Skeletal muscle fatigue, or the decline in expectant force development, is tightly coupled with oxygen supply and demand( Reference Barclay 1 – Reference Hogan, Arthur and Bebout 4 ). The ability of skeletal muscle to rapidly adjust developed force when oxygen is limiting or demand is increased is well established and associated with reduced muscle function without disturbance in tissue levels of ATP( Reference Hogan, Kurdak and Arthur 5 ). Therefore, efficient use of available oxygen is of upmost importance with regard to repetitive and sustained skeletal muscle force production.
Structural membrane composition directly affects cell function( Reference Gorjao, Azevedo-Martins and Rodrigues 6 ). When long-chain (LC) n-3 PUFA from dietary fish oil are incorporated into myocardial membranes( Reference McLennan, Howe and Abeywardena 7 – Reference Charnock, Abeywardena and Poletti 10 ), the function of the heart is enhanced, particularly when oxygen supply is compromised( Reference McLennan, Abeywardena and Charnock 8 , Reference McLennan, Bridle and Abeywardena 11 ). In skeletal muscle membranes, a similar process of incorporation occurs, whereby LC n-3 PUFA DHA concentrations are well above the dietary or circulating concentrations( Reference Charnock, Abeywardena and Poletti 10 , Reference Pan and Storlien 12 – Reference Peoples and McLennan 14 ). A number of key metabolic( Reference Clavel, Farout and Briand 15 ) and physiological( Reference Kamolrat, Gray and Thivierge 16 ) processes have been reported to be associated with the increased concentrations of LC n-3 PUFA DHA in skeletal muscle membranes.
Preliminary evidence has emerged that skeletal muscle oxygen consumption is modulated, as in the heart, after fish oil supplementation. In trained cyclists, dietary fish oil supplementation, delivering high concentrations of DHA, has been shown to reduce heart rate and whole-body oxygen consumption during prolonged submaximal cycling performance( Reference Peoples, McLennan and Howe 17 ). Skeletal muscle is the dominant consumer of oxygen during intense exercise, and this implies that the efficiency of oxygen use by the working muscles is enhanced. To directly test this hypothesis, oxygen consumption has been measured across autoperfused contracting hindlimbs( Reference Peoples, Hoy and Henry 18 ). Incorporation of DHA into skeletal muscle membranes results in oxygen cost-saving effects, as observed in the heart. However, oxygen consumption has been measured at only one time point in the fatigue process. Muscle fatigue commences from the initiation of force production, and in light of the critical role of oxygen supply and demand, this detailed relationship deserves further investigation.
No clear improvement in human fatigue tolerance has yet been demonstrated with dietary fish oil( Reference Buckley, Burgess and Murphy 19 – Reference Raastad, Hostmark and Stromme 21 ). This can be attributed either to varying n-3 PUFA doses and durations of supplementation( Reference Mickleborough 22 ) or to fatigue measures with poor reliability and sensitivity. The latter can largely be ascribed to the focus of research on potential cardiac, peripheral vascular or haematological mechanisms for improved exercise endurance. In other words, whole-body exercise tolerance is multifactorial, and unless careful design is employed, the many underlying mechanisms as to why one cannot sustain exercise, including the properties of skeletal muscle itself, cannot be resolved. Specifically, with regard to our oxygen modulation hypothesis, neither human( Reference Peoples, McLennan and Howe 17 ) nor animal( Reference Peoples and McLennan 14 ) studies have primarily been designed to directly examine the effects of LC n-3 PUFA on muscle endurance, focusing instead on cardiac endurance and on the recovery of skeletal muscle contractile force between repeat contractile bouts. Moreover, a previous study has employed a muscle stimulation rate too slow and of insufficient duration to elicit a major depression in skeletal muscle force production and thus enable an objective measure of muscle fatigue.
To date, no attempt has been made to investigate the direct impact of fish oil supplementation and specifically incorporation of LC n-3 DHA into skeletal muscle membranes on prolonged contractile fatigue resistance. Therefore, the aim of the present study was to use the autologous blood-perfused hindlimb method in rats( Reference Peoples, Hoy and Henry 18 , Reference Hoy, Peoples and McLennan 23 ) to investigate the effects of dietary LC n-3 PUFA, and specifically DHA, on skeletal muscle fatigue resistance including measuring oxygen consumption at multiple time points of force depression. It was hypothesised that the increased efficiency of skeletal muscle oxygen consumption, at multiple time points, would be expressed as an attenuation of muscle force depression over a sustained bout of contraction eliciting fatigue.
Experimental methods
In total, eighteen young male Wistar rats (400–500 g; 18–20 weeks of age) were used for the study and were evenly divided into groups of six per dietary intervention. The rats were housed two per cage in the animal facility of the University of Wollongong with temperature being maintained at 23–25°C under a 12 h light–dark cycle. Experiments were assessed and approved by the Animal Ethics Committee of the University of Wollongong, and all national and institutional guidelines were followed.
Diet composition
In the present study, three diets (saturated fat (SF), n-6 PUFA and n-3 PUFA) were prepared. All the diets contained a balanced mix of macro- and micronutrients to avoid any nutrient deficiencies based on the AIN-93M diet( Reference Reeves, Nielsen and Fahey 24 ). In brief, the diets were designed to deliver (1) similar total PUFA amounts in the n-6 PUFA (36·65 g/kg) and n-3 PUFA (31·40 g/kg) diets, (2) similar n-6 PUFA amounts in the n-3 PUFA (5·01 g/kg) and SF (4·77 g/kg) diets and (3) similar n-3 PUFA amounts in the n-6 PUFA (0·49 g/kg) and SF (0·58 g/kg) diets. Olive oil was used as a light (refined) oil; thus, the diets were free of confounding sources of natural antioxidants; beef tallow was used as saturated fat; safflower oil as a source of n-6 PUFA; high-DHA tuna fish oil as a source of n-3 PUFA (α-linolenic acid 0·56 g/kg, EPA 4·87 g/kg and DHA 20·2 g/kg) (Nu-Mega Ingredients). All the diets contained 10 % fat by weight. The proportions of fat sources have been reported previously( Reference Peoples and McLennan 14 ): the SF diet contained 30:70 olive oil and beef tallow; the n-6 PUFA diet contained 50:50 olive oil and safflower oil; the n-3 PUFA diet contained 30:70 olive oil and tuna oil. The diets were prepared from purified ingredients and stored at − 20°C( Reference Owen, Peter-Przyborowska and Hoy 25 ).
Study design
The rats were fed a washout diet containing olive oil for 14 d to standardise muscle membrane fatty acid composition. The eighteen rats were then randomly allocated to three groups and fed the SF, safflower oil (n-6 PUFA) or tuna fish-oil (n-3 PUFA) diet for 8 weeks (ad libitum). Fresh food was provided twice per week, and daily intake was estimated by weighing the remainder from the previous feeding. The weight of the rats was measured once per week.
Surgical preparation for ventilation, muscle perfusion and stimulation
The autologous pump-perfused hindlimb method has been described previously in both canines( Reference Hogan, Gladden and Grassi 26 ) and rats( Reference Peoples and McLennan 14 , Reference Hoy, Peoples and McLennan 23 ). In brief, rats (18–20 weeks) were anaesthetised (sodium pentobarbital 6 mg/100 g intraperitoneally) with supplementary injections (2 mg/100 g intraperitoneally) as required. The experiments were carried with each of the rats and the perfusion system being enclosed in a heated Perspex chamber (32·2 ± 0·3°C) with the body temperature of the rats being maintained at 37°C (rectal temperature). The specific diet group that each rat belonged to was blinded to the scientist carrying out the procedure. The rats were prepared for artificial ventilation (1 ml/100 g body weight, Rodent Ventilator 7025; Ugo Basile) and blood pressure recording by intubation of the trachea and cannulation of the left carotid artery, respectively.
All cannulae were fluid filled with saline containing 6 % dextran (w/v) and 5000 IU heparin/100 ml (Sigma-Aldrich). Arterial (oxygenated) blood was accessed via a cannula inserted into the right femoral artery (non-perfused leg) towards the heart and was connected to a resealing flexi-tube, passed through a peristaltic roller pump (Minipuls 3; Gilson). Arterial blood was then passed through a cannula inserted into the left femoral artery (perfused leg) and positioned to deliver blood directly to muscle groups below the knee. A T-junction inserted in the blood flow line on the perfused hindlimb side of the pump was connected to a pressure transducer (Argon CDXIII; Maxim Medical) for the measurement of hindlimb perfusion pressure. The return of deoxygenated venous blood to the heart and lungs was achieved via passive flow through a cannula inserted into the left femoral vein of the perfused leg and the right jugular vein.
Hindlimb muscle contraction was achieved via sciatic nerve stimulation. A bipolar electrode (F-B5EI; Grass Technologies) was placed under the nerves for direct electrical stimulation of the trunk supplying the muscles of the gastrocnemius–plantaris–soleus muscle bundle. The nerve was crushed above the stimulation point to prevent retrograde conduction, standard practice in hindlimb experiments( Reference Stainsby and Renkin 27 ). The gastrocnemius–plantaris–soleus muscle group tendons were tied with non-compliant silk and connected to a force transducer (FT03C; Grass Technologies). Saline-soaked gauze was placed over the muscles to prevent drying.
Hindlimb perfusion and stimulation protocol
The hindlimbs of the rats were perfused for 30 min at 1 ml/min with no stimulation to allow for perfusion pressure to reach steady state (approximately 100 mmHg), and then baseline arterial and venous blood samples (200 μl) were drawn. The flow rate was reset and maintained at 2 ml/min during a single 30 min repetitive twitch stimulation bout (2 Hz, 7–12 V, 0·05 ms) to the sciatic nerve. Venous samples were collected throughout the stimulation bout period at time points 30 s, 60 s, 2·5 min and 5 min and then every 5 min until 30 min.
Measurements
Blood pressure and twitch force
Data were referenced to ground and amplified (Onspot Australia). The data acquisition software (Labview for Windows; National Instruments) was used to collect perfusion pressure and twitch force data throughout the perfusion trials (sampling rate 200 Hz).
Twitch characteristics were analysed at time points corresponding to blood sampling. Developed tension and other characteristics were averaged from ten consecutive contractions. Peak tension was defined as the highest developed force in each twitch curve. Maximal peak twitch tension was defined as the mean tension over the ten highest peak tensions. The first derivative of developed tension was used to determine the maximum rate of developed tension and relaxation. The area under the twitch curve (tension–time index) was calculated relative to peak tension at the point of initial contraction (first ten contractions), at the point of maximum contraction (maximal ten contractions) and after 30 min of contraction (final ten contractions).
Blood samples
Arterial and venous blood samples (200 μl) were collected via resealing silicone sections of the cannulae located proximal and distal to the hindlimbs. Of the 200 μl blood sampled, 80 μl were used for the measurement of PO2, PCO2, Na+, K+, pH and Hb using the laboratory blood gas and electrolyte machine (ABL77; Radiometer). The remaining 120 μl were spun down on a benchtop microcentrifuge (Millipore), and the plasma was removed and frozen for later analysis of blood glucose and lactate. Erythrocytes from the plasma samples were resuspended in an equal volume of saline and re-injected into the venous side of the perfusion. Hb concentrations were maintained above 12·5 g/100 ml whole blood throughout the experiments.
Muscle samples
Control muscle samples (gastrocnemius, plantaris and soleus) were collected from the right (non-perfused) leg immediately before the right femoral artery cannulation to be used as within-animal control tissue. At the completion of each trial and while the blood was still flowing, left leg (perfused and contracted) muscle samples (gastrocnemius, plantaris and soleus) were rapidly extracted. All the muscles were freeze-clamped and stored ( − 80°C) for later analysis. Soleus muscle was sampled as red/oxidative tissue and gastrocnemius muscle was sampled as white/glycolytic tissue. Additional muscle samples (deep posterior tibial, extensor digitorum longus and tibialis anterior) from the perfused hindlimbs were collected to determine the total wet weight of the skeletal muscle involved in the perfusion.
Statistical analysis
Two-way ANOVA was conducted with diet and time main effects and diet × time interaction using Statistix, version 8 (Analytical Software). Individual dietary pairwise comparisons were made using a corrected Bonferroni post hoc analysis for multiple comparisons of individual means. α was set at P< 0·05. Data are expressed as means with their standard errors.
Results
Food consumption and body weight
There was no significant difference in the quantity of food consumed between the dietary groups at either the start or the end of the trials or in the mean daily intake (SF: 6·68 (0·20 sem) g/100 g body weight per d; n-6 PUFA: 6·54 (0·15 sem) g/100 g body weight per d; n-3 PUFA: 6·37 (0·13 sem) g/100 g body weight per d) over the 8-week feeding period. The mean body weight of the n-6 PUFA group (544 (35 sem) g) was higher than that of the SF (465 (28 sem) g) and n-3 PUFA (472 (34 sem) g) groups (P< 0·05). There were no significant differences in the wet weight of the gastrocnemius–plantaris–soleus muscle bundle, which represented the contracting skeletal muscle bundle, between the dietary groups (SF: 3·08 (0·21 sem) g wet weight; n-6 PUFA: 2·93 (0·17 sem) g wet weight; n-3 PUFA: 2·51 (0·23 sem) g wet weight).
Baseline perfusion
Stable baseline conditions were achieved in all the dietary groups (Table 1). Rectal temperature was equally well maintained during all the surgical procedures and experimental conditions in all the dietary groups (SF: 37·0 ± 0·9°C; n-6 PUFA: 36·7 ± 0·6°C; n-3 PUFA: 36·0 ± 0·7°C). There was no significant difference in hindlimb perfusion pressure following 30 min of controlled flow (1 ml/min) (SF: 109 (11 sem) mmHg; n-6 PUFA: 102 (3 sem) mmHg; n-3PUFA: 106 (6 sem) mmHg). In all the dietary groups, artificial ventilation (SF: 151 (12 sem) ml/min; n-6 PUFA: 172 (13 sem) ml/min; n-3 PUFA: 147 (11 sem) ml/min) and resulting PaO2 were sufficient to achieve approximately 98 % oxygen saturation of erythrocytes and corresponding high content of arterial oxygen (approximately 19–20 ml/100 ml). Baseline venous PO2 was significantly lower than arterial PO2 (P< 0·05), demonstrating basal oxygen consumption by the resting muscle bundle. There was no effect of diet on the (a − v) O2 difference or basal oxygen consumption. There were no differences in the basal blood lactate or glucose concentrations between the dietary groups.
Table 1 Baseline (non-contracting) arterial and venous blood gas and resulting oxygen consumption following 30 min of perfusion at a constant flow rate (1 ml/min)* (Mean values with their standard errors, n 6 per group)

SF, saturated fat; PaO2, arterial O2 partial pressure; PaCO2, arterial CO2 partial pressure; CaO2, arterial content of O2; HCO3, carbonate concentration; PvO2, venous O2 partial pressure; PvCO2, venous CO2 partial pressure; CvO2, venous content of O2; (a− v) O2, arterial venous O2 difference; VO2, O2 consumption.
* There were no significant differences between the dietary groups for variables measured at baseline (P>0·05).
Tension development and oxygen consumption
In all the dietary groups, twitch tension increased from the tension developed in the first twitch to reach maximum twitch tension after about 150 contractions (60–80 s). Peak twitch tension then gradually declined over the course of 30 min repetitive stimulation bouts (Fig. 1(a); P< 0·05). The n-3 PUFA group developed significantly greater maximum twitch tension and attained greater peak tensions throughout the stimulation period (P< 0·05). There was no difference in time from initial twitch to the twitch of maximum tension (Fig. 2(a)) or in time from the twitch of maximum tension to the twitch of 80 % maximum tension between the dietary groups (Fig. 2(b)). However, in the n-3 PUFA group, the peak twitch tension took longer time to decline to 50 % of the maximum compared with that in the SF and n-6 PUFA groups, with the difference being significantly different from that in the n-6 PUFA group (P< 0·05).

Fig. 1 Effect of dietary fat on mean contractile and blood measures over time in the gastrocnemius–plantaris–soleus muscle bundle during 30 min repetitive sciatic nerve stimulation bouts (2 Hz, 7–12 V, 0·05 ms). (a) Isometric twitch tension, (b) oxygen consumption, (c) efficiency index (tension developed per mol O2 consumed), (d) blood lactate concentrations (symbols represent femoral venous lactate concentrations and horizontal dashed line represents arterial lactate concentrations) and (e) femoral venous pH. Time 0 represents prestimulation and is not shown for twitch tension or efficiency index. Values are means (n 6 per group), with their standard errors represented by vertical bars which are omitted when smaller than the symbol. * Mean values were significantly different between the dietary groups (P< 0·05). † Mean values were significantly different within the dietary groups over time (P< 0·05). , Saturated fat diet;
, n-6 PUFA diet;
, n-3 PUFA diet.

Fig. 2 Effect of dietary fat on (a) time from the first twitch to the maximum peak twitch tension, (b) time for peak twitch tension to decline to 80 and 50 %, respectively, of the maximum (max) peak twitch tension. Values are means (n 6 per group), with their standard errors represented by vertical bars. * Mean value was significantly different from that of the n-6 PUFA dietary group (P< 0·05). † Mean value was significantly different from that of the saturated fat (SF) dietary group (P< 0·1).
Oxygen consumption increased with time during the repetitive stimulation bouts in all the groups (P< 0·05 for time) and stabilised after about 10 min of contractions (Fig. 1(b)). It was very similar in all the dietary groups for the first 5 min of contractions. Beyond this time, the oxygen consumption of the n-6 PUFA and SF groups stabilised, whereas that of the n-3 PUFA group continued to increase before stabilising significantly higher after 15 min (P< 0·05 for diet; Fig. 1(b)). The decline in peak twitch tension coupled with the increased oxygen consumption led to a decrease in the efficiency index over time, independent of diet (P< 0·05; Fig. 1(c)). The higher oxygen consumption in the n-3 PUFA group was associated with greater peak twitch tension in the n-3 PUFA group throughout the stimulation period and a significantly higher efficiency of oxygen utilisation (P< 0·05; Fig. 1(c)).
The twitch characteristics such as rise time, fall time, contraction duration and maximum rates of tension development and relaxation increased over the duration of the contraction bouts (P< 0·05; Fig. 3). However, the n-3 PUFA diet attenuated the changes in twitch characteristics, resulting in significantly faster twitch development and relaxation rates and shorter rise time, fall time and twitch duration compared with either the n-6 PUFA or SF diet across the 30 min of contraction bouts (P< 0·05).
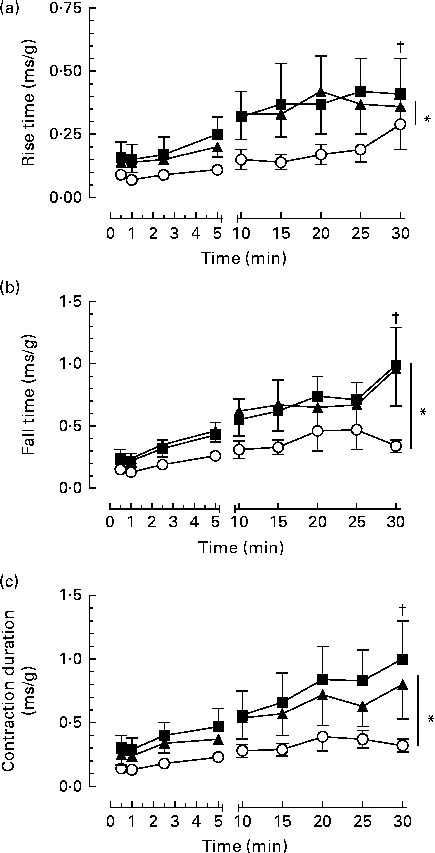
Fig. 3 Effect of dietary fat on contractile function over time in the gastrocnemius–plantaris–soleus muscle bundle during 30 min repetitive sciatic nerve stimulation bouts (2 Hz, 7–12 V, 0·05 ms). (a) Relative rise time (time to increase from 10 to 90 % maximum developed tension), (b) fall time (time to decrease from 90 to 10 % maximum tension) and (c) contraction duration (at 50 % maximum tension). Values are means (n 6 per group), with their standard errors represented by vertical bars which are omitted when smaller than the symbol. * Mean values were significantly different between the dietary groups (P< 0·05). † Mean values were significantly different within the dietary groups over time (P< 0·05). ■, Saturated fat diet; ◆, n-6 PUFA diet; ○, n-3 PUFA diet.
Blood lactate, blood glucose and muscle glycogen
Femoral venous blood lactate concentrations increased above the resting levels (P< 0·05) during repetitive muscle contractions and venous pH decreased (P< 0·05) (Fig. 1(d) and (e)). Lactate concentrations increased rapidly initially (within 60 s) and continued to increase gradually throughout the stimulation period. Venous lactate concentrations and pH changes were augmented in the n-3 PUFA group and were significantly different from those in both the n-6 PUFA and SF groups (P< 0·05).
There were no differences in arterial glucose concentrations (SF: 5·8 (0·2 sem) mmol/l; n-6 PUFA: 5·7 (0·3 sem) mmol/l; n-3 PUFA: 6·1 (0·3 sem) mmol/l) or femoral venous glucose concentrations (SF: 5·5 (0·2 sem) mmol/l; n-6 PUFA: 5·5 (0·3 sem) mmol/l; n-3 PUFA: 6·0 (0·3 sem) mmol/l) between the dietary groups at rest. Femoral venous glucose concentrations did not change throughout the contraction period (30 min) (SF: 4·8 (0·5 sem) mmol/l; n-6 PUFA: 5·4 (0·5 sem) mmol/l; n-3 PUFA: 5·7 (0·6 sem) mmol/l). Glycogen concentrations in the stimulated muscle after 30 min of repetitive contractions were lower than those in the control muscle (P< 0·05), and yet they were not affected by diet in either the control gastrocnemius muscle (SF: 9·33 (1·09 sem) mg/g wet weight; n-6 PUFA: 9·19 (0·46 sem) mg/g wet weight; n-3 PUFA: 8·78 (0·70 sem) mg/g wet weight) or the stimulated gastrocnemius muscle (SF: 6·93 (1·19 sem) mg/g wet weight; n-6 PUFA: 6·11 (0·64 sem) mg/g wet weight; n-3 PUFA: 5·78 (0·67 sem) mg/g wet weight) following 30 min of contractions. The results obtained for glycogen were reflected in both the soleus and the plantaris muscle (data not shown).
Discussion
In rats fed fish oil, skeletal muscle was resistant to fatigue during repetitive twitch contractions in vivo, extending the original observations of the modulation of VO2 in skeletal muscle by fish oil during bouts of contraction and recovery( Reference Peoples and McLennan 14 ), and in the myocardium( Reference Pepe and McLennan 28 ). This represents an increased efficiency of contraction-dependent oxygen use over a range of force production with no dietary differences in basal VO2, similar to that observed in the K+-arrested quiescent heart( Reference Pepe and McLennan 28 ).
The fatigue process could be clearly divided into an early phase of rapid decline in twitch tension achieved with consecutive contractions followed by a slow phase. In the n-3 PUFA rats, the early phase was characterised by greater contractile force and a reduced rate of fatigue with no extra cost of VO2 (increased O2 use efficiency). In contrast, there was little difference in efficiency in the later phase of fatigue between the dietary groups, where higher contraction force in the n-3 PUFA rats was matched by higher VO2 and more rapid force development and relaxation. The early rapid fatigue during low-frequency stimulation (in which oxidative metabolism predominates)( Reference Fitts 29 ) has been attributed to metabolic changes( Reference Baker, Carson and Miller 30 ), which may in turn be attributable to the accumulation of inorganic phosphate (Pi) observed in a similar preparation of hindlimb muscle used in the present study. The accumulation of Pi reduces Ca2+ sensitivity and precipitates CaPi to reduce sarcoplasmic reticulum Ca2+ release. The secondary slowing of contraction and relaxation suggests retarded removal of Ca2+ from the cytoplasm, which may be attributable to poor energy efficiency powering the sarcoplasmic reticulum ATPase or inhibition by Pi or reactive oxygen species( Reference Allen, Lamb and Westerblab 31 ). Reduced rates of calcium release from the sarcoplasmic reticulum into the cytosol and re-uptake by the sarcoplasmic reticulum Ca2+-ATPase are implicated in muscle fatigue( Reference Favero 32 ), and in the present study, DHA protection against the fatigue-related slowing of relaxation time in turn implicates attenuation of the impairment of cytoplasmic Ca2+ re-uptake( Reference Verberg, Thorud and Eriksen 33 ). There is a parallel with the altered cellular Ca2+ handling implicated in many of the effects of dietary fish oil in the heart( Reference McLennan and Abeywardena 34 ), including the modulation of excessive oxygen consumption and cardiac arrhythmias, both of which are usually associated with conditions of cellular sarcoplasmic reticulum and mitochondrial Ca2+ overload( Reference Pepe and McLennan 28 , Reference Pepe, Tsuchiya and Lakatta 35 ). The increased efficiency has a further parallel to the reduced whole-body VO2 throughout the prolonged exercise bouts in trained cyclists supplemented with fish oil( Reference Peoples, McLennan and Howe 17 ) and improved post-ischaemic cardiac recovery in rats( Reference Pepe and McLennan 28 , Reference Abdukeyum, Owen and McLennan 36 ).
The optimal oxygenation and perfusion properties of the in vivo autologous hindlimb model( Reference Hoy, Peoples and McLennan 23 ), adapted from canine muscle fatigue experiments( Reference Stainsby and Welch 37 ), were important contributors to the observations made in the present study. Isolated muscle preparations are often compromised by non-physiological operating temperatures or by oxygen delivery impaired by low oxygen-carrying capacity of crystalline perfusate and limited perfusion into large muscle bundles in vitro ( Reference Zhang, Bruton and Katz 38 ) and by abnormal accumulation of metabolites and electrolytes in the absence of perfusion. These methodological deficiencies could, therefore, explain the failure of an earlier study to observe the effects of dietary LC n-3 PUFA-induced changes in membrane composition on skeletal muscle function in vitro, except in relation to an essential fatty acid-deficient diet( Reference Ayre and Hulbert 13 ).
Fish oil feeding leads to the incorporation of LC n-3 PUFA DHA into the hindlimb skeletal muscle( Reference Peoples and McLennan 14 ). This is the principal n-3 PUFA incorporated into excitable tissues, including heart and skeletal muscles in humans and animals( Reference McLennan and Abeywardena 34 ), even when high-EPA fish oil or purified EPA is consumed( Reference McLennan, Howe and Abeywardena 7 , Reference Pepe and McLennan 9 , Reference Harris, Sands and Windsor 39 ). The similarities between the effects of fish oil on skeletal muscle and myocardial function and fatty acid composition suggest common underlying mechanisms. In the present study, the rise time and fall time of force production were found to be not slowed in the muscle of the n-3 PUFA group to the same extent as in that of the SF and n-6 PUFA groups. Therefore, the Ca2+ cycling process appears to be one potential mechanism of action of fish oil fatty acids in the function of excitable cells that may translate to skeletal muscle. Interestingly, the higher unsaturation index of skeletal muscle membrane phospholipids derived from dietary fish oil implies an increased risk of cellular oxidative damage, which might be expected to translate into poor contractile performance in line with the free-radical theory of fatigue( Reference Ferreira and Reid 40 ). However, similar to exercise training, which puts muscles under increased oxidative stress, yet preconditions against subsequent stress-induced cellular damage, the paradoxical fatigue resistance provoked by dietary fish oil suggests that similar to ischaemic preconditioning( Reference al Makdessi, Brandle and Ehrt 41 ) and nutritional preconditioning by LC n-3 PUFA in the heart( Reference Abdukeyum, Owen and McLennan 36 ) or exercise training( Reference Pansarasa, D'Antona and Gualea 42 ), the LC n-3 PUFA up-regulate endogenous protective mechanisms in skeletal muscle. However, future research will need to specifically address these aspects.
There was no evidence in the present study that diet-induced differences in substrate accumulation consistent with vigorous exercise were responsible for the observed contractile changes. Lactate production increased as muscles fatigued; however, as the venous concentrations were greatest in the n-3 PUFA tissues that fatigued least, these results support the assertion that lactate and hydrogen ion (H+) accumulation is not a major cause of fatigue( Reference Zhang, Bruton and Katz 38 ). Rather, lactate production, and subsequent decrease in pH, simply reflects the sustained contractile function of muscles and a greater extent of anaerobic metabolism( Reference Westerblab, Bruton and Katz 43 ). In turn, such changes to local metabolic conditions can enhance oxygen extraction as observed in fish oil-fed rats during the later phase of fatigue through a rightward shift in the oxygen–Hb dissociation curve, permitting greater oxygen unloading in the n-3 PUFA skeletal muscle, despite provision of a constant blood flow and arterial PO2. These results also further reflect the tight coupling of oxygen supply and demand where the efficient use of oxygen, in muscles with high DHA concentrations, will ultimately result in more prolonged and sustained force development.
A number of further questions can be explored with regard to the role of DHA in skeletal muscle membranes and fatigue. To date, studies on muscle contraction have been limited to twitch conditions. Although this is important for understanding a basic level of interaction between DHA and muscle function, there is further need to investigate the role of fish oil under physiologically relevant conditions of intermittent tetanic muscle contractions that demand a greater turnover of intracellular calcium. It is also intriguing to postulate that possible dietary fish oil preconditioning in skeletal muscle may allow preparation for stressful conditions where oxygen delivery may be depressed, such as hypoxia or peripheral ischaemia. Equally, given that epidemiological data now support the role of LC n-3 PUFA in the diet with respect to indices of maintained muscle function( Reference Abbatecola, Cherubini and Guralnik 44 ), there is good reason to further explore the potential for enhanced muscle physiology or simply attenuated decline by increasing DHA content in human diets. Most importantly, ‘low-dose’ fish oil, independent of background n-6 PUFA concentrations, can achieve equivalent membrane DHA incorporation in skeletal muscle( Reference Slee, McLennan and Owen 45 ), and this concentration is more than achievable in human diets (one to two meals of fatty fish per week).
In summary, using the rat in vivo autologous pump-perfused hindlimb model, in the present study, it was found that muscles sustained higher repeated contractile force after fish oil supplementation, which was objectively measured as an increased time to fatigue. Modulation of skeletal muscle oxygen consumption was evident across multiple time points through the fatigue process, associated with increased membrane DHA concentrations. With the exclusion of cardiac and vascular mechanisms that affect oxygen delivery, the increased oxygen efficiency coupled with attenuation of the fatigue-related prolongation of twitch duration and slowed recovery to baseline from peak twitch force points towards improved handling of calcium in the skeletal muscle cells. The present findings demonstrate that DHA is incorporated into skeletal muscle membranes to provide direct nutritional preconditioning to sustain muscle contractile performance. Preconditioning and improved muscle function have traditionally been associated with exercise training stimulus. However, in populations with skeletal muscle dysfunction precluding exercise training, such as the frail and elderly, the revelation of these potential benefits of dietary LC n-3 PUFA is exciting and points to future human applications.
Acknowledgements
The high-DHA fish oil was provided by Nu-Mega Ingredients.
The present study was jointly supported by an Australian Research Council – Strategic Partnerships with Industry – Research and Training (ARC-SPIRT) grant with Clover Corporation, Sydney, Australia. G. E. P. was supported by the ARC Key Centre for Smart Foods (University of Wollongong). None of the organisations had any role in the design and data analysis of the study or in the writing of this article.
The authors' contributions were as follows: G. E. P. and P. McL. contributed equally to all aspects of manuscript preparation.
None of the authors has any conflicts of interest.