Introduction
The mitigation of global antimicrobial resistance (AMR) will require national and global coordination of interventions at the technological, behavioural, economic and political levels. Different tools need to be deployed intelligently; these include incentivizing new antimicrobial development, stewarding existing antimicrobials better, improving diagnostics to identify optimal treatments for patients and enhancing control measures to prevent infection spread (O’Neill, Reference O’Neill2016). A key tool that offers unique advantages in combating AMR spread is the development and use of vaccines against infectious diseases.
The potential for vaccination as a tool against AMR has long been recognized, but has recently received renewed attention (Abbott, Reference Abbott2017). Reductions in antibiotic use and bacterial resistance were observed after the introduction of vaccines against Streptococcus pneumoniae (Fireman et al., Reference Fireman, Black and Shinefield2003) and Haemophilus influenzae (Tandon & Gebski, Reference Tandon and Gebski1991). Publicly commissioned reviews of AMR in the United Kingdom (O’Neill, Reference O’Neill2016), the European Union (European Commission, Reference Commission2017) and the United States (The White House, Reference House2015) have pinpointed the role of vaccines as a key measure to lower demand for antimicrobials and hence combat AMR.
However, these reviews underestimate the potential benefit of vaccines because they only consider a subset of the pathways by which vaccines can affect antimicrobial use and resistance. Several additional reviews have recently outlined multiple interacting ecological, epidemiological and health systems pathways through which vaccines affect AMR (Lipsitch & Siber, Reference Lipsitch and Siber2016; Atkins et al., Reference Atkins, Lafferty and Deeny2018).
How vaccines can reduce the burden of AMR
Vaccines used in humans
Vaccines may act to reduce the burden of AMR through a number of pathways (Lipsitch & Siber, Reference Lipsitch and Siber2016; Atkins et al., Reference Atkins, Lafferty and Deeny2018). Below we briefly describe six potentially important effects to consider (Figure 8.1).
1) Preventing infections by focal pathogens. Vaccines may reduce the incidence of infection by a resistant pathogen. This can occur both through direct protection to those vaccinated, and through indirect protection resulting from reduced exposure to the infection in the unvaccinated (herd immunity). Use of vaccines that either reduce risk of infection or reduce transmission by infected vaccinees may result in these effects. A clear example of this is the H. influenzae type b (Hib) conjugate vaccine (see next section).
2) Bystander effects. Any vaccines that lead to changes in antibiotic use could potentially have an impact on AMR in organisms not targeted by the vaccine as a result of reduced antibiotic selection pressure. For example, since influenza infections are frequently treated with antibiotics (either inappropriately for the primary viral infection, or for a secondary bacterial infection), an effective and widely used vaccine that reduces the number of influenza infections should result in population-wide reductions in antibiotic use. In some cases, these reductions in antibiotic use may lead to reductions in resistance (Fireman et al., Reference Fireman, Black and Shinefield2003; Kwong et al., Reference Kwong, Stukel and Lim2008; Dagan et al., Reference Dagan, Barkai and Givon-Lavi2008). Since many bacterial pathogens are commonly carried asymptomatically and only rarely cause disease, such bystander effects may be the main path by which resistance is selected for. A reduction in bystander selection could thus have a substantial impact. It is important to note that the potential changes in antibiotic use resulting from vaccination may include both reductions in the volume of antibiotics used (fewer infections that need treating as a result of vaccination), as well as changes in the choice of antibiotic (for example, there may be reduced need for broad-spectrum antibiotics for a clinical syndrome if the vaccine reduces a resistant organism sufficiently that such cover is no longer required). Both changes could also lead to reductions in bystander selection of resistance.
3) Infection severity effects. Vaccines that reduce the risk of symptomatic infection without reducing the risk of carriage/asymptomatic infection can lead to reductions in the proportion of infections which are treated with antimicrobials and therefore a reduction in the selection pressure for resistant phenotypes. Malaria caused by Plasmodium falciparum provides an example where natural immunization may have played a role in slowing the emergence of resistance in some settings. In high malaria prevalence settings, levels of immunity in the population are higher than in low prevalence settings; as a result, a higher proportion of infections are asymptomatic and therefore untreated, leading to overall lower selection for resistance. This simple effect may explain why antimalarial resistance has historically arisen in lower-prevalence Asian settings rather than in higher prevalence areas of Africa (Pongtavornpinyo et al., Reference Pongtavornpinyo, Yeung and Hastings2008).
4) Subtype selection effects. When a pathogen population is composed of multiple competing subtypes and the vaccine targets only a subset of these, then if targeted subtypes are more likely to be resistant, overall resistance may decrease. The experience with pneumococcal conjugate vaccines provides an example of this (Dagan et al., Reference Dagan, Melamed and Muallem1996). Note that this type of selection could also lead to increases in resistance if subtypes targeted by the vaccine are less likely to be resistant, and initial reductions in resistance might be reduced by subsequent acquisition of resistance by non-vaccine serotypes (Mera et al., Reference Mera, Miller and Fritsche2008).
5) Interspecific effects. Bacteria and viruses interact in complex ways. For example, influenza or respiratory syncytial virus (RSV) infections may increase the risk of secondary bacterial infections (Mera et al., Reference Mera, Miller and Fritsche2008; McCullers, Reference McCullers2006; Bosch et al., Reference Bosch, Biesbroek and Trzcinski2013; Joseph, Togawa & Shindo, 2013) and patients with certain viral infections may transmit more bacterial pathogens (Eichenwald, Reference Eichenwald1960; Sherertz, Reference Sherertz1996). Vaccination against one organism could therefore reduce transmission of another, leading to declines in both resistant and sensitive phenotypes. Note that in some cases competition effects between species could mean that vaccination against one organism could result in increases in risks of infection with competing species, as has been suggested for Staphylococcus aureus following pneumococcal vaccination (Brook & Gober, Reference Brook and Gober2009).
6) Selective targeting effects. Interventions, such as hygiene improvements or vaccination, which might be expected to have equal effects on sensitive and resistant phenotypes of a given pathogen, could lead to differential effects if targeted to certain groups in structured populations. For example, if a resistant strain of a given pathogen transmits preferentially in hospitals (where antibiotic use is high), while a competing sensitive strains transmits better in the community, targeting the hospital population with a vaccine or passive immunotherapy conferring short-lived immunity would be expected to have a greater overall effect on the resistant strain, leading to declines in resistance in both hospital and community (van Kleef et al., Reference van Kleef, Luangasanatip and Bonten2017).
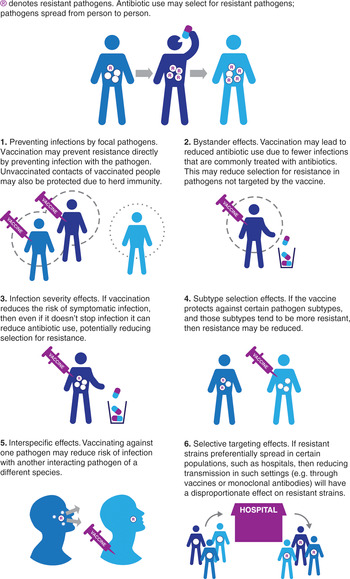
Figure 8.1 Ways in which vaccines may reduce antimicrobial resistance.
Other, more complex, effects may also be in operation. For example, it is conceivable that vaccines could directly or indirectly alter bacterial populations in ways that lead to less or more opportunity for genetic exchange of resistance elements, potentially altering the rate at which resistance genes spread (Lipsitch & Siber, Reference Lipsitch and Siber2016).
It is also possible that vaccines will be developed to exploit other ways of reducing resistance. For example, it may be possible to develop vaccines to selectively target highly-resistant clones of a bacterial species. This may be possible by targeting toxins or other virulence factors which appear to be stably associated with resistant lineages (Wardenburg & Schneewind, Reference Wardenburg and Schneewind2008), or by targeting resistance determinants themselves (Senna et al., Reference Senna, Roth and Oliveira2003; Zarantonelli et al., Reference Zarantonelli, Antignac and Lancellotti2006; Joice & Lipsitch, Reference Joice and Lipsitch2013).
Vaccines used in animal production systems
The mechanisms by which vaccines may help reduce AMR in human pathogens also apply to animal production systems. Food-producing animals represent an important reservoir of drug-resistant bacteria and resistance genes. Such resistance may have detrimental effects on animal welfare and agricultural production. It may also affect human health through foodborne infections and through the transfer of resistance genes (Collignon et al., Reference Collignon, Conly and Andremont2009). Antibiotic-resistant bacteria may spread from animals to humans either by direct contact or indirectly, through food preparation and consumption, via contaminated water, and through the use of animal waste as fertilizer (Marshall & Levy, Reference Marshall and Levy2011).
The World Organisation for Animal Health (OIE) has an ad hoc working group that focuses on the prioritization of diseases for which vaccines could reduce antimicrobial use in animals (World Organisation for Animal Health, 2015). This group is initially focusing on pigs, poultry and fish and aiming to identify the potential value of using both existing vaccines and potential new or improved vaccines targeting either bacteria where antimicrobial resistance is a problem or pathogens causing infections that are commonly treated with antibiotics in animal production systems. There may be even greater potential for reducing antimicrobial use in such animal production systems than there is in human populations, because of much greater rates of antibiotic use and the availability of vaccines which are not licensed for human use. This includes, for example, vaccines against Escherichia coli for which no corresponding vaccine is licensed for human populations, as well as many vaccines for pathogens which are important in animals but not in humans.
A good example of how vaccines have been able to reduce anti-microbial use in animal production systems is the experience of salmon farmers in Norway. Antibiotic use in commercial salmon farming in Norway was cut to virtually zero following development of a vaccine against furunculosis, a bacterial fish disease. Before the vaccine was introduced, large amounts of antibiotics were used, mixed in with the fish-feed. Following the introduction and widespread adoption of the vaccine this practice has been virtually eliminated (World Health Organization, Reference Organization2015).
Advantages of vaccination
While vaccines are only one tool among many that can be used to combat AMR, they have a number of characteristics that make them particularly attractive for this purpose.
Vaccines work by enabling the immune system to recognize antigens that are highly specific to their targeted pathogens, and sometimes even to specific strains of a pathogen. In contrast, antibiotics work by targeting bacterial functions that are common across many species of microorganisms, both pathogenic and commensal. Consequently, vaccines usually have little or no effect on the evolution of microorganisms besides the targeted strains. In contrast, antibiotics can impose selective evolutionary pressure on both targeted and non-targeted microorganisms to develop resistance. Hence the development of resistance is likely to be simply a matter of time even if new generations of antibiotics are developed.
The specificity of vaccines opens up potential strategies that are unavailable with antimicrobials, let alone with nonpharmaceutical measures. For example, vaccines can be developed that target strains of a pathogen that are particularly pathogenic. The serotypes in the pneumococcal conjugate vaccines were generally selected to be the ones most likely to cause invasive disease (Hausdorff et al., Reference Hausdorff, Bryant and Paradiso2000). This also means that they target the strains that are generally most likely to develop resistance, since pathogenic strains are the ones that lead to disease symptoms and thus antibiotic use. Going a step further, vaccines could be deliberately designed so that they target strains that are most likely to become resistant.
Furthermore, vaccines are usually deployed as a population-wide, preventive measure. Many vaccines are given in infancy and can protect their recipients for years or even decades. In contrast, antimicrobials need to be used sparingly because of their potential toxicity to the host and host microbiome, as well as to avoid the development of resistance. Hence, in most cases, they are administered only when people are already infected, and have to be continuously administered with each infection. This also means that they have less potential to prevent onward transmission of resistant microorganisms. Even if they reduce host infectivity, there is usually a delay between the onset of infectiousness and the time at which antimicrobials are received and become active. Hence vaccines offer greater potential for long-lasting population-wide effects that can prevent the onset of any disease at all. This potential is demonstrated by the eradication of smallpox and the near elimination of bacteria such as vaccine-targeted strains of H. influenzae and Neisseria meningitidis from countries with high coverage of the corresponding vaccines. Vaccines may be able to eradicate the underlying microbe rather than simply buy more time until resistance to a new antimicrobial emerges.
Vaccines and antimicrobials can work in a synergistic fashion – vaccines can reduce the rate at which populations are infected and hence extend the time until a pathogen evolves resistance to an antimicrobial. An antimicrobial can complement a vaccination programme by treating individuals who are unable to be vaccinated due to immune compromise.
Specific pathogens where vaccines and other immunotherapies could help reduce AMR burden
In this section we consider specific pathogens and the vaccines or other immunotherapies that could be used to combat AMR. We consider both currently licensed products and the pipeline for future immunotherapies. Information is summarized in Table 8.1, but key pathogens and associated vaccines are described in more detail below.
Table 8.1 Vaccine and AMR status for selected important human pathogens
Pathogen | Vaccine status | AMR status | Vaccine action on AMR |
---|---|---|---|
Bacteria | |||
Streptococcus pneumoniae | Conjugate vaccines targeting the main disease-causing pneumococcal serotypes are widely used. Vaccines with broad protection against all serotypes are being investigated | Common resistance to several classes of antibiotics, especially in vaccine serotypes | Direct and bystander effects |
Haemophilus influenzae type b | Conjugate vaccine licensed and widely used | Widespread prior to vaccine introduction | Direct and bystander effects |
Vibrio cholerae | Oral vaccine licensed and widely used | Growing single and multidrug resistance | Mostly direct |
Salmonella typhi | Several vaccines available, with the new conjugate vaccine showing the most promising efficacy profile | Recent global expansion of resistant lineages which are associated with treatment failure to first-line antibiotics | Direct and bystander effects |
Mycobacterium tuberculosis | Licensed widely used live vaccine (BCG) with variable, medium-term efficacy. Vaccines with higher and longer-lived protection in development | Growing single and multidrug resistance | Direct |
Neisseria meningitidis | Vaccines against most of the main disease-causing serogroups (A, B, C, W and Y) | Some resistance to antibiotics | Direct |
Clostridium difficile | Vaccines in development. Monoclonal antibodies licensed | C. difficile infection is associated with antibiotic use. Resistance to antibiotics in C. difficile itself has been observed. | Direct, bystander and selective targeting effects |
Escherichia coli | Several vaccine candidates in development of Enterotoxigenic E. coli (ETEC) and Uropathogenic E. coli (UPEC) | AMR a major concern for UPEC treatment | Direct and bystander effects |
Group B Streptococcus (GBS) | No vaccines licensed, but several candi dates are in development (capsular polysaccharide-protein conjugate vaccines and protein-based vaccines). Currently in phase I and II trials (Heath, Reference Heath2016) | AMR is a concern primarily for patients who are allergic to first-line antibiotics. Resistance to second-line antibiotics is common in many settings (Castor et al., Reference Castor, Whitney and Como-Sabetti2008) | Bystander effects might be expected to dominate given the high frequency of intrapartum antibiotic prophylaxis for GBS |
Klebsiella pneumoniae | No vaccine candidates have been tested in humans | Multidrug resistance is endemic in many parts of world | |
Neisseria gonorrhoeae | No vaccine at present, but indications that the vaccine against N. meningitidis type b may protect against N. gonorrhoeae (Petousis-Harris, Reference Petousis-Harris, Paynter and Morgan2017) | AMR is increasing and a serious concern in some cases leading to untreatable infections |
Viruses | |||
---|---|---|---|
Influenza virus | Several vaccines are licensed and widely used | Some resistance to antivirals | Bystander and interspecific effects |
Rotavirus | Several vaccines are licensed and widely used | No antivirals in use | Bystander effects |
Norovirus | Two vaccines have reached clinical trials. Several in preclinical stages | No antivirals in use | Bystander effects |
Respiratory syncytial virus | Several vaccines are undergoing clinical trial | Not a concern | Bystander and interspecific effects |
Dengue virus | Only licensed vaccine offers partial protection and may increase the risk of severe disease in some vaccinees | No antivirals in use | Bystander effects |
Parasites | |||
---|---|---|---|
Plasmodium falciparum (malaria parasite) | Licensed vaccine offers partial protection | Some resistance to antimalarials | Direct |
Streptococcus pneumoniae
The impact of vaccination with pneumococcal conjugate vaccines on the epidemiology of antibiotic resistance in S. pneumoniae has been well-described and is one of the clearest examples of the potential impact of vaccination on AMR. In the United States the introduction of the seven-valent pneumococcal conjugate vaccine (PCV7) was associated with an 84% reduction in multidrug-resistant invasive pneumococcal disease (Kyaw et al., Reference Kyaw, Lynfield and Schaffner2006). Reductions in resistance occurred through both direct effects (reduced total pneumococcal disease) and serotype selection effects (the vaccine serotypes were more likely to be resistant).
Haemophilus influenzae type b (Hib)
A conjugate vaccine against Hib is widely used. When it was first licensed, antibiotic resistance in Hib was an emerging problem, with both intrinsic resistance mechanisms limiting the activity of many antibiotics and highly-prevalent beta-lactamase production associated with resistance to ampicillin and amoxicillin (Tristram et al., Reference Tristram, Jacobs and Appelbaum2007). The fact that this resistance is not of more concern is the result of the virtual elimination of serious infections with this pathogen (through both direct protection and herd immunity) in areas where population-wide Hib vaccination has been introduced. This highlights the potential of vaccines to reduce the AMR burden by simply reducing infections with the targeted pathogen.
Neisseria meningitidis
Resistance in the bacteria causing meningococcal disease has emerged, although it is not yet a widespread problem (Bash & Matthias, Reference Bash and Matthias2017). Vaccines now exist against most of the main disease-causing serogroups of N. meningitidis (A, B, C, W and Y).
Vibrio cholerae
Concerns about cholera, which is caused by the bacterial pathogen V. cholerae, have been greatly increased by the emergence of antibiotic resistant lineages. Resistance to first-line antibiotics and multidrug resistance both occur frequently and are associated with more severe illness (World Health Organization, Reference Organization2017a; Gupta et al., Reference Gupta, Pant and Bhandari2016). Either one or two doses of the oral cholera vaccine are effective at preventing medically-attended cholera (Azman et al., Reference Azman, Parker and Rumunu2016). Use of this vaccine clearly has the potential to reduce AMR through its direct effect on cholera.
Nosocomial bacterial pathogens
Multidrug resistance in bacterial species associated with nosocomial infection is common, facilitated by high levels of antibiotic use in health-care settings, high densities of patients, staff and visitors in close proximity as well as a high prevalence of immunocompromize among patients. Immunotherapies against several of these species are currently under development. Such therapies include active vaccines which lead to humoral and cellular immunity and passive immunotherapy which involves direct transfer of antibodies. Active vaccines can provide longer protection against target pathogens and are likely to be substantially cheaper. Among important nosocomial pathogens, pipelines for such active immunotherapies are limited to ongoing phase II/III clinical trials for Clostridium difficile, Staphylococcus aureus and Pseudomonas aeruginosa, with an earliest possible anticipated registration date of 2019 (Czaplewski et al., Reference Czaplewski, Bax and Clokie2016).
Passive immunotherapies based on pathogen-specific monoclonal antibodies (mAbs), while only providing transient protection, have the potential benefit over active vaccines in that they confer immunity to the whole population including the elderly and immunocompromized who may often fail to develop effective immunity from active vaccines. Also, mAbs tend to have low rates of adverse reactions and can confer immunity in a shorter time after administration. The uses of mAbs are widespread as therapies for cancer and autoimmune diseases. While current costs represent a major barrier to wider adoption, production costs are expected to decrease as production volume increases and manufacturing technology matures. Notably, the Bill & Melinda Gates Foundation has recently invested more than $20 million in a biotech company, Achaogen, to accelerate the overall development of its mAbs discovery platform, in particular focusing on mAbs against the multidrug-resistant bacteria which are most problematic in low- and middle-income countries (LMICs) (Genetic Engineering & Biotechnology News, 2017). A number of mAbs are under development for S. aureus and P. aeruginosa, currently in phase I and II trials (Czaplewski et al., Reference Czaplewski, Bax and Clokie2016). A monoclonal antibody against C. difficile toxin B, Bezlotoxumab, was approved by the US Food and Drug Administration in 2017 for use in the prevention of C. difficile infection (CDI) recurrence in patients under 18. This has been shown to reduce the risk of CDI recurrence from 26–28% to 16–17% (Wilcox et al., Reference Wilcox, Gerding and Poxton2017). While C. difficile is not usually considered to be a pathogen where AMR is clinically important (as resistance does not impact on treatment), antibiotic resistance in C. difficile does occur and may significantly impact on the epidemiology and add to the burden of infection in settings where antibiotic use is high, as antibiotic-related disruption to the microbiome may provide an opportunity for C. difficile overgrowth. Notably, the large reductions in C. difficile infection that have been seen in England following multiple interventions have resulted primarily from reductions of fluoroquinolone-resistant strains (Dingle et al., Reference Dingle, Didelot and Quan2017). Modelling work suggests that use of a vaccine or monoclonal antibodies targeted to health-care settings might be expected to have a substantially larger impact on resistant C. difficile strains if these spread preferentially in hospitals (van Kleef et al., Reference van Kleef, Luangasanatip and Bonten2017).
The “ESKAPE” pathogens (Enterococcus faecium, Staphylococcus aureus, Klebsiella pneumoniae, Acinetobacter baumannii, Pseudomonas aeruginosa, and Enterobacter species) are responsible for some of the most severe AMR problems. For example, in recent years the emergence of Acinetobacter baumannii lineages resistant to almost all antibiotics capable of treating Gram-negative bacteria has caused considerable alarm (Evans, Hamouda & Amyes, Reference Evans, Hamouda and Amyes2013). In some parts of the world the emergence of lineages resistant to first carbapenems (hitherto the last remaining conventional treatment option) and then colistin has severely compromised the ability to treat infections with this organism. Recently, multidrug-resistant A. baumannii has been estimated to account for over 36 000 deaths annually in Thailand (where carbapenem resistance is widespread), 41% more than if the infection had not been multidrug-resistant (Lim et al., Reference Lim, Takahashi and Hongsuwan2016). This excess mortality makes this organism the dominant cause of multidrug-resistance-associated mortality in hospitalized patients in the country.
Similarly, multidrug-resistant Klebsiella pneumoniae has emerged in many healthcare settings as the leading cause of resistance-associated mortality and morbidity. For these reasons, carbapenem-resistant A. baumannii, and carbapenem-resistant, third-generation cephalosporin-resistant Enterobacteriaceae (which include K. pneumoniae), alongside carbapenem-resistant P. aeruginosa, were ranked in a recent World Health Organization (WHO) exercise as the pathogens of the highest priority where new antibiotics were needed – and, by extension, where the potential impact of vaccines could be greatest (World Health Organization, Reference Organization2017b). Unfortunately, with the exception of P. aeruginosa, there is little activity in developing vaccines or other immunotherapies for these pathogens that has extended beyond animal models and it is thought unlikely that these vaccines will be available within the next 10 years. Major technical hurdles to developing vaccines for these “ESKAPE” pathogens exist and comprise limited understanding of pathogen biology including natural immunity, limited knowledge of vaccine targets, the existence of multiple strains and a complex and poorly defined epidemiology (Wellcome Trust and Boston Consulting Group, Reference Trust and Group2018). Lack of animal models of clear clinical relevance also presents an important hurdle for preclinical work, while the relatively low incidence of infections with these pathogens would make clinical trials of vaccines targeting these organisms challenging. Even if vaccines could be developed, the major difficulty in identifying a target population where vaccination would be cost-effective would present a high barrier to uptake.
Escherichia coli
Infections caused by E. coli are a major cause of morbidity and associated antibiotic use. In particular, enterotoxigenic E. coli (ETEC) is a leading cause of diarrhoea in children in developing countries and is estimated to account for 9% of all deaths attributed to diarrhoea (Lozano et al., Reference Lozano, Naghavi and Foreman2012). Ciprofloxacin-resistant ETEC strains represent a major challenge for ETEC treatment strategies in some parts of the world (Begum et al., Reference Begum, Talukder and Azmi2016). While there are no licensed vaccines for ETEC (Bourgeois et al., Reference Bourgeois, Wierzba and Walker2016), vaccine development for ETEC is a World Health Organization priority. WC-rBS, a killed whole-cell vaccine, designed and licensed primarily to prevent cholera, has been recommended by some groups to prevent diarrhoea caused by E. coli, although a Cochrane review found insufficient evidence to recommend it for protecting against ETEC diarrhoea (Ahmed et al., Reference Ahmed, Bhuiyan and Zaman2013). There are, however, a number of ETEC vaccine candidates in development and currently undergoing phase II trials, with one of the most advanced candidates a tetravalent inactivated whole-cell vaccine, ETVAX. While antibiotics are not recommended for the routine treatment of diarrhoea, even for infections such as ETEC which might respond (World Health Organization, Reference Organization2005), in practice antibiotics are widely perceived as being the treatment of choice for diarrhoea in the community and were found to be used to treat about half of all episodes of diarrhoea in India and Kenya (Zwisler, Simpson & Moodley, 2013). The introduction of an ETEC vaccine could therefore potentially play an important role in reducing resistance primarily through its impact on reduced antibiotic consumption and therefore reduced bystander selection.
Urinary tract infections (UTIs) are the second most commonly seen infections in primary care (after respiratory tract infections). They account for a high volume of antibiotic usage in the community and represent a major economic and public health burden both of which are exacerbated by AMR (Flores-Mireles et al., Reference Flores-Mireles, Walker and Caparon2015). The leading cause of uncomplicated community-acquired urinary tract infection is uropathogenic E. coli (UPEC). AMR in this organism is a major and increasing concern in the treatment of such infections, particularly in developing countries (Bryce et al., Reference Bryce, Hay and Lane2016). There has been considerable effort in the development of UPEC vaccines for the treatment of recurrent or chronic UTIs (Barber et al., Reference Barber, Norton and Spivak2013; Brumbaugh & Mobley, Reference Brumbaugh and Mobley2012). However, the fact that prior UTI does not elicit a protective immune response suggests this might not be easy. Another significant challenge is the high diversity of the UPEC population and multiple virulence factors (with no single one necessary for UTI).
Salmonella typhi
Antibiotic resistance has recently emerged as a major problem in Salmonella typhi infection, with the global dissemination of a ciprofloxacin-resistant lineage that is associated with fluoroquinolone treatment failure (Wong et al., Reference Wong, Baker and Pickard2015; Pham Thanh et al., Reference Pham Thanh, Karkey and Dongol2016; Feasey et al., Reference Feasey, Gaskell and Wong2015). Two vaccines have been available since the 1990s and are recommended by the WHO: the live Ty21a vaccine and the Vi-polysaccharide vaccine (Anwar et al., Reference Anwar and Goldberg2014). Although both are effective in reducing typhoid fever, protection is partial and relatively short-lived, with Ty21 preventing one third to one half of typhoid cases in the first two years after vaccination and the Vi-polysaccharide vaccine preventing up to two thirds of cases in the first year after vaccination. Both vaccines have seen limited use in endemic countries and are mainly used to protect travellers to those countries. Several next-generation conjugate vaccines are in various stages of development, with two vaccine candidates having received licensure in India (Meiring et al., Reference Meiring and Gibani2017). With Gavi, the Vaccine Alliance, having opened a funding window for this vaccine, it could potentially see greater use in LMICs.
Viral pathogens
Vaccines against viruses may play an important role in tackling AMR. This may occur both through reducing bystander selection by decreasing antibiotic usage for syndromes that may have either viral or bacterial causes and which are frequently treated with antibiotics (fever, respiratory infection, diarrhoea), and through reducing secondary bacterial infections that are causally linked to initial viral infections (such as influenza infection).
Among currently available viral vaccines, influenza vaccines (including inactivated influenza vaccines and live attenuated influenza vaccines) may have the greatest potential impact on AMR, with some observational data to support this. In 2000, the Canadian province of Ontario introduced universal influenza immunization. This was associated with a doubling of vaccine uptake to 28% (Kwong et al., Reference Kwong, Maaten and Upshur2009). This increase in uptake was associated with declines in rates of respiratory antibiotic prescriptions for most classes of antibiotics. Influenza-associated antibiotic prescriptions were estimated to represent 2.7% of respiratory antibiotic prescriptions before universal influenza vaccination, but only 1.1% of prescriptions afterwards. In Canadian provinces where influenza vaccination policy did not change, no such reductions in antibiotic prescribing were observed. While 2.7% seems a relatively small contribution to total antibiotic use (suggesting modest bystander selection effects and only a small benefit of vaccination), the fact that such antibiotic use is clustered in space and time could make selection effects for resistance locally more intense than population averaged figures suggest. Another study has estimated that reducing influenza activity by 20% would reduce fluoroquinolone prescriptions by 8%, although again translating such reductions to potential impact on resistance is challenging. We might expect impacts on AMR to be particularly large if children are targeted for vaccination. This is both because children typically consume more antibiotics than adults and also because they tend to play a disproportionate role in the transmission of both influenza and bacterial pathogens.
Similar benefits may occur if a vaccine against RSV can be deployed. Currently, there is no licensed RSV vaccine but a number are undergoing clinical trials, including vaccines that are likely to be optimal for the paediatric population as well as some that are likely to be appropriate for pregnant women and the elderly (Esposito & Di Pietro, Reference Esposito and Di Pietro2016).
Vaccines against viruses causing diarrhoea may also lead to reductions in antibiotic use and reduced resistance by altering bystander selection. This may be important even though antibiotics are not usually recommended for treating diarrhoea, because, as noted above, in some parts of the world many caregivers still believe that antibiotics are effective at stopping diarrhoea, and inappropriate antibiotic treatment accounts for much of the costs of treating it (Zwisler et al., Reference Zwisler, Simpson and Moodley2013). The currently licensed rotavirus vaccines may therefore play an important role, as they protect against the most common cause of severe diarrhoea in young children and prevent up to a third of severe diarrhoea cases in developing countries (World Health Organization, Reference Organization2013; Soares-Weiser et al., Reference Soares-Weiser, Maclehose and Bergman2012). A vaccine against norovirus would also be useful; norovirus accounts for nearly 20% of all cases of acute gastroenteritis which causes the second greatest burden of all infectious diseases globally (Ahmed et al., Reference Ahmed, Hall and Robinson2014). Currently, there is no licensed norovirus vaccine, but two candidate vaccines have reached clinical trials and there are a number of candidates at preclinical development stages (Cortes-Penfield et al., Reference Cortes-Penfield, Ramani and Estes2017).
Quantifying the economic benefit of vaccines that prevent antimicrobial resistance
The value for money of a vaccination programme can be estimated using an economic evaluation such as a cost–effectiveness analysis, which considers the balance between the incremental costs and incremental health impacts of an intervention. Hence, a vaccination programme will have a greater health impact and so will appear more cost-effective if it succeeds in reducing the absolute incidence of an antimicrobial resistant pathogen and/or the prevalence of resistance in the pathogen. AMR reduction is likely to be a key benefit of vaccines such as those against H. influenzae serotype b (Bärnighausen et al., Reference Bärnighausen, Bloom and Canning2011). Yet a recent review of published models of the impact of vaccines on the dynamics of AMR did not find any studies that considered the economic value of this benefit (Atkins et al., Reference Atkins, Lafferty and Deeny2018).
The benefit of vaccination in reducing AMR is normally seen in terms of avoiding the additional cost and increased health detriment incurred as a result of being infected by a resistant strain. This additional burden has been measured through matched cohort studies or regression models that compare the length of stay and treatment costs of patients infected with susceptible versus resistant microorganisms (Cohen et al., Reference Cohen, Larson and Stone2010). Hence, the simplest way to estimate the benefit of vaccination is to multiply the reduction in risk of acquiring a resistant strain in vaccinated individuals with the health detriment and financial cost of being infected with such a strain.
However, there are a number of ways in which this simple model of economic benefit may be too limited to comprehensively capture the value of a vaccine in reducing AMR. Firstly, public health interventions against infectious diseases may have wider ecological externalities on the community. For example, vaccines can confer protection not just on the people who have been vaccinated but also on other people who may otherwise have been infected by them (herd protection). Reduction of antimicrobial resistance through a number of pathways (see Introduction) is a further positive ecological externality of vaccines. Hence the benefit of vaccination should be measured not simply in individuals protected, but in the indirect effects in preventing both transmission and also reduction in AMR through several routes.
Second, several reviews of the economic benefits of vaccines have pointed out that economic analyses often overlook the wider benefits of vaccination on households and economies (Jit et al., Reference Jit, Hutubessy and Png2015). This is particularly relevant in terms of prevention of AMR. The wider economic cost of AMR can be quite substantial when considering reductions in productivity from patients infected with resistant pathogens, the need to spend research and development funds in developing new antimicrobials because of resistance to existing antimicrobials, and the worst-case scenario of being unable to perform routine medical procedures such as surgery because of untreatable surgical site infections (Smith & Coast, Reference Smith and Coast2013).
One further issue is that the scope of the positive externalities of vaccines in this regard may be global, since avoiding the development of resistance is a global benefit to all countries. However, the actors in the vaccine market are usually individual countries or even private individuals. Hence the full externalities of vaccination may not be adequately priced in a completely free market, discouraging manufacturers from developing vaccines that have benefits in reducing resistance. This market failure could be corrected by international cooperation and pooling of resources to encourage the development of vaccines with additional benefits in terms of AMR reduction. Mechanisms used in the vaccine world such as the Advanced Market Commitment for pneumococcal vaccines as well as pooled procurement by organizations such as Gavi and UNICEF offer models of this (De Roeck et al., 2006).
The importance of accurately capturing the value of vaccines against AMR
The vaccine development pipeline has been a busy one over the past few decades, and there are a number of vaccines that are currently being developed or trialled. As the number of vaccines in recommended schedules has increased, so has the cost of vaccination. For instance, the cost of fully vaccinating a child with all of the WHO’s recommended vaccines has risen from $0.67 in 2001 to $45.59 in 2014 (Médecins Sans Frontières, Reference Frontières2015). As a result of the high cost of both procuring and delivering vaccines, vaccine access faces challenges particularly in low- and middle-income countries. For instance, there was a 12-year gap between the first high-income country introduction of hepatitis B vaccines and the first introduction in a low-income country (Gavi Alliance, Reference Alliance2012). Gavi was set up in 2000 as a public–private partnership in order to address this access gap. However, most middle-income countries, including some of the highest users of antibiotics, are not eligible (van Boeckel et al., Reference van Boeckel, Gandra and Ashok2014). Vaccine purchasers in middle-income countries need strong economic rationales to introduce new vaccines in the face of many competing health priorities. Hence, establishing the value proposition for vaccine development and use has become increasingly important.
Such introduction decisions are market signals for vaccine researchers and manufacturers to prioritize developing particular products and maintaining particular product lines over others (Robbins & Jacobson, Reference Robbins and Jacobson2015). Adequate incorporation of the full value of vaccines in preventing AMR will provide the right incentives for development of vaccines which target resistant organisms. This can also guide the decision over which groups to vaccinate. For example, pipeline vaccines against common hospital-acquired bacterial infections such as S. aureus and C. difficile may be prioritized for people most likely to receive antibiotics.
Most vaccines are developed for their main disease impact, with their impact on AMR a secondary consideration. However, properly valuing this secondary benefit is important in prioritizing the vaccine development pipeline, as well as choosing between alternative interventions (such as a new vaccine and a new antibiotic). Furthermore, precautionary vaccines may be developed against organisms such as K. pneumoniae that are currently of relatively minor health importance but may potentially emerge as a more serious threat should drug-resistant strains become widespread. Population models of the interplay between microbiological, ecological and economic forces in a population or even in the whole world are needed to quantify this additional benefit of vaccines and to see whether it would alter the priority ordering of vaccines that need to be developed. Methods for incorporating the impact on AMR into standard economic evaluations of vaccines were recently described, although it was also acknowledged that further research is needed to accurately quantify the pathways linking vaccination with health and economic outcomes (Sevilla et al., Reference Sevilla, Bloom and Cadarette2018).
Areas for future research
To fully capture the value of vaccines in reducing AMR, three sets of pathways need to be quantified:
1) The health systems pathways governing the impact of vaccines on antimicrobial prescriptions. These may be best informed by evidence from clinical trials as well as retrospective studies using electronic databases, such as those linking influenza vaccination with reduced antibiotic prescribing (see the Viral pathogens section).
2) The epidemiological pathways governing the impact of vaccines on AMR (both directly and through reduced prescribing). A major research question is how reductions in antimicrobial use following vaccination will (or will not) translate into reductions in resistance. In some cases there is clear evidence from observational data that reductions in certain combinations of antimicrobials are associated with decreased AMR combinations (Bell et al., Reference Bell, Schellevis and Stobberingh2014). However, this relationship is far from fully understood, and there is a long way to go before the impact that reductions in antimicrobial use will have on the prevalence of resistance could be reliably predicted. The task is complicated by uncertainty around the fitness cost of resistance (i.e. the ability of a resistant organism to reproduce compared to a susceptible one), since measurements in the laboratory may not translate into real world settings and compensatory mutations may mean such fitness costs change over time. There is also uncertainty around the importance of genetic hitchhiking (resistance levels may rise not through any selective advantage, but as a result of fortuitous association with a successful and expanding lineage). From a modelling point of view, this step may require the use of dynamic transmission models that capture both direct and indirect effects of vaccines (Atkins et al., Reference Atkins, Lafferty and Deeny2018), including herd effects through reduction of transmission, acquisition of resistant genes and competition between susceptible and resistant strains of pathogen.
3) The economic pathways governing the value of reduced AMR. Quantifying these pathways will ideally make use of models that capture the macroeconomic potential of vaccines reducing AMR over long-term time horizons, incorporating counterfactual scenarios such as the need to continuously develop new antibiotics and antibiotic classes, as well as the risk that entire medical procedures may become riskier or even impossible.