overexpression of CYP7A1 leads to increased hepatic expression of SREBP2, suggesting a link between bile acid synthesis and hepatic cholesterol regulation (Reference Miyake, Doung and Strauss25).
Enterohepatic circulation allows for recycling of bile acids and other substances that are absorbed from the intestine, packaged as lipid micelles, and transported back to the liver via portal blood circulation (see Fig. 2 for an overview) (Reference Chiang8). In addition, some free hydrophobic bile acids are reabsorbed by passive diffusion in the GI tract. Once in the liver they are secreted into the bile and subsequently re-enter the intestine. A large proportion (95 %) of bile acids are recycled in this way, with the remaining 5 % being excreted in faeces (Reference Joyce and Gahan5). Enterohepatic recycling is important to preserve the bile acid pool which is vital for many functions of the liver and GI tract such as bile flow, solubilisation and excretion of cholesterol as well as intestinal absorption of lipophilic compounds (Reference Mertens, Kalsbeek and Soeters26). In addition, this process conserves cholesterol and bile acids for re-use and avoids the loss of these valuable sterols which would otherwise need to be endogenously synthesised or obtained from the diet.

Fig. 2. Enterohepatic circulation of cholesterol and bile acids: between 0·2 and 0·6 g bile acid is synthesised per day in the liver to maintain the human bile acid pool which is made up of approximately 3 g of bile acids. Absorption of nutrients following food intake results in stimulation of the gallbladder, which releases bile acids into the small intestine. In the ileum, conjugated bile acids can be easily reabsorbed by active transport, while a small amount of unconjugated bile acids are reabsorbed by passive diffusion in the small and large intestines. Bile acids are then extracted from the portal blood by the liver. Small amounts of bile acids are excreted in the faeces (approximately 5 %) and must be replaced by neo-synthesis in the liver.
The microbiota residing in the GI tract can influence the proportions of primary and secondary bile acids (Reference Staley, Weingarden and Khoruts27). This is because several species of gut bacteria, including Clostridium, Enterococcus, Bifidobacterium, Lactobacillus and members of the genus Bacteroides, are capable of converting primary bile acids into secondary bile acids, such as lithocholic acid (LCA) and deoxycholic acid (DCA), via 7α-dehydroxylation (BAI encoded) enzymes and further conversion into unconjugated forms, in the distal ileum by BSH enzymes (Table 1) (Reference Ridlon, Harris and Bhowmik28). In the large intestine, bile acids undergo bacterial bio-transformations including de-conjugation followed by further metabolism such as oxidation of hydroxyl groups and dihydroxylation (Reference Lefebvre, Cariou and Lien29). Secondary bile acids, especially the more hydrophobic ones, are less well absorbed in the enterohepatic cycle, and therefore a greater amount may be excreted in the faeces, leading to a net loss of cholesterol (Reference Long, Gahan and Joyce3). This is because bacterial de-conjugation results in more hydrophobic bile acids which are poorly absorbed via passive diffusion. Table 1 presents the relative hydrophobicity of the main bile acids. LCA is commonly cited as the most hydrophobic bile acid (Reference Hanafi, Mohamed and Sheikh Abdul Kadir30); however, the hydrophobicity index for un-conjugated LCA could not be found in the literature. Meanwhile, conjugated bile acids can be more easily taken up by ileal bile acid transporters/apical bile acid transporters (IBAT/ABAT) transporters (Reference Begley, Hill and Gahan7). The secondary bile acids constitute up to 35 % of the circulating bile acids and have important regulatory functions in metabolic processes including weight maintenance and glucose/lipid tolerance (Reference Wahlström, Sayin and Marschall31). As such, the gut microbiota plays an important role in the enterohepatic circulation and cholesterol regulation.
Table 1. Circulating bile acids and their signalling potential

Abbreviations: FXR, farnesoid X receptor; LXRα, liver X receptor α; PXR, pregnane X receptor; TGR5, G-protein-coupled bile acid receptor 1; VDR, vitamin D receptor.
Adapted from circulating bile acid concentrations measured in fasting plasma using LC-MS/MS in healthy adults between the ages of 18 and 45 years (Reference Ginos, Navarro and Schwarz166). Hydrophobicity index (HI) quantitatively defines the merged hydrophilic–hydrophobic balance of bile salts, based on bile salt capacity factor logarithms calculated using reversed-phase high-performance liquid chromatography (HPLC) and standardised arbitrarily to the indices of taurocholate (HI 0) and taurolithocholate (HI 1) (Reference Heuman, Hylemon and Vlahcevic157).
A mechanistic link between gut microbiota composition and host physiology is thought to occur via the microbiologically produced secondary bile acids. These act as key regulators in several metabolic processes since they are considered to be stronger agonists for several receptors involved in host metabolism compared with primary bile acids (Reference Li and Chiang32). Bile acids activate specific nuclear receptors including FXR, vitamin D receptor (VDR), pregnane X receptor (PXR) and the G-protein-coupled receptor TGR5 which are implicated in cell signalling pathways in both the liver and the GI tract (see Table 1 for signalling potential of common bile acids) (Reference Hylemon, Zhou and Pandak33). FXR activation has been linked to the maintenance of normal triacylglycerol (TAG) and cholesterol levels, as well as regulating factors in the liver and intestine which influence CVD risk such as lipid and glucose homeostasis, endothelial function and atherosclerosis (Reference Cariou and Staels34). Meanwhile, the secondary bile acids LCA and DCA act as powerful ligands for TGR5 (Reference Chen, Lou and Meng35). This influences several important metabolic pathways, such as thermogenesis, energy metabolism and glucose homeostasis. Activation of TGR5 leads to increased intestinal production of the gut hormone glucagon-like peptide (GLP)-1 promoting insulin secretion and regulation of appetite, and increased energy expenditure via conversion of thyroid hormone T4 into the active form, T3 (Reference Watanabe, Houten and Mataki36,Reference Thomas, Gioiello and Noriega37) .
The profile of secondary bile acids, both in the GI tract and systemically, may differ in conjunction with improved and diminished host health. For instance, in animal models, increased circulating levels of taurocholate have been associated with greater proliferation of the gut bacteria Bilophila wadsworthia, which are related to inflammatory bowel disease (Reference Devkota, Wang and Musch38). Higher serum concentrations of DCA, a secondary bile acid produced via colonic microbial transformation of cholic acid, have been found in obese mice, likely due to dysbiosis of the gut microbiota such as a proliferation of clostridium species (Reference Yoshimoto, Loo and Atarashi39). Meanwhile levels of other secondary bile acids may be linked with beneficial effects on host health. For example, increased levels of ursodeoxycholic acid (UDCA) and its glycine and taurine conjugates in bariatric surgery patients have been demonstrated alongside increased insulin sensitivity (Reference Albaugh, Flynn and Cai40). Furthermore, a study in rats by Basso et al. (Reference Basso, Soricelli and Castagneto-Gissey41) found higher levels of UDCA after partial gastrectomy which occurred in conjunction to enhanced insulin sensitivity and fat distribution, independent of weight loss (Reference Basso, Soricelli and Castagneto-Gissey41). Increased levels of circulating bile acids are also found in Roux-en-Y gastric bypass patients because, when the duodenum and proximal jejunum are bypassed, bile acids do not mix with food until they reach the mutual portion of the jejunum (Reference Fouladi, Mitchell and Wonderlich42). This means that greater concentrations of bile acids are delivered to the jejunum, and their uptake by IBAT/ABAT transporters is increased in the ileum. As a consequence, higher concentrations of bile acids occur in the portal vein, and more bile acids are released into the systemic circulation. Furthermore, alterations of the gut microbiota are known to occur following bariatric surgery, with a tendency toward reduced Firmicutes-to-Bacteroides ratio and an increase in Proteobacter, which can alter the proportions of secondary bile acids in the intestinal bile acid pool through increased BSH activity (Reference Li and Chiang32).
Systemic effects of secondary bile acids on host health have also been demonstrated by direct consumption or intrajejunal administering of specific secondary bile acids. For example, intake of UDCA given as granules, in healthy human subjects has been linked with improved postprandial blood glucose levels and raised GLP-1 secretion. This gut hormone controls glucose-induced insulin excretion and gastric emptying (Reference Murakami, Une and Nishizawa43). Furthermore, consumption of UDCA in rats has been shown to reduce fructose-induced metabolic syndrome, demonstrated by the addition of 150 mg/kg UDCA to drinking-water (Reference Mahmoud and Elshazly44). Moreover, in humans, intrajejunal taurocholic acid has been reported to decrease blood glucose and activate release of satiety hormones such as GLP-1 and peptide YY (Reference Wu, Bound and Standfield45).
Bile acids are thought to regulate various receptors influencing the regulation of metabolism and lipid profiles. Various secondary bile acids act as strong or weak agonists of FXR and TGR5, while some can suppress their activity, for example tauro-β-muricholic acid, an FXR antagonist (Reference Sayin, Wahlström and Felin46). Therefore, the profile of circulating bile acid (BA) in contact with these receptors in different tissues may determine their level of regulation. Activation of such nuclear receptors may provide the key to linking the microbiota composition and activity with cardiovascular health via the bile acid converting activity of certain strains of gut bacteria. In light of this, circulating bile acids may represent useful biomarkers of cardiovascular health in humans.
The influence of prebiotics, probiotics and polyphenol-rich foods on bile acids, gut microbiota and CVD risk
An important contributor to the balance of the gut microbiota and, thus, bile acid homeostasis is diet. Gut bacteria profiles can be changed by dietary factors, with population studies demonstrating higher consumption of fruits, vegetables and fibres to have beneficial effects attributed to components such as probiotics, prebiotics and polyphenols (Reference Joyce and Gahan47–Reference Arora, Singh and Sharma50). Functional BSH enzymes have been found in all of the main species of gut bacteria with potential to modify primary bile acids, converting them to secondary bile acids (Reference Jones, Begley and Hill51). Meanwhile very few species of gut bacteria have the BAI encoded enzymes required to perform bile acid 7α-dehydroxylation and further bile acid transformation. Bacteria with BSH activity may also be involved in control of metabolic pathways such as those involved in glucose and lipid metabolism, integrity of the gut, inflammation and the circadian rhythm via generation of unconjugated bile acids from tauro-conjugated bile acids which allows further metabolism of bile acids by the gut microbiota (Reference Joyce, MacSharry and Casey6). This has been demonstrated with gastro-intestinal expression of BSH enzymes in mice, which resulted in changes in plasma bile acid profiles as well as altered transcription of lipid metabolism genes including peroxisome proliferator-activated receptor γ, angiopoietin-like 4, and ATP-binding cassette sub-family G member 5/8 (involved in cholesterol metabolism) in the liver or small intestine. Indeed, increased expression of BSH in these mice led to reduced weight gain and lower levels of plasma cholesterol and liver triglycerides (Reference Joyce, MacSharry and Casey6). Therefore, any dietary component which has the ability to influence the proliferation of bacteria with BSH activity in the gut may also modulate bile acid homeostasis and the ability to impact on host cardiovascular health.
Fibres such as β-glucans, found in oats, and pectin, found in apples, and polyphenols such as proanthocyanidins, catechins or tannins, have been reported to possess bile acid sequestering activity (Reference Ikeda, Imasato and Sasaki52,Reference Ikeda, Yamahira and Kato53) . This can cause the bile acids to travel unabsorbed into the colon where they are excreted or transformed again into secondary bile acids by the colonic bacteria. Studies have shown that pharmaceutical sequestering agents may decrease circulating LDL-C, reduce obesity, improve insulin sensitivity and induce thermogenesis (Reference Watanabe, Morimoto and Houten54). Therefore, bile acid sequestering agents have potential for treatment of metabolic disease.
In view of the reported effects of prebiotics, probiotics and polyphenols on increasing the prevalence of gut bacteria with bile acid metabolising activity and bile acid sequestering activity, little is known about whether dietary modulation of circulating bile acids is associated with the beneficial effects reported on CVD risk markers. The latter part of this review will present the evidence from animal and human studies on the impact of prebiotics, probiotics and polyphenol-rich foods on bile acid metabolism as a potential mechanism for their positive effects on host lipid profiles and cardiovascular health. The studies presented have included measures of bile acids and modulation of the lipid profile and/or gut microbiota.
Gut dysbiosis
A deviation from the natural balance in the gut microbiota (dysbiosis) is associated with obesity and conditions such as inflammatory bowel disease (Reference Matsuoka and Kanai55). Reduced diversity and decreased levels of certain bacteria in the gut can lead to activation of the mucosal immune system, resulting in damage to the GI tract, which is particularly evident in inflammatory bowel disease (Reference Sokol, Lay and Seksik56). Dysbiosis may also result in alterations in bile acid metabolism which can further disrupt gut homeostasis via effects on bacterial deconjugation, transformation and desulphation of bile acids (Reference Duboc, Rajca and Rainteau57). This interruption of normal bile acid metabolism may result in increased intestinal epithelial inflammation and gut permeability, allowing translocation of bacterial lipopolysaccharides across the gut–blood barrier, which in turn results in systemic inflammation. A high-fat diet has been frequently associated with dysbiosis and bile acid dysregulation (Reference Zheng, Huang and Zhao58). This reflects the importance of bile acids as postprandial signalling molecules that can affect gut mucosal defences via both antibacterial and anti-inflammatory actions reducing the synthesis of pro-inflammatory cytokines (TNF-α, monocytes and macrophages) (Reference Sannasiddappa, Lund and Clarke59). In addition, decreased synthesis of secondary bile acids leads to reduced activation of nuclear receptors such as FXR, PXR, TGR5 and VDR. This can cause changes in bile acid synthesis and have a negative impact on lipid homeostasis (Reference Chen, Thomsen and Vitetta60). Dysbiosis and the resulting interruption to the intestinal epithelial barrier is therefore linked with several diseases, including non-alcoholic fatty liver disease and cardiometabolic diseases (Reference Carding, Verbeke and Vipond61). Consumption of probiotics, prebiotics and polyphenol-rich foods may restore the balance of gut microbiota, regulating bile acid metabolism and restoring nuclear receptor activation.
Probiotics
Probiotics are beneficial strains of bacteria with positive effects on host health. Both Bifidobacterium and Lactobacillus species have been identified as probiotics with BSH activity, with the highest BSH activity identified in Bifidobacterium breve and Lactobacillus plantarum LA3 strains (Reference Öner, Aslim and Aydaş62). BSH enzymes catalyse the first step in the conversion of conjugated primary bile acids into unconjugated species, allowing further transformation into secondary bile acid species, and may therefore lead to reduced reabsorption of bile acids in the enterohepatic cycle since secondary bile acids may be less easily absorbed. Certain secondary bile acids may be more readily excreted from the body, and bile acid neo-synthesis and LDL clearance may be enhanced (Reference Costabile, Buttarazzi and Kolida63,Reference Costabile, Klinder and Fava64) . LCA, for example, as the most hydrophobic bile acid, is reabsorbed poorly into the enterohepatic circulation, and thus, greater levels of LCA are excreted in the faeces (Reference Hanafi, Mohamed and Sheikh Abdul Kadir30).
Effects on lipid metabolism
Several studies using animal models have demonstrated reduced circulating LDL-C levels following probiotic supplementation (Reference Wa, Yin and Gu65–Reference Jeun, Kim and Cho71). Such results have also been replicated in human studies with the findings of a meta-analysis including 13 trials reporting a combined mean net change in total cholesterol (total participants n = 485) of −1·66 mM LDL-C as −1·27 mM and TAG as −0·45 mM (Reference Guo, Liu and Zhang72) in those individuals treated with probiotics versus controls. Jones et al. (Reference Jones, Martoni and Parent73) found that the consumption of Lactobacillus reuteri for 6 weeks reduced total and LDL-C by 8·9 % and 4·8 %, respectively, in healthy hypercholesteraemic men and women. The treatment group (n = 56) consumed 115 g of natural yoghurt and 10 g of microcapsules containing BSH-active L. reuteri (equivalent to 1·4 × 109 CFU), twice per day, compared with a placebo group (n = 58) given 125 g of natural yoghurt (Reference Jones, Martoni and Parent73). Similarly, Costabile et al. (Reference Costabile, Buttarazzi and Kolida63) observed a significant decrease in LDL-C (by 13·9 %) and a non-significant tendency for a reduction in total cholesterol (by 2 %) in healthy adults following 12 weeks of supplementation with Lactobacillus plantarum (2 × 109 CFU (0·1 g) twice daily) compared with a placebo (twice daily in the same capsular format) (Reference Costabile, Buttarazzi and Kolida63). Meanwhile, Martoni et al. (Reference Martoni, Labbé and Ganopolsky74) found reduced serum LDL-C in hypercholesteraemic adults (n = 10), compared with baseline concentrations, after 4 weeks of consuming a delayed-release probiotic capsule (Lactobacillus reuteri NCIMB 30242, 3–9 × 109 CFU twice daily) (Reference Martoni, Labbé and Ganopolsky74).
Effects on bile acids
Studies have been performed in animals and, to a lesser extent, in humans to provide further insights into the link between bile acid metabolism and lipid regulation in response to probiotic intake. A summary of studies which have investigated the effect of probiotic supplementation on bile acids in humans (n = 4) and animal models (n = 16) is presented in Table 2 and Supplementary Table 1, respectively. Several studies in animal models have found increased excretion of bile acids in stools following intervention with various probiotic bacteria strains (Reference Ma, Zhang and Lu66,Reference Guo, Li and Tang68,Reference Jeun, Kim and Cho71,Reference Zhai, Liu and Wang75–Reference Degirolamo, Rainaldi and Bovenga78) . However, in the limited number of human trials measuring faecal bile acid levels following chronic probiotic use, an effect on bile acid excretion has not been demonstrated (Reference Martoni, Labbé and Ganopolsky74,Reference Stadlbauer, Leber and Lemesch79) . Additionally, a study by Oshiro et al. (Reference Oshiro, Nagata and Wang80) on premature infants born between 24 and 31 weeks of gestation, found that supplementing parenteral nutrition with Bifidobacterium breve resulted in reduced faecal total bile acids compared with a placebo (Reference Oshiro, Nagata and Wang80). These discrepancies highlight the need for further human studies examining the tentative link between probiotic bacteria and bile acid/absorption/excretion.
Table 2. Summary of studies investigating the effect of polyphenols, prebiotics and probiotics on bile acids and lipid profiles/gut microbiota in humans

Abbreviations: BA, bile acid; BSH, bile salt hydrolysing; BW, body weight; CO, crossover; DB, double blind; HDL-C, high-density lipoprotein cholesterol; HyperChol, hypercholesteraemic; LDL-C, low-density lipoprotein cholesterol; MS, metabolic syndrome; O, obese; OW, overweight; PCT, placebo control trial; RCPI, randomised controlled prospective intervention; RCT, randomised control trial; RGC, randomised group comparison; SCFA, short-chain fatty acid; TC, total cholesterol; TAG, triacylglycerol; VOO, virgin olive oil.
Prebiotics
A dietary prebiotic is defined as ‘a substrate that is selectively utilised by host microorganisms conferring a health benefit’ (Reference Gibson, Hutkins and Sanders81). The most common prebiotics are carbohydrate substrates, and the majority of studies associated with prebiotics and bile acid profiles or cholesterol homeostasis have been performed using β-glucans, fructans, fructose polysaccharide inulin oligosaccharides or galacto-oligosaccharides (Reference Figueroa-González, Quijano and Ramírez82). Dietary prebiotics can alter the microbiota profile in the gut in a dose-dependent manner, and recent evidence, from a limited number of studies, suggests this may impact upon CVD risk markers. For example, a recent review of 16S r-RNA studies on prebiotics and the microbiome found that, generally, consumption of inulin-type fructans (n = 17 studies) in human studies resulted in increased abundance of Bifidobacterium but had little effect on other gut microbes (Reference Swanson, de Vos and Martens83). Meanwhile, supplementation with glucose-based fibres, such as types of resistant starch, had wider effects on the microbial community, especially abundance of Ruminococcus species. Galacto-oligosaccharides, xylo-oligosaccharides and arabinoxylan-oligosaccharides (n = 8 studies) generally led to increases in Bifidobacteria and only slight increases in relative abundance of other gut microbes (Reference Swanson, de Vos and Martens83).
Effects on lipid metabolism
Prebiotic consumption is thought to improve cardiovascular health via favourable effects on the blood lipid profile. A reduction in total cholesterol levels was demonstrated by Wang et al. (Reference Wang, Harding and Thandapilly84) following chronic intake of barley β-glucan in n = 30 mildly hypercholesterolaemic adults (mean serum total cholesterol 5·49 mM). In this study, participants consumed breakfast with 3 g high-molecular-weight, 5 g low-molecular-weight or 3 g low-molecular-weight barley β-glucan (given as breakfast foods in the format of crepes, tortillas, porridge and chips formulated from barley to contain β-glucan varying in molecular weight) or a control diet (breakfast foods formulated from wheat and rice to substitute barley) for 5 weeks. They found that, while serum total cholesterol was reduced significantly, by 2·18 %, after intake of 3 g high-molecular-weight barley β-glucans, cholesterol absorption and synthesis (both assessed using stable isotope methods) were unaffected (Reference Wang, Harding and Thandapilly84). Increased serum 7α-hydroxy-4-cholesten-3-one concentrations, representing synthesis of bile acids, was noted in participants following consumption of the high-molecular-weight β-glucan. These results indicate that the cholesterol-lowering action of β-glucans may be related to enhanced bile acid synthesis, utilising circulating cholesterol, as opposed to inhibition of cholesterol synthesis or absorption. Another study by Nicolucci (2017) demonstrated a reduction in serum TAG in healthy, overweight children (n = 22) following supplementation with 8 g/d of oligofructose-enriched inulin for 16 weeks, compared with an isoenergetic dose of 3·3 g/d of maltodextrin placebo. The improvement in serum lipids coincided with increased faecal Bifidobacterium proliferation, many strains of which have strong BSH activity (Reference Nicolucci, Hume and Martínez85). In a study by Cronin et al. (Reference Cronin, Allsopp and Slevin86), daily calcium supplements (800 mg/d) consumed with short-chain fructo-oligosaccharides (3 g/d) for 24 months led to a time-by-treatment effect on the reduction in total and LDL-C levels in postmenopausal women (n = 300) compared with daily calcium (800 mg) alone (Reference Cronin, Allsopp and Slevin86). The authors speculated that the reduced cholesterol absorption in the gut could be due to increased binding with the fructo-oligosaccharides, and potentially the bile acids, promoting their excretion in the stool.
Effects on bile acids
As with probiotics, several studies (n = 16) have found a link between consumption of prebiotic foods and altered bile acid profiles or metabolism (Table 2 and Supplementary Table 1). For example, a number of studies in animal models have shown increased excretion of total bile acids in the faeces after chronic supplementation (between 4 and 17 weeks duration) with a prebiotic compared with a control intervention (Reference Meneses, Martínez-Carrera and Torres87–Reference Gunness, Michiels and Vanhaecke90). This has also been demonstrated in two human studies (Reference Nicolucci, Hume and Martínez85,Reference Ellegård and Andersson91) . In some cases, the enhanced excretion of bile acids could be related to increased proliferation of probiotic bacteria, including Lactobacillus and Bifidobacterium in the gut, possibly due to the BSH activity of such strains of bacteria. For example, Drzikova et al. (Reference Drzikova, Dongowski and Gebhardt92) found, in addition to increased faecal total bile acids and reduced serum total cholesterol, an increase in gut proliferation of Bifidobacterium following consumption of 500 g oat-based extrudates, in the form of oat flour or oat bran, for 6 weeks in rats (n = 10) (Reference Drzikova, Dongowski and Gebhardt92). Similarly, Meneses et al. (Reference Meneses, Martínez-Carrera and Torres87) found increased gut Lactobacillus proliferation, as well as increased bile acid excretion, in mice (n = 8) after ingestion of Ganoderma lucidum, an oriental fungus with prebiotic properties, with a high-cholesterol diet (Reference Meneses, Martínez-Carrera and Torres87). This was also related to improved lipid profiles, with reduced serum total cholesterol (by 19·2–27·1 %), LDL-C (by 4·5–35·1 %) and TAG (by 16·3–46·6 %) concentrations in the mice. A study by Gunness et al. (Reference Gunness, Michiels and Vanhaecke90) also found that a diet rich in oat β-glucans for 28 d reduced blood total bile acids, total and LDL-C compared with a control diet (no addition of β-glucan) in pigs (n = 6) (Reference Gunness, Michiels and Vanhaecke90). These findings were associated with increased faecal UDCA, changes in faecal HDCA and LCA (non-significant) and an overall reduction in faecal fatty acids, suggesting that β-glucans can alter bile acid metabolism. These results could provide, in part, the mechanism for the cholesterol-lowering effect of such foods since consumption of prebiotics can enhance proliferation of bacteria with bile acid metabolising activity in the gut.
In contrast, in a study by Wu et al. (Reference Wu, Cheng and Chen167), faecal secondary bile acids were reduced, while primary bile acids were increased in healthy adults (n = 15) after consumption of konjac (4·5 g/d), a high-molecular-weight, non-ionic, linear glucomannan consisting of β-1,4-linkages which resist digestion in the upper GI tract and is thus a rich source of soluble fibre. This was despite gut Bifidobacterium and Lactobacillus growth being increased, indicating a prebiotic effect, with enhanced proliferation of BSH bacteria strains. Although the effect on lipid profiles was not measured in this study, it may be that different mechanisms are involved in the cholesterol-lowering effect of some prebiotic foods, other than bile acid metabolising activity and subsequent reduced adsorption/increased neo-synthesis of bile acids. For example, it is known that konjac consumption can increase SCFA production in the gut and thus can modulate both BA profiles and SCFA concentrations (Reference Chen, Cheng and Wu93).
Some studies have also found addition of prebiotics to the diet to modulate circulating bile acid levels. For example, Hijova et al. (Reference Hijová, Bomba and Bertková94) found reduced serum bile acids after a high-fat diet supplemented with 2 % oligofructose-enriched inulin with extracts of horse chestnut (1 %) and/or flaxseed (2 %) in rats (n = 12), compared with a high-fat diet alone. For rats treated with inulin alone, this was also linked with reduced total cholesterol and TAG concentrations, as well as increased Lactobacilli in the stools (Reference Hijová, Bomba and Bertková94). However, a study by Lærke et al. (Reference Lærke, Pedersen and Mortensen95) found no effect of rye wholemeal and rye bran on circulating bile acids in hypercholesteraemic pigs (n = 8) despite reduced plasma total-to-LDL-C ratio (Reference Lærke, Pedersen and Mortensen95). Although faecal bile acid excretion was not measured, it is also possible that the effect observed is dependent on both the type of prebiotic consumed and the animal species used. For example, pig models have a closer similarity to human lipid metabolism compared with rat models. In addition, certain prebiotics, especially inulin-type fructans, may have a positive effect on endothelial function via effects on the gut microbiota, bile acid profiles and activation of the nitric oxide (NO) synthase/NO pathway responsible for NO-dependent endothelium relaxation. Inulin-type fructans promote proliferation of Bifidobacterium, which contribute to NO generation by reducing pH and acidic non-enzymatic reduction of nitrite (Reference Sobko, Reinders and Jansson96). Catry et al. (Reference Catry, Bindels and Tailleux97) demonstrated a positive effect of inulin-type fructans on endothelial dysfunction, an important marker of CVD, in mice (Reference Catry, Bindels and Tailleux97). Apoe−/− mice were fed an n-3 polyunsaturated fatty acid (PUFA)-depleted diet (12 weeks) with or without inulin-type fructans supplementation (for last 15 d of the study). Endothelial dysfunction was completely reversed in the mesenteric and carotid arteries, through activation of the NO synthase/NO pathway. In addition, inulin-type fructan supplementation led to increased proliferation of NO-producing bacteria and Akkermansia, with reduced abundance in bacteria which are implicated in synthesis of secondary bile acids. The observed changes in bile acid composition, along with increased L-cell density and enhanced GLP-1 production, were proposed as being responsible for activation of the NO synthase/NO pathway and ultimately for preservation of endothelial function.
Although some potential mechanisms for beneficial effects of prebiotics on CVD risk have been derived from the animal studies presented above, the lack of studies conducted in humans which have included measures of gut microbiota composition and markers of bile acid metabolism warrants further study, as the links between bile acid and lipid regulation remain inconclusive.
Polyphenol-rich foods
Diets high in phenolic compounds such as fruits and vegetables are considered to have beneficial effects on gut health. In addition to fibre, polyphenols have also been reported to reach the gut intact where they are fermented by the resident bacteria leading to generation of smaller phenolic compounds which can be absorbed in the colon(Reference Hervert-Hernández and Goñi98). For example, procyanidins are metabolised by the gut bacteria into several metabolites such as phenyl valerolactone and phenylacetic and phenylpropionic acids which are more easily absorbed and capable of producing systemic effects including anti-inflammatory and vasodilatory effects (Reference Holt, Heiss and Kelm99,Reference Zhang, Wang and Li100) .
The prebiotic effect of apples, specifically the high-polyphenol-containing Renetta Canada variety, was highlighted by a study conducted by Koutsos et al. (Reference Koutsos, Lima and Conterno101). Using a batch culture colonic model inoculated with faeces from healthy human donors (n = 3), the apples induced substantial changes in the composition and metabolic activity of the gut microbiota in vitro, with a reduction in abundance of Bacteroidetes, and enhanced proliferation of Proteobacteria, Bifidobacteria and Faecalibacterium prausnitzii, found relative to inulin and cellulose control models(Reference Koutsos, Lima and Conterno101). Sembries et al. (Reference Sembries, Dongowski and Mehrländer102) also showed increased faecal Lactobacilli and Bifidobacterium following addition of apple pomace (the dried solid by-product of apple product manufacturing) to the diet of rats (n = 12) for 4 weeks (Reference Sembries, Dongowski and Mehrländer102). However, apples and apple pomace contain other active components which could have affected the gut microbiota such as fibre in the form of pectin. Prebiotic effects have also been shown for red wine, known to be high in polyphenols, in humans (n = 20) (Reference Queipo-Ortuño, Boto-Ordóñez and Murri103). Consumption of red wine (272 ml/d) enhanced the growth of Blautia coccoides–Eubacterium rectale groups, Bifidobacterium, Eggerthella lenta and Bacteroides uniformis, while growth of Clostridium and Clostridium histolyticum was inhibited after 20 d compared with consumption of an alcohol control (100 ml/d gin). However, such beneficial effects on composition of the gut microbiota were not found in a recent study by Wallace et al. (Reference Wallace, Eady and Hunter104). Indeed, ingestion of a high-polyphenol boysenberry beverage (750 mg polyphenols) did not give rise to any significant changes in faecal bacteria, including total Lactobacilli, Bifidobacterium and Bacteroides or Clostridium perfringens in healthy human volunteers (n = 25) (Reference Wallace, Eady and Hunter104). The discrepancies between different studies may be due to different polyphenol doses, the selected comparator intervention, types of food given and the polyphenolic compounds they contain, with an effect in particular of the food matrix.
Effects on lipid metabolism
Polyphenols, particularly complex polyphenols, such as proanthocyanidins, have been found to influence the enterohepatic circulation via sequestering of bile acids within the colon and/or increasing the proliferation of gut microbiota involved in the de-conjugation and hydrolysis of primary to secondary bile acids (Reference Scalbert and Williamson105–Reference Naumann, Haller and Eisner108). For example, supplementation with green tea polyphenols (3·2 g epigallocatechin gallate (EGCG) per kg of high-fat chow) was found to increase faecal bile acid concentrations by 1·5-fold in mice (n = 20), from approximately 0·4 µmol/d to 1·1 µmol/d (Reference Huang, Feng and Liu109). This likely occurs owing to inhibition of bile acid micelles because of hydrophobic interactions with polyphenolic compounds such as EGCG. This phenomenon has been demonstrated in in vitro studies to lead to the elimination of phosphatidylcholine and cholesterol from the micellar structure, which results in reduced bile acid solubility (Reference Ogawa, Hirose and Nagaoka110). Bile acid binding assays have revealed that, in in vitro mixtures, 30 % of taurocholic acid, 70 % of glycodeoxycholic acid and 25 % of taurodeoxycholic acid could be bound by grape seed extract (containing 50·8 g total flavanols per 100 g of extract) and similarly by the polyphenols gallic acid, catechin and epicatechin (Reference Adisakwattana, Moonrat and Srichairat111,Reference Ngamukote, Mäkynen and Thilawech112) . Further studies, particularly in humans, would be valuable to fully understand the impact of increased polyphenol intakes on bile acid sequestering action.
Indeed, consumption of polyphenols has been shown to improve dyslipidaemia in several human studies. Koutsos et al. (Reference Koutsos, Riccadonna and Ulaszewska113) found a beneficial effect of consuming two high-polyphenol Renata Canada apples daily (total polyphenols, 239 mg/d) for 8 weeks on lipid profiles, with reduced serum total and LDL-C and TAG concentrations in slightly hypercholesteraemic subjects (n = 40) (Reference Koutsos, Riccadonna and Ulaszewska113). The changes in serum lipids were also associated with small but significant improvements in endothelium-dependent microvascular vasodilation, a marker of endothelial function and another indicator of cardiovascular health. No significant changes in circulating bile acids were found, but further exploratory analysis demonstrated links between circulating LCA and glycoursodeoxycholic acid (GUDCA) and the total cholesterol response in women. Fujita and Yamagami (2008) found a similar response to black tea polyphenols, with improved circulating total and LDL-C and TAG levels following 3 months of supplementation with 166·5 mg black tea extract (taken in tablet form) (n = 47) (Reference Fujita and Yamagami114). Furthermore, Tzounis et al. (Reference Tzounis, Rodriguez-Mateos and Vulevic170) also demonstrated significant reductions in plasma TAG concentrations in healthy human volunteers (n = 22) following ingestion of a high-cocoa-flavanol drink (494 mg cocoa flavanols/d) for a 4-week period, compared with a low-cocoa-flavanol drink (23 mg cocoa flavanols/day). This occurred concurrently with significantly increased Bifidobacterium and Lactobacilli populations, as well as significantly reduced Clostridia counts in the gut, demonstrating the potential link between polyphenol-induced alterations in gut microbiota and improved CVD risk markers.
Beneficial effects of polyphenols on CVD risk are not limited to improved lipid profiles. In rat models of CVD, the polyphenol resveratrol (0·4 % administered in a standard chow diet) was shown to lower production of trimethylamine-N-oxide. This amine oxide is generated from choline, betaine and carnitine by gut microbial metabolism of red meat and fat (Reference Chen, Yi and Zhang48) and is linked with an increased risk for significant adverse cardiovascular events (115). Trimethylamine-N-oxide encourages development of atherosclerosis (narrowing of arteries caused by fatty plaques, leading to CVD), and plays a role in cholesterol uptake and BA synthesis, reducing reverse cholesterol transport and downregulating hepatic CYP7A1 activity (116,117). Indeed, in the same rat model, a reduction in vascular disease was observed (48). The findings observed were shown to correlate with the increased production of new bile acids due to changes in the enterohepatic FXR-FGF15 pathway after modulation of the gut microbiota. Resveratrol was found to significantly increase expression of CYP7A1, leading to increased BA synthesis in the liver while also enhancing the growth of Lactobacillus and Bifidobacterium in the gut (48). It was suggested, therefore, that consumption of this polyphenol can limit the effects of trimethylamine-N-oxide on CVD development by promoting greater uptake of circulating cholesterol by the liver to make new bile acids.
Effects on bile acids
Evidence from the animal studies may provide an explanation for the potential mechanisms of action behind polyphenol-induced improvements in lipid risk markers, since these beneficial effects are linked to changes in circulating and excreted bile acids. A summary of studies conducted in humans and animals is presented in Table 1 and Supplementary Table 1 (see supplementary material), respectively. Aprikian et al. (Reference Aprikian, Busserolles and Manach118) found that the daily consumption of apples decreased plasma total and LDL-C (by 22 % and 70 %, respectively) concentrations in rats, which were associated with increased faecal excretion of total bile acids (+56 % in lean and +30 % in obese rats) (Reference Aprikian, Busserolles and Manach118). A recent study by Ravn-Haren et al. (Reference Ravn-Haren, Krath and Markowski119) also demonstrated a beneficial effect of apple pomace on total cholesterol levels in mice (n = 40), with an associated increase in excreted total and primary bile acids after 4 weeks of consumption (Reference Ravn-Haren, Krath and Markowski119). In another study, similar results were shown following supplementation with the green tea polyphenol, EGCG, with reduced serum cholesterol concentrations and severity of fatty liver disease and increased faecal excretion of cholesterol and total lipids in mice (n = 50). Reduced intestinal and increased faecal excretion of total bile acids was also found, indicating an effect on bile acid reabsorption and neo-synthesis as a potential mechanism for the observed reduction in cholesterol levels (Reference Huang, Feng and Liu109). A pattern of increased faecal bile acids, with reduced serum total and LDL-C and TAG concentrations, has been shown in multiple studies in animal models, using a range of polyphenols (EGCG, kaempferol, anthocyanins, flavonoid extracts, quercetin and resveratrol) and high-polyphenol-containing foods (pu-erh tea, cassini herbal tea and red yeast rice) (Reference Chavez-Santoscoy, Gutierrez-Uribe and Granados120–Reference Hoang, Jia and Mok128). Conversely, one study by Zhang et al. (Reference Zhang, Xie and Gao129) found no change in TAG or total cholesterol levels, but increased LDL-C, despite increased faecal total bile acid excretion and up-regulation of hepatic CYP7A1 following administration of quercetin (0·4 %) in rats (n = 20) (Reference Zhang, Xie and Gao129). These reported inconsistencies may be due to other studies investigating the effect of quercetin on hypercholesterolemic animals or those fed a high-cholesterol diet, while Zhang et al. used healthy rodents with a standard diet formulation (AIN-93G diet) (Reference Mathew, Yoseph and Dessale121,Reference Zhang, Xie and Gao129,Reference Daniel, Devi and Augusti130) .
A study by Guo et al. (Reference Guo, Han and Tan131) found that treatment with phenolic blueberry extract (5 g per litre of drinking-water daily, 14 weeks) led to improved markers of metabolic disease in mice (n = 9), in conjunction with enhanced brown adipose tissue energy expenditure and improved hepatic lipid metabolism through TGR5 and FXR activation (Reference Guo, Han and Tan131). Notably, the phenolic extract led to increased proliferation of Akkermansia, Bifidobacterium, Lactobacillus and Desulfovibrio in the gut, which correlated with increased plasma secondary bile acids such as chenodeoxycholic acid, DCA and LCA and reduced circulating tauro-(α)-muricholic acid (TαMCA) and tauro-(β)-muricholic acid (TβMCA), both of which are potent FXR inhibitors (Reference Guo, Han and Tan131). Similarly, a study by Anhe et al. (Reference Anhê, Nachbar and Varin132) showed treatment of mice (n = 12) with polyphenol-rich extract of camu camu (an Amazonian fruit, daily oral doses (200 mg/kg) of re-suspended crude extract for 8 weeks), led to improved metabolic health with improved glucose tolerance, reduced weight gain and adiposity and blunted metabolic inflammation and endotoxaemia (Reference Anhê, Nachbar and Varin132). This was linked to enhanced brown adipose tissue energy expenditure and strongly correlated with increased expression of TGR5 in brown adipocytes (Reference Anhê, Nachbar and Varin132). In addition, the extract led to altered plasma bile acid profiles, with reduced circulating TαMCA and TβMCA and increased proportion of β-muricholic acid, ω-muricholic acid, chenodeoxycholic acid, DCA and UDCA as well as decreased circulating bile acids and increased proportion of secondary and of unconjugated bile acids. This was coupled with a significant increase in gut Akkermansia muciniphila and a reduction in Lactobacillus. These results indicate that activation of nuclear receptors, TGR5 and FXR play a key role in reduced metabolic disease risk from consumption of phenolic compounds and related to microbial metabolism of bile acids, resulting in alterations in the bile acid pool with reduced FXR antagonists (such as TαMCA and TβMCA) and increased FXR/TGR5 agonists (such as cholic acid).
However, most of the above studies only quantified total bile acids and did not measure bile acid profiles and were conducted in animal models. Thus, further mechanistic studies investigating polyphenol consumption, microbiota modulation and changes in circulating bile acid profiles in humans are warranted.
The effect of phenolic compounds on bile acids may be specific to the type of polyphenol and the matrix in which it is given. In humans, consumption of sun-dried raisins for 6 weeks resulted in reduced faecal bile acids compared with baseline levels, especially for lithocholic, deoxycholic, chenodeoxycholic and cholic acids (Reference Spiller, Story and Lodics133). It is worth noting that, while raisins are high in phenolic compounds (total phenolic content approximately 9–12 mg of gallic acid equivalents per gram), they also contain high levels of fibre, which could play a role in altering bile acid profiles either physically by binding of bile acids and inhibiting their resorption or via effects on the gut microbiota (Reference Williamson and Carughi134). Furthermore, black tea polyphenols, given as a powder or in tablet form, have been shown to have no effect on excreted bile acids in humans, despite their beneficial effect on lipid profiles (Reference Fujita and Yamagami114,Reference Mai, Katki and Harmsen135) . Future studies could be useful to further investigate links between changes in bile acids and improvements in CVD risk markers following polyphenol consumption.
Potential mechanisms of action
The studies highlighted above indicate that a number of potential mechanisms may exist by which dietary components that promote beneficial changes on gut microbiota composition impact on lipid regulation (Fig. 3).
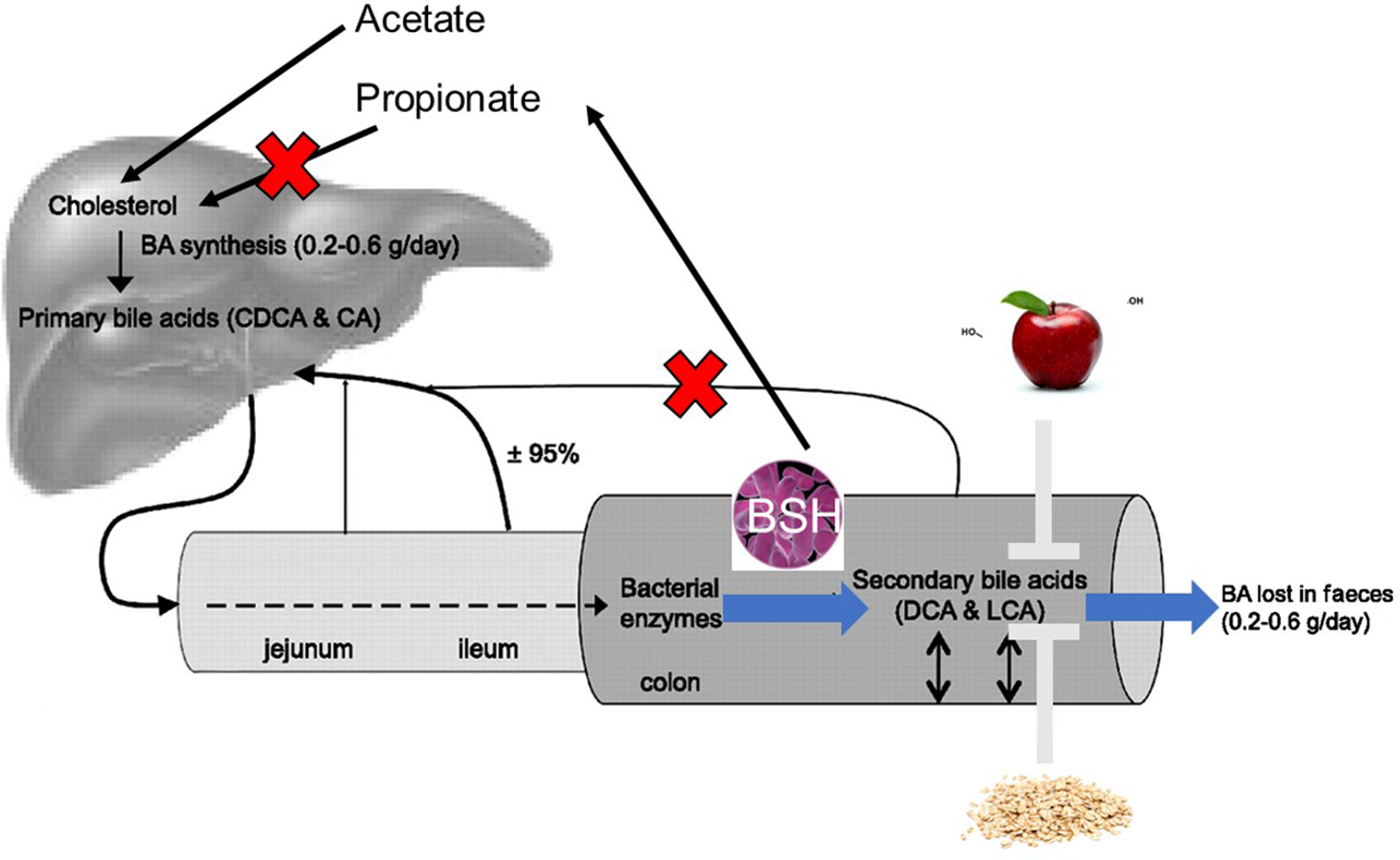
Fig. 3. Potential mechanisms to explain the beneficial effects of prebiotics, probiotics and polyphenol-rich foods on bile acid metabolism and lipid regulation: BSH strains of probiotic bacteria in the gut can modify primary bile acids to produce secondary bile acids which are less well absorbed in the enterohepatic cycle. These bacteria also produce SCFAs, via fermentation of fibre, acetate, which enhances hepatic cholesterol synthesis, and propionate, which may inhibit cholesterol synthesis and encourage uptake of circulating cholesterol via up-regulation of hepatic LDL-receptors. Fibre and polyphenol-rich foods may act as bile sequestering agents, enhancing excretion of bile acids and encouraging bile acid neo-synthesis to replace those lost in the faeces.
BSH activity
In addition to effects on absorption of bile acids, via bile acid metabolising action initiated by BSH activity, the ability of pre/probiotics and polyphenol-rich foods to either stimulate or inhibit FXR may also affect their ability to impact upon host metabolism. In a study by Degirolamo et al. (Reference Degirolamo, Rainaldi and Bovenga78), the probiotic mixture VSL#3 (containing BSH active probiotic strains) had a significant effect on bile acid profiles in mice after oral gavage with 50 × 109 CFU/d for 21 d, compared with daily oral gavage with saline for the same duration (Reference Degirolamo, Rainaldi and Bovenga78). The high levels of BSH activity inhibited the enterohepatic FXR response and led to increased excretion of total bile acids in the faeces with no effect on the faecal CA/DCA ratio but a reduced ratio of faecal conjugated:unconjugated bile acids. In addition, a reduction in gut FGF15 expression and increased bile acid synthesis in the liver was shown; however, serum lipids were not measured. FGF15 is the mouse homologue of FGF19 (released upon activation of FXR) which migrates to the liver to activate the cellular receptor FGFR4, inhibiting CYP7A1 expression (Reference Inagaki, Choi and Moschetta136). Conversely, Choi et al. (Reference Choi, Kim and Jeong171) found increased FXR expression in mice following a high-fat diet supplemented with L. curvatus and L. plantarum (n = 10) for 6 weeks. This was coupled with changes in metabolic markers such as reduced plasma TAG. Similarly, in humans, FGF19 expression may be increased in response to gut proliferation with probiotic bacteria. For example, in addition to effects on LDL-C concentrations, Martoni et al. (Reference Martoni, Labbé and Ganopolsky74) found that Lactobacillus reuteri supplementation led to stimulation of the FXR axis as well as increased levels of circulating bile acids. As such, in addition to further in vivo studies on probiotics and bile acid excretion, studies on the effect of probiotics and food components, which lead to increased proliferation of probiotic bacteria in the gut, on FXR activity would also be of interest.
Bile acid sequestrants
Another mechanism to explain the increased excretion of bile acids following consumption of prebiotics and polyphenol-rich foods could be due to the bile sequestering capacity of these dietary components (Fig. 3). Dietary fibre such as β-glucans and polyphenol compounds can bind to bile acids in the intestine to drive the bile acids down to the colon, which modifies their absorption and excretion (Reference Chen and Huang137). This leads to a reduction in hepatic bile acid concentration and, in turn, activates CYP7A1, the enzyme responsible for conversion of cholesterol to bile acids(Reference Theuwissen and Mensink138). A number of studies have noted increased hepatic CYP7A1 expression, indicating bile acid neo-synthesis following consumption of polyphenolic compounds in animals (Reference Hirsova, Karlasova and Dolezelova139,Reference Watanabe and Ayugase140) . In rodents, increased CYP7A1 expression has been shown in conjunction with reduced serum total cholesterol and TAG following administration of grape seed polyphenols (Reference Jiao, Zhang and Yu141,Reference Heidker, Caiozzi and Ricketts142) . In line with these findings, Downing et al. (Reference Downing, Heidker and Caiozzi143) showed elevated faecal bile acids and reduced serum bile acids following administration of grape seed polyphenols in rats (n = 8) (Reference Downing, Heidker and Caiozzi143). In addition, the grape seed polyphenols resulted in reduced serum TAG levels and increased faecal excretion of cholesterol and total lipids. However, serum total cholesterol was not affected in this study. Quifer et al. (Reference Quifer-Rada, Choy and Calvert144) also found increased faecal excretion of cholesterol, as well as increased excretion of secondary bile acids deoxycholic and lithocholic acid, following grape seed extract (GSE) consumption in pigs (n = 6) (Reference Quifer-Rada, Choy and Calvert144).
Evidence for increased CYP7A1 expression in the liver has also been demonstrated in animal models with consumption of prebiotics. Mandimika et al. (Reference Mandimika, Paturi and De Guzman89) found increased hepatic CYP7A1 expression and improved circulating lipid profiles after consumption of broccoli fibre (75 g/kg daily) in rats (n = 16) fed a high-corn-oil diet for 17 weeks. However, Lærke et al. (Reference Lærke, Pedersen and Mortensen95) found that consumption of rye wholemeal and rye bran led to inhibition of CYP7A1 expression in hypercholesteraemic pigs (n = 8) along with reduced plasma TC/LDL-C; therefore, the effect may be specific to type of prebiotic given and animal model used (95).
Chronic supplementation with probiotics may also lead to increased hepatic CYP7A1. For example, a study by Jeun et al. (Reference Jeun, Kim and Cho71) found a greater CYP7A1 expression, coupled with increased bile acid excretion and improved lipid profiles, following supplementation with L. plantarum (109 CFU) in mice (n = 7) for 4 weeks (Reference Jeun, Kim and Cho71). Similarly, Michael et al. (Reference Michael, Davies and Moss76) showed the same effects on CYP7A1 expression, bile acid excretion and improved blood lipids, following 14 d of consumption of L. plantarum (108 CFU/d) in mice (n = 12) (Reference Michael, Davies and Moss76). The effect on CYP7A1 expression and lipid profiles was revealed by Wang et al. (Reference Wang, Huang and Xia145), while Zhai et al. (Reference Zhai, Liu and Wang75) also found a similar effect on bile acid excretion but did not include measurement of lipid parameters (Reference Zhai, Liu and Wang75,Reference Wang, Huang and Xia145) . These results demonstrate a beneficial effect of L. plantarum on CVD risk markers, which may be in part mediated by increased bile acid neo-synthesis.
Expression of lipid synthesis genes
In addition, fermentation of soluble fibres by the gut bacteria results in the production of SCFA which are easily absorbed into the portal vein and can then be metabolised in the liver. There is some evidence that SCFA, particularly propionic acid, are involved in suppression of 3-hydroxy-3-methyl-glutaryl-CoA reductase (HMGR), the rate-limiting enzyme for cholesterol synthesis in the liver, leading to up-regulation of LDL receptors (Fig. 3) (Reference Hara, Haga and Aoyama146). For example, Meneses et al. (Reference Meneses, Martínez-Carrera and Torres87) showed that a high-cholesterol diet supplemented with extract of Ganoderma lucidum (0·5–1·0 %) for 43 d in C57BL/6 mice (n = 8) led to reduced expression of HMGR coupled with an increase in LDL-receptor gene expression in the liver (Reference Meneses, Martínez-Carrera and Torres87). These changes in gene expression coincided with reduced serum total and LDL-C, TAG levels, and hepatic total cholesterol and TAG content. Supplementation with probiotic bacteria, Enterococcus faecium (5·0 × 109 CFU/ml in PBS, 1 ml/d) for 35 d has also been shown to inhibit expression of hepatic HMGR, while increasing LDL-receptor and CYP7A1 expression in mice (n = 5), with resulting improvements in serum lipid profiles (Reference Huang, Zhang and Xu67). Similar effects have been demonstrated for polyphenol extracts with reduced HMGR expression after consumption of a flavonoid- and saponin-rich extract (0·25–0·5 %) with a high-cholesterol diet for 35 d in mice (n = 8) (Reference Chavez-Santoscoy, Gutierrez-Uribe and Granados120). These changes were in parallel to the increased faecal bile acid secretion and bile acid neo-synthesis (increased CYP7A1 in the liver) observed, as well as beneficial effects on circulating lipid profiles. Conversely, several studies have shown that consumption of probiotics, prebiotics or polyphenol-rich foods may result in up-regulation of hepatic HMGR expression. For example, Damodharan et al. (Reference Damodharan, Lee and Palaniyandi77) found increased HMGR expression in mice (n = 8), following ingestion of L. acidophilus (3 × 108 CFU/ml) with a high-cholesterol diet for 32 d (Reference Damodharan, Lee and Palaniyandi77). This was despite an increase in hepatic LDL-receptor expression and reduced serum LDL-C. A study by Parnell et al. (Reference Parnell and Reimer156) also showed increased hepatic HMGR as a result of oligofructose and inulin supplementation (10–20 %) for 10 weeks in rats (n = 8). Indeed, Mandimika et al. (Reference Mandimika, Paturi and De Guzman89) demonstrated increased hepatic HMGR expression in rats (n = 16) when fed broccoli fibre (75g/kg daily) as part of a high-corn-oil diet for 17 weeks (Reference Mandimika, Paturi and De Guzman89). This was despite improvements in circulating lipid profiles and increased excretion of bile acids. In addition, Mueller et al. (Reference Mueller, Zhang and Juraschek147) found an association between increased serum propionate and raised LDL-C in humans (n = 163) using linear regression models to examine the relationship between changes in SCFAs with cardio-metabolic parameters following introduction of different diets (carbohydrate-rich, protein-rich and unsaturated-fat-rich) for 6 weeks (Reference Mueller, Zhang and Juraschek147). These contrasting findings could reflect the differences in lipid metabolism pathways which exist between rats and humans. Further studies are needed to fully elucidate the role of SCFAs in regulating cholesterol synthesis in humans.
There is limited evidence that the different SCFA have differential effects of lipid metabolism, although this has mainly been demonstrated in rodent models. The major SCFA in the colon is acetate, which has been shown to up-regulate hepatic lipid synthesis (Reference Fushimi, Suruga and Oshima148). Meanwhile, propionate has been reported to reduce cholesterol synthesis via inhibition of HMGR, in animal models (Reference Wright, Anderson and Bridges149). Therefore, it is possible that dietary components which reduce the acetate-to-propionate ratio may inhibit de novo lipid synthesis to reduce serum lipids (Reference Wong, De Souza and Kendall150). Different strains of bacteria produce different amounts of each type of SCFA, which may explain the differences observed in studies investigating the effects of gut-microbe-altering foods on HMGR expression. For example, Bifidobacteria and Lactobacilli do not produce butyrate (Reference Zampa, Silvi and Fabiani151). Lactobacillus rhamnosus is capable of producing significant amounts of propionate but not either butyrate or acetate. Meanwhile, strains of Bifidobacterium B. longum and B. bifidum both produce acetate, with the latter also capable of propionate production (Reference LeBlanc, Chain and Martín152). The majority of butyrate-producing bacteria belong to the Firmicutes phylum, including the families Ruminococcaceae and Lachnospiraceae (Reference Geirnaert, Calatayud and Grootaert153). However, the situation is likely to be more complicated since SCFA profiles remain similar between individuals despite significant inter-individual differences in gut microbiota composition, likely due to the vast overall numbers of SCFA-producing bacteria in the gut (Reference Reichardt, Vollmer and Holtrop154).
The evidence for an effect of foods which may increase production of SCFAs on expression of hepatic HMGR is conflicting, and reductions in plasma cholesterol may not occur solely via inhibition of cholesterol synthesis in the liver. Indeed, it has been shown that SCFAs can up-regulate expression of hepatic SREBP2 mRNA, resulting in activation of LDL-receptor gene expression and, therefore, improved circulating lipoprotein profiles via enhanced clearance of circulating LDL particles (Reference Zhao, Liu and Hao155). Increased expression of SREBP2 mRNA in the liver has been shown in a limited number of studies in animal models following both probiotic (Reference Damodharan, Lee and Palaniyandi77) and prebiotic supplementation (Reference Parnell and Reimer156).
Studies investigating the mechanisms underlying the relationship between circulating bile acids and lipid CVD risk markers in response to probiotics, prebiotics and polyphenol-rich foods have been predominately conducted in animals. However, these findings are not always transferable between species. Some animals, including rats, do not have a gall bladder and thus may display differences in bile acid flow in response to food intake. This unfortunately presents a limitation of using animal models for looking at bile acid metabolism and highlights the need for more studies to be performed in humans which incorporate measures of bile acid metabolism and CVD risk.
Conclusion
There is a growing body of evidence for a link between the consumption of probiotics, prebiotics and polyphenol-rich foods and the gut microbiota, which may be due to effects on bile acid metabolism. It has been shown that these dietary components can encourage bile acid excretion and neo-synthesis, providing a mechanism for the beneficial effects of these compounds on circulating lipid profiles. Animal model studies have demonstrated an effect of prebiotics, probiotics and polyphenol-rich foods/extracts on the FXR/FGF-15 axis as well as generally increasing expression of hepatic CYP7A1 (the rate-limiting enzyme for bile acid synthesis) and potential effects on endogenous cholesterol synthesis via SREBP and HMGR genes leading to the enhanced clearance of circulating LDL. It is evident that further research in humans is needed to fully define the relationship between diet and bile acid metabolism to determine the mechanisms behind the beneficial effects of these dietary components on host health and CVD risk. Further research could establish if circulating bile acids can be utilised as a biomarker to identify potential inflammatory and metabolic health in humans as well as which dietary components can potentially influence this relationship.
Financial Support
R.G.P. was supported by the BBSRC (grant number BB/P028209/1) as part of the project HDHL-Biomarkers: CirculAting Bile Acids as biomarkers of metabolic health - Linking microbiotA, Diet and Health (CABALA_DIET&HEALTH), and S.A. was supported by a Kuwait Government studentship award.
Conflict of Interest
None.
Authorship
R.G.P. conducted the literature searches and wrote the main body of text. S.A. assisted with literature searches and compiling of tables. J.A.L. and K.G.J. participated in the concept and planning of the review as well as revision of the manuscript, and J.A.L. was responsible for the final content of the manuscript.
Supplementary material
To view supplementary material for this article, please visit https://doi.org/10.1017/S0954422421000081