INTRODUCTION
Human ancestors evolved in the most flammable biome on the planet—tropical savannas—and almost certainly have an evolutionary history shaped by fire (Parker et al., Reference Parker, Keefe, Herzog, O'Connell and Hawkes2016). Anthropogenic burning was likely a significant factor in ecological changes associated with the arrival of Homo sapiens to new environments in the Late Quaternary (McWethy et al., Reference McWethy, Whitlock, Wilmshurst, McGlone, Fromont, Li, Diffenbacher-Krall, Hobbs, Fritz and Cook2010; Bowman et al., Reference Bowman, Balch, Artaxo, Bond, Cochrane, D'Antonio and DeFries2011). Despite this close connection between people and fire, the ecological significance of landscape burning by Indigenous people in North America has often been controversial (Vale, Reference Vale2002; Roos et al., Reference Roos, Bowman, Balch, Artaxo, Bond, Cochrane and D'Antonio2014). In many cases, this has hinged on the ambiguity of distinguishing an anthropogenic signal in paleofire records that are already rich with fire (Roos et al., Reference Roos, Williamson and Bowman2019). In other cases, large-scale fire syntheses (regional to continental) often lack clear evidence of human impacts (Oswald et al., Reference Oswald, Foster, Shuman, Chilton, Doucette and Duranleau2020), but the linkage between these syntheses and the scales at which land-use behavior operated has not been established (Roos, Reference Roos2020).
Indigenous communities have lived in the fire-adapted forests of the North American Southwest (hereinafter “the Southwest”) for millennia, but their long-term legacies on Southwest fire histories is debated, because the climate is amenable to frequent fires and lightning is an abundant source of ignitions (Allen, Reference Allen and Vale2002). But more than just the presence or absence of fires, the ecological significance of Indigenous burning in landscapes with abundant nonhuman fire is likely to be subtle in terms of changes in burned area, fuels, and patch size (McWethy et al., Reference McWethy, Higuera, Whitlock, Veblen, Bowman, Cary and Haberle2013). Here we leverage prior archaeology, history, ethnography, and Apache oral traditions in a landscape geoarchaeology approach to identify spatial and temporal variability in fire regimes in the ponderosa pine (Pinus ponderosa) forests of east-central Arizona (Fig. 1). We use stratigraphic charcoal, pollen, extractable phosphorus (soil P), sedimentology, and sedimentation rates to infer changes in biomass burning, vegetation, and ash-related nutrients while controlling for the impact of depositional variability on these fire proxies (Nichols et al., Reference Nichols, Cripps, Collinson and Scott2000; Scott et al., Reference Scott, Cripps, Collinson and Nichols2000). Bayesian calibration of accelerator mass spectrometry radiocarbon dates and age–depth modeling allow us to compare fire regime proxies from four different periods that document different intensities of occupation by Ancestral Pueblo and Apache people: AD 1000–1400; AD 1400–1550; AD 1550–1870; AD 1870 to present.

Figure 1. The location of the Mogollon Rim study area, study watersheds, geoarchaeological localities, and modern analog sample sites relative to the distribution of ponderosa pine forests and the 2002 Rodeo-Chediski Fire and the 1974 Day Burn. Day Wash 16 (DaW 16) is a stratigraphic context that provides a “modern analog” for high-severity fire from the Day Burn.
Environmental context
We concentrate on ponderosa pine forests along the eastern Mogollon Rim in east-central Arizona that were home to ancestors of Pueblo and Western Apache people (Fig. 1). These forests span elevations between 1700 and 2300 m above sea level along the southern margin of the Colorado Plateau (Kaldahl and Dean, Reference Kaldahl, Dean, Mills, Herr and Van Keuren1999). Ponderosa pine forests are dominated by one canopy species, ponderosa pine, with rare contributions of various juniper (Juniperus spp.) and piñon (Pinus spp.) at lower elevations, and Douglas fir (Pseudotsuga menziesii) and Southwestern white pine (Pinus strobiformis) at higher elevations. Historically, understory plant communities were dominated by bunchgrasses (especially Festuca arizonica), forbs (especially those in Asteraceae and Amaranthaceae), and some shrubs, including various species of oak (Quercus spp., which can also grow in tree form as occasional codominants), sumac (Rhus trilobata), and manzanita (Arctostaphylos spp.). These forests are dotted by rare, small meadows (<25 ha) with a similar composition of grasses and forbs as were present in the historical understory.
Landscapes “below” the Mogollon Rim (i.e., to the south) receive more rainfall than those above due to the effect of orography. Average annual precipitation at Show Low, AZ, just above the Mogollon Rim, is 420 mm with strong bimodality of peak rainfall during the summer monsoon in July, August, and September and a second, smaller mode from cyclonic winter storms from the Pacific from October to March. April, May, and June are reliably dry, creating conditions each year when fires can ignite and spread. Average low temperatures are above freezing from April through October, the primary growing season for both wild plants and crops, although late (spring/summer) and early (summer/autumn) frosts are not uncommon (Kaldahl and Dean, Reference Kaldahl, Dean, Mills, Herr and Van Keuren1999).
Frequent, low-severity surface fires were historically ubiquitous in ponderosa pine and dry mixed-conifer forests in the Southwest until livestock grazing, logging, and fire suppression led to a near total cessation of wildfires beginning in the late nineteenth century (Swetnam and Baisan, Reference Swetnam, Baisan, Veblen, Baker, Montenegro and Swetnam2003; Swetnam et al., Reference Swetnam, Farella, Roos, Liebmann, Falk and Allen2016). Fire-scarred ponderosa pines can be tree-ring dated to the year and often to the portion of the growing season when the surface fires occurred (Dieterich and Swetnam, Reference Dieterich and Swetnam1984). Between ca. AD 1700 and AD 1900 (the period of greatest tree-ring sample depth), fires tended to occur in a stand once or twice per decade in surface fuels (grasses, needle litter, etc.) during the arid foresummer (April–June) or at the onset of the monsoon with dry lightning storms (July) (Fulé et al., Reference Fulé, Covington and Moore1997; Moore et al., Reference Moore, Covington and Fulé1999). Unpublished tree-ring records from the greater Mogollon region indicate that the region experienced unusually high frequencies of surface fire (three or more per decade) for at least two centuries before early twentieth-century fire suppression (Kaib et al., Reference Kaib, Swetnam and Morino2000).
Cultural context
As part of the larger movement to Indigenize the past, we embrace tribal knowledge that speaks to Indigenous presence in east-central Arizona since time immemorial. By underpinning our own research with goals that speak to tribal perceptions of their own cultural persistence in the Southwest, this is an acknowledgment of tribal sovereignty and self-determination necessary to understand and situate the past in the twenty-first century (Gonzalez et al., Reference Gonzalez, Modzelewski, Panich and Schneider2006). This is also an important recognition in the context of land-use and fire management histories, because Indigenous communities have developed and curated fire-keeping practices for generations and this knowledge is powerful, salient, and important today (Kimmerer and Lake, Reference Kimmerer and Lake2001; Lake et al., Reference Lake, Wright, Morgan, McFadzen, McWethy and Stevens-Rumann2017). By prioritizing indigenous perspectives on cultural and land-use history, we organize our understanding of the archaeology and ethnohistory in a way that emphasizes persistence while subdividing time to recognize variability in the intensity of use by different cultural groups.
We concentrate on Ancestral Pueblo (for some, the term “Mogollon Pueblo” is preferred; Riggs, Reference Riggs2010) and Western Apache land use after AD 1000, when widely distributed small villages, hamlets, and farmsteads appeared (Mills and Herr, Reference Mills, Herr, Mills, Herr and Van Keuren1999; Mills et al., Reference Mills, Herr, Kaldahl, Newcomb, Riggs, Van Dyke, Mills, Herr and Van Keuren1999a; Herr, Reference Herr2001). Although the settlements were small, this was the period of greatest population size in the region (Newcomb, Reference Newcomb, Mills, Herr and Van Keuren1999). Grinding stones and paleoethnobotanical remains all indicate a dependence upon farming (Huckell, Reference Huckell, Mills, Herr and Van Keuren1999). By the late AD 1200s, populations coalesced into a few large but widely separated pueblos (Kaldahl et al., Reference Kaldahl, Van Keuren, Mills, Adams and Duff2004). One of these (the Bailey Ruin) was located in the Day Wash area above the Mogollon Rim (Mills et al., Reference Mills, Van Keuren, Stinson, Graves, Kaldahl, Newcomb, Mills, Herr and Van Keuren1999b), and another (Tundastusa) in the Forestdale Valley below it (Haury, Reference Haury1985b; Riggs, Reference Riggs2010). Ethnographically and archaeologically, we know that Pueblo people used fire for myriad purposes, including burning to establish and clean agricultural fields and irrigation ditches; to promote wild plant resources for food, medicine, and craft materials; in game drives, to promote habitat for preferred game animals; and in religious pilgrimages and practices (Gifford, Reference Gifford1940; White, Reference White1943; Bohrer, Reference Bohrer1983; Sullivan and Mink, Reference Sullivan and Mink2018; Roos et al., Reference Roos, Swetnam, Ferguson, Liebmann, Loehman, Welch and Margolis2021). Although populations moved their perennial homes away from the area by AD 1400, Pueblo people have continued to maintain relationships with the Mogollon Rim region (Ferguson and Hart, Reference Ferguson and Hart1985).
The area is also home to Western Apaches, who were historically seasonally mobile forager-gardeners who lived in ponderosa pine forests and adjacent woodlands from spring through autumn as part of seasonal mobility patterns (Graves, Reference Graves and Reid1982). Apache Elders describe traditions of their presence in eastern Arizona since time immemorial (Welch and Riley, Reference Welch and Riley2001). Incorporating Apache oral tradition in reference to Apache presence in the Southwest since time immemorial not only sets a baseline for understanding Apache presence but also helps to foreground Apache interpretations of their own culture and histories that have often been constructed and interpreted by non-Apache researchers. While current interpretations based on archaeology (Herr, Reference Herr2013) and ethnohistory (Forbes, Reference Forbes1960) document intensive Western Apache use of the Mogollon Rim region by AD 1550, here we recognize oral traditions that Apaches were in the area at the same time as Ancestral Pueblo peoples. Western Apaches used fire in many activities, including those described for Ancestral Pueblo peoples (Griffin et al., Reference Griffin, Leone, Basso, Basso and Opler1971; Buskirk, Reference Buskirk1986). A large portion of the eastern Mogollon Rim region is within the Fort Apache Indian Reservation, which was established in 1870.
MATERIALS AND METHODS
In June and August 2005, we surveyed and sampled off-site alluvial stratigraphic sequences in two first-order watersheds along the Mogollon Rim of east-central Arizona for proxies of fire-related sedimentation and vegetation (Fig. 1). To explore the variable legacies of Indigenous fire use, we used existing archaeological data to select two watersheds that were likely to have both Ancestral Pueblo and Apache land-use histories. The Forestdale Valley is located south of Show Low, AZ, on the Fort Apache Indian Reservation and has been the subject of intensive archaeological research since the 1930s (Haury, Reference Haury1985c). The latest Ancestral Pueblo village in the Forestdale Valley, Tundastusa, was occupied until at least AD 1400, when Pueblo people migrated elsewhere (Haury, Reference Haury1985b; Riggs, Reference Riggs2010). The Day Wash watershed in Apache-Sitgreaves National Forest includes the late-occupied Bailey Ruin (ca. AD 1275–AD 1325) (Mills et al., Reference Mills, Van Keuren, Stinson, Graves, Kaldahl, Newcomb, Mills, Herr and Van Keuren1999b). Other archaeological evidence from near the Bailey Ruin and the Forestdale Valley indicate at least episodic, repeated settlement of these areas since ca. AD 200, with continuous, intensive occupation after AD 1000 (Haury, Reference Haury1985a [1940]; Haury and Sayles, Reference Haury and Sayles1985 [1947]; Mills, Reference Mills, Mills, Herr and Van Keuren1999; Mills and Herr, Reference Mills, Herr, Mills, Herr and Van Keuren1999; Roos, Reference Roos2005). Archaeological and ethnohistorical evidence documents intensive Western Apache use of the Mogollon Rim region, including the Forestdale and Day Wash areas, for at least the past 440 years (Basso, Reference Basso1998; Herr et al., Reference Herr, North and Wood2009).
Using our understanding of cultural persistence, we use archaeology, ethnohistory, and oral tradition to subdivide the past 1000 years into periods of varying land-use intensity. Period 1 (AD 1000–1400) represents a time of relatively intensive use by Ancestral Pueblo people as well as use by Apache groups. Period 2 (AD 1400–1550) represents a time of less intensive use of the area. Period 3 (AD 1550–1870) is a time of more intensive Apache use of the landscape. Period 4 (AD 1870 to present) represents more intensive use by settler colonists and general suppression of traditional land-use practices by the U.S. military, although some traditional practices persisted through this period (Watt, Reference Watt2015).
Day Wash and the Forestdale Valley are both small, first-order watersheds (the smallest stream category with no real tributary streams [Strahler, Reference Strahler1957]—less than 4000 ha in our case). Concentration on small, first-order discontinuous ephemeral streams means that most of the record is characterized by charcoal, pollen, and sediment accumulated from hillslope sources within the watershed rather than from storage in older alluvium, making these hyperlocal records of fire-derived sediments produced within these small basins (Bull, Reference Bull1997; Hereford, Reference Hereford2002). Both watersheds are generally low gradient and have similar bedrock geology dominated by nutrient-poor Cretaceous and Permian sedimentary rocks with average slopes between 5.2% (Day Wash) and 8.6% (Forestdale).
Incised reaches of targeted watersheds were surveyed on foot for exposed alluvium and soils that indicated somewhat rapid and recent accumulation (fluvent soils: alluvial soils with weakly developed soil morphology) (Birkeland, Reference Birkeland1999; Holliday, Reference Holliday2004). Narrow (ca. 1- to 2-m-wide) trenches were manually excavated into selected localities within the two watersheds: two in Day wash and four in the Forestdale Valley. Detailed analysis was done on one Day Wash locality (DaW 14), and two localities in Forestdale Valley (FDV 6 and 10; Fig. 2). Soil stratigraphic units were described in the field based on color, soil structure, bedding features, and boundary types using standard terminology (Schoeneberger et al., Reference Schoeneberger, Wysocki and Benham2012).
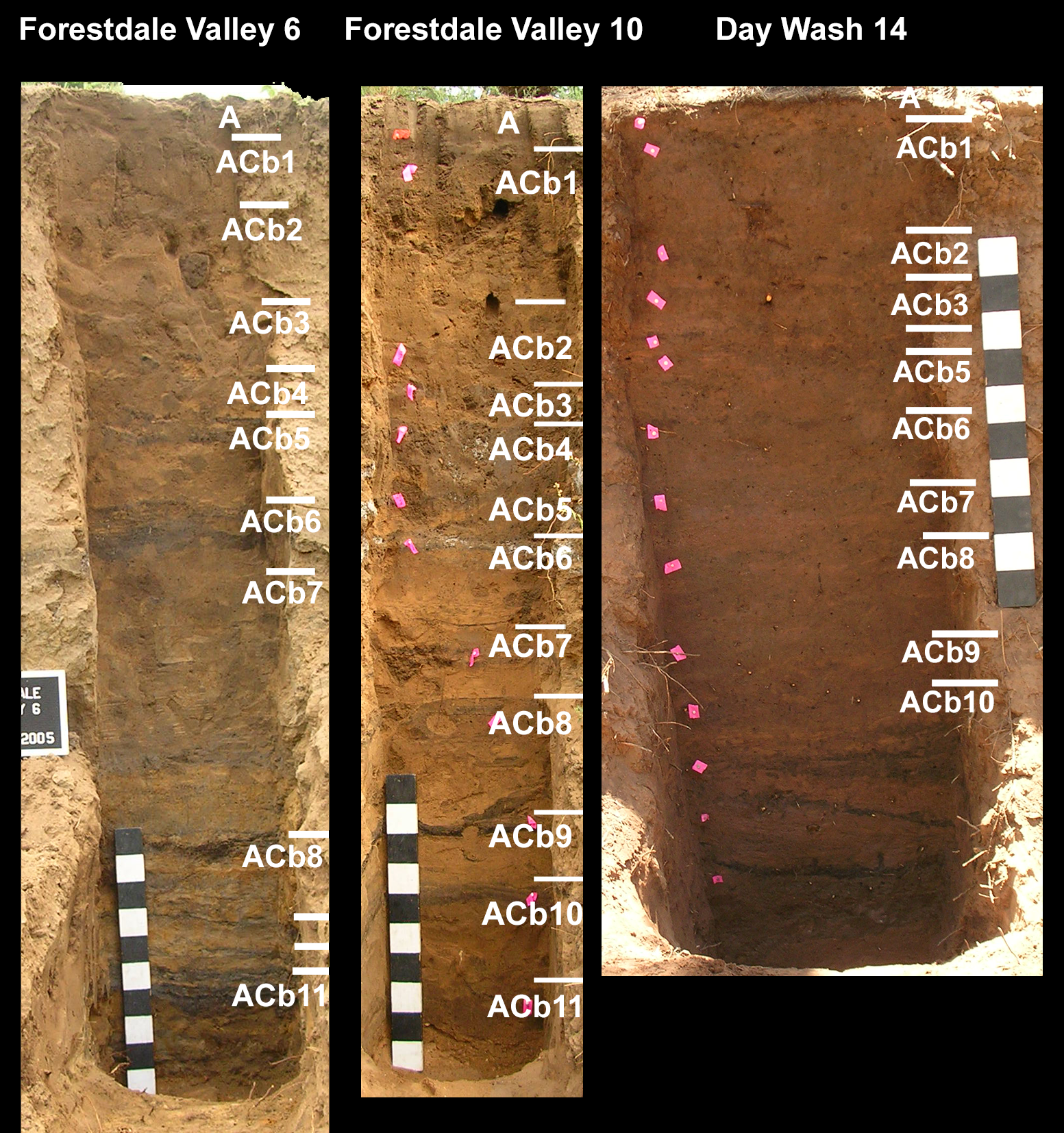
Figure 2. Photographs of exposed profiles at the three geoarchaeological localities annotated with soil stratigraphic boundaries and assignments. ACb9 and ACb10 are unlabeled in Forestdale Valley 6 (FDV 6), and ACb4 is unlabeled in Day Wash 14 (DaW 14).
Surface sediments from a channel fan/bar context in Rocky Draw in Apache-Sitgreave and a shallow, recent channel fan in Day Wash (DaW 16) were both sampled as “modern analogs” of fine-grained (mostly <2 mm grain size) channel fan sediments produced in depositional settings analogous to our ancient ones after the 2002 Rodeo-Chediski Fire and the 1974 Day Burn, respectively (Fig. 1). At ancient localities and modern analog sites, ca. 7- to 9-cm-thick undisturbed blocks were collected from pedogenic or depositional stratigraphic units for micromorphological analysis to supplement in-field descriptions of soil stratigraphic units (Stoops, Reference Stoops2003; Goldberg and Berna, Reference Goldberg and Berna2010).
At each intensively studied locality, bulk samples for the analysis of gravel content (>2 mm), grain-size distributions less than 2 mm (Janitsky, Reference Janitsky, Singer and Janitsky1986b), CaCO3 content (Machette, Reference Machette, Singer and Janitsky1986), and unburned soil organic matter content (SOM) (Janitsky, Reference Janitsky, Singer and Janitsky1986a) were collected from each described soil stratigraphic and sedimentary unit (Table 1). To simplify grain-size presentations, these data are presented as the ratio of fines (silt + clay; <63 μm) to sand (63 μm–2 mm). Continuous or overlapping monoliths of soil/sediment were collected in the field and sampled continuously at 2 cm intervals for sedimentary charcoal analysis (Whitlock and Larsen, Reference Whitlock, Larsen, Smol, Birks and Last2001). Five cubic centimeters of sample was disaggregated using 25 mL 10% Na-hexametaphosphate in 100 mL of deionized water, lightly oxidized using 25 mL of 3% H2O2 (Rhodes, Reference Rhodes1998), sieved with deionized water through a 250 μm steel sieve, and counted under a binocular microscope at 7–40× magnification. Raw counts of charcoal pieces >250 μm were converted to concentrations (counts/cm).
Table 1. Geoarchaeological locality measurements and samples.

Both watersheds are situated on nutrient-poor sedimentary bedrock and derived soils that are naturally low in phosphorus and other nutrients. Biological phosphorus volatilizes when combusted at more than 770°C, but at lower temperatures it oxidizes to phosphate where it adsorbs to soil mineral surfaces or forms new calcium phosphate minerals in the alkaline ash (DeBano et al., Reference DeBano, Neary and Ffolliott1998). Ash from low-intensity wildfires is an important source of phosphorus in low-P soil environments like those of the Mogollon Rim (Cowan et al., Reference Cowan, Smith and Fitzgerald2016). Mehlich II extractable soil P (a measure of ash-derived nutrient fluxes) was measured every 4 cm using a colorimetric method on the <125 μm fraction of monolith samples (Terry et al., Reference Terry, Hardin, Houston, Nelson, Jackson, Carr and Parnell2000).
Samples of sediment from the monoliths were collected for extracting and quantifying pollen from soil horizons using standard methods (Traverse, Reference Traverse2007). Pollen and fungal spores were identified using in-house comparative collections for the Southwest (Davis, Reference Davis2007). Pollen was generally well preserved but variably abundant. On average, deteriorated and indeterminate pollen grains made up 6.5% of individual samples (±6.6%). These grains (and any encountered wetland taxa) were not included in the terrestrial pollen sum. Pollen concentrations were generally low and variable, so we restrict our interpretations to the relative abundances of two major categories: undifferentiated Pinus, and grasses and ruderals (Amaranthaceae + Asteraceae + Poaceae). Samples with pollen grain counts below 50 are displayed in figures as gray dots but not included in our pollen interpretations. General vegetation interpretations are guided by pollen values for reference studies of modern ponderosa pine forests and meadows (Martin, Reference Martin1963; Rankin, Reference Rankin, Stafford and Rice1980; Hevly, Reference Hevly and Gumerman1988). These indicate that ponderosa pine forests have 40–80% pine pollen and 10–30% grass and ruderal pollen, while meadows typically have lower Pinus pollen (15–60%) and more abundant pollen of grasses and ruderals (50–80%). The dung fungus Sporormiella is reported as a percentage of the terrestrial pollen sum and is interpreted as a proxy for herbivore biomass (Davis, Reference Davis1987). Table 2 identifies potential interpretations of variability in these major proxy categories.
Table 2. Paleoecological proxies and possible interpretations.

Individual pieces of charcoal for radiocarbon dating were collected from the disaggregated and sieved charcoal samples. When possible, non-wood xylem tissues were preferred for dating. Radiocarbon dates were calibrated using a Bayesian algorithm in BCal (Buck et al., Reference Buck, Kenworthy, Litton and Smith1991, Reference Buck, Christen and James1999) that incorporated stratigraphic relationships between samples as prior information. Charcoal in alluvium generally provides terminus post quem ages (“date after which”) (Brown, Reference Brown1997), so unusually old dates that did not fit the rest of the population were excluded from Bayesian calibrations. All standard and Bayesian calibrations were done using IntCal20 (Reimer et al., Reference Reimer, Austin, Bard, Bayliss, Blackwell, Bronk Ramsey and Butzin2020) and are reported at 2σ and 95% highest probability density (hpd) for Bayesian date ranges (Table 3). Age–depth models were calculated in CLAM (Blaauw, Reference Blaauw2010) using the Bayesian 95% hpd ranges to assign soil stratigraphic units to land-use time periods and to generate estimated time series.
Table 3. Radiocarbon data for Forestdale Valley 6 and 10 and Day Wash 14.

a All dates on charcoal measured at the University of Arizona Mass Spectrometry lab.
b Calibrated ages from Bayesian modeling are in italics. hpd, highest probability density.
RESULTS
Charcoal in modern analog samples showed a strong log-linear relationship with fines:sand ratios, suggesting that depositional energy had a strong impact on charcoal variability in modern samples (Fig. 3a), further supporting experimental observations of charcoal taphonomy by Nichols and Scott (Nichols et al., Reference Nichols, Cripps, Collinson and Scott2000; Scott et al., Reference Scott, Cripps, Collinson and Nichols2000). In aggregate, the ancient charcoal samples from FDV 6, FDV 10, and DaW 14 do not show such a strong relationship with grain size (Fig. 3b), indicating that there was variability in ancient charcoal accumulations related to fire activity and not just depositional energy. At DaW 16, soil P and unburned organic matter also demonstrate a strong correlation to either depositional energy or charcoal concentration (Fig. 3d). In thin section at DaW 16 and the Rocky Draw modern analog sites, unburned plant tissues are roughly as common as charcoal in modern postfire erosion deposits, and these unburned plant tissues may be the origin for their curious palynological properties. Pine pollen from four pollen samples at DaW 16 and one at the Rocky Draw range from 82% to 93.4%, higher in the earliest deposits and higher even than twentieth-century pine forest samples despite having a portion of the tree canopy killed by fire (see Table 4). This is probably due to enhanced postfire scouring of surfaces bringing unburned organic matter with adhering pine pollen as well as charcoal and phosphate-rich ash to the depositional sites.

Figure 3. (a) Scatter plot and regression line for modern analog charcoal samples by grain size; (b) scatter plot of ancient charcoal and grain size; (c) photograph of the profile exposed at modern analog site Day Wash 16 (DaW 16); and (d) stratigraphic plot of grain size, organic matter, charcoal, and phosphorus from DaW 16. Predicted charcoal abundance was calculated using a linear regression of charcoal concentration predicted by the log10 of silts and clays to sands. The relationship between charcoal and grain size for all modern analog samples was used to calculate the predicted high-severity fire curve. The relationship between charcoal and grain size for all paleoecological samples was used to calculate the predicted charcoal concentration for ancient fires.
Table 4. Paleoecological proxies across cultural periods.a

a Values for modern analog samples after high-severity fire are included for comparison.
b DaW, Day Wash; FDV, Forestdale Valley.
FDV 6 and FDV 10 were cut into alluvial terraces in the upper reaches of the valley bottom alluvium in the Forestdale Valley (Fig. 1). FDV 6 is a 3.83 m exposure near the northeastern limit of the large valley bottom meadow today. FDV 10 is a 3.4 m alluvial exposure surrounded by forest (hence consistently higher Pinus pollen values at FDV 10 than at FDV 6; see Figs. 4 and 5). Both Forestdale localities shared a similar sequence of 12 weakly separated alluvial soils within three sequential lithostratigraphic units (Fig. 2). The bottom unit (Unit I; soil units ACb7 to ACb11) is composed of well-bedded upward-fining sets of sandy to silty alluvium with high concentrations of charcoal in the finer-grained facies (Figs. 4–6). Fine-grain facies were enriched in SOM and displayed evidence for incipient soil formation (diffuse boundaries, lack of preserved bedding features) but low carbonate concentrations. Unit I soils at FDV 6 also displayed evidence for fluctuating water tables in the form of field-observed and micromorphological redoximorphic features (e.g., diffuse Fe-oxyhydroxide nodules and mottling; see Fig. 6). Unit II conformably overlies Unit I and consists of organic-rich, massive, fine-grained sandy silts and clays (soils ACb3 to ACb6). Unit II displayed weakly separated or welded fluvents with abundant SOM and pedogenic carbonate filaments overprinted from soil formation in the overlying Unit III soils. Unit III is composed of predominantly sandy sheetwash with very weakly separated alluvial soils within it (soils A to ACb2). The lithostratigraphic and soil stratigraphic similarity across these two localities and their overlapping radiocarbon dates indicate that each of these three stratigraphic units is roughly contemporaneous with the others. FDV 6 and 10 both probably began accumulating in the AD 1000s or AD 1100s (presumably after major downcutting immediately prior; Hereford, Reference Hereford2002; Karlstrom, Reference Karlstrom2005; Table 2). Sedimentation slowed with the overbank deposition of Unit II in the AD 1400s, which probably corresponded to shallow entrenchment downstream that eroded part of the Tla Kii ruin great kiva (Haury, Reference Haury1985c). Unit III saw a further change in sediment delivery from predominantly fine-grained overbank deposits to sandy sheetwash sometime in the AD 1500s. Entrenchment of the channel of Forestdale Creek to current levels did not happen until after AD 1910 (Haury, Reference Haury1985c), thereby abandoning FDV 6 and 10 as contexts for further deposition.

Figure 4. Stratigraphic plot for Forestdale Valley 6 (FDV 6) showing grain size, organic matter, carbonate, charcoal, phosphorus, Pinus pollen, Amaranthaceae + Asteraceae + Graminae pollen, Sporormiella, the location of radiocarbon and micromorphology samples, and rounded Bayesian-calibrated age ranges. Blue areas indicate rough assignments of soil strata to cultural periods based on an age–depth model of Bayesian-calibrated radiocarbon ages. Samples with pollen grain counts below 50 are displayed in figure as gray dots but not included in our pollen interpretations.

Figure 5. Stratigraphic plot for Forestdale Valley 10 (FDV 10) showing grain size, organic matter, carbonate, charcoal, phosphorus, Pinus pollen, Amaranthaceae + Asteraceae + Graminae pollen, Sporormiella, the location of radiocarbon and micromorphology samples, and rounded Bayesian-calibrated age ranges. Blue areas indicate rough assignments of soil strata to cultural periods based on an age–depth model of Bayesian-calibrated radiocarbon ages. Samples with pollen grain counts below 50 are displayed in figure as gray dots but not included in our pollen interpretations.
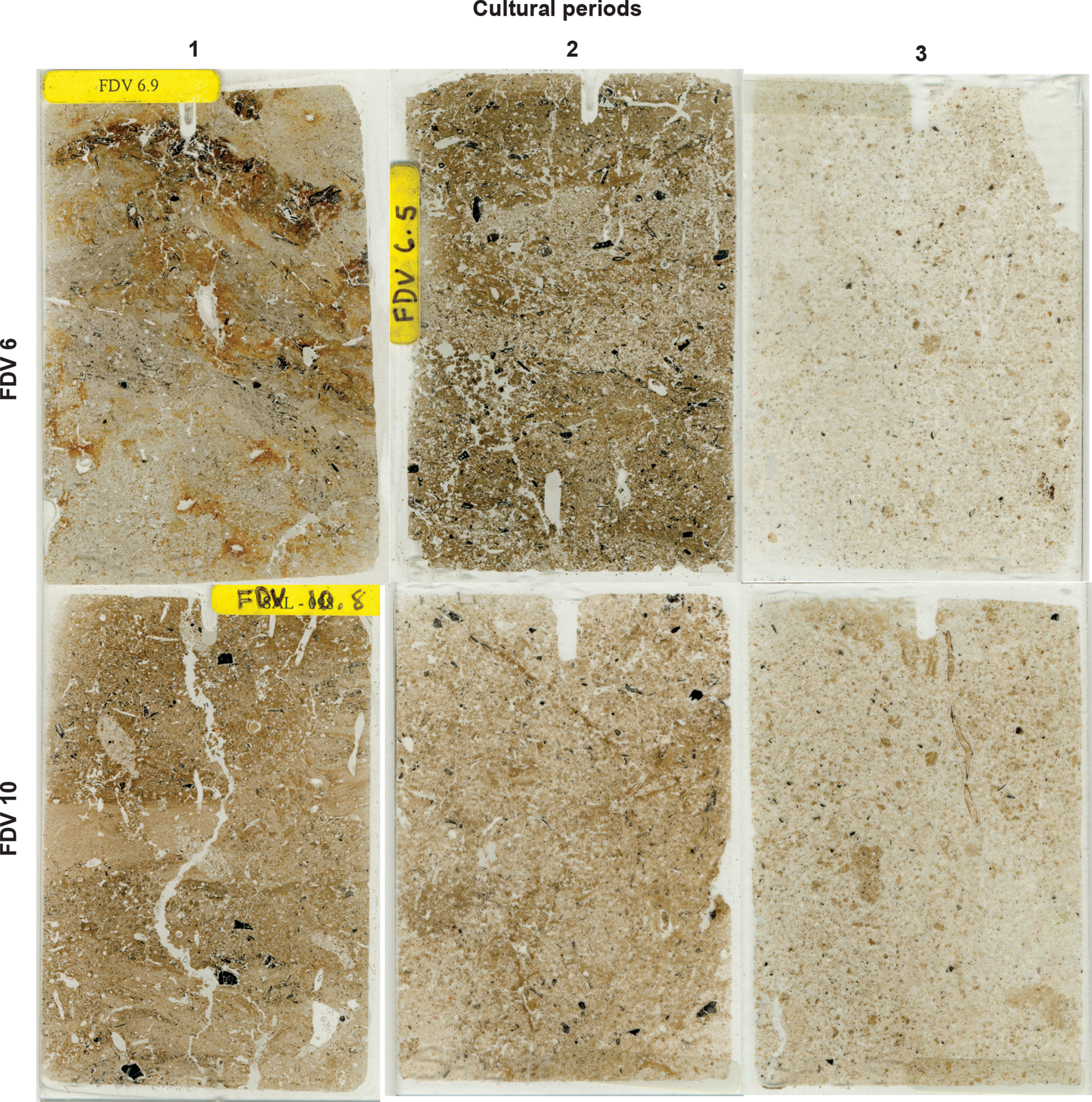
Figure 6. Macroscans of soil thin sections representative of each cultural period in the Forestdale Valley localities. Note the evidence of redox activity in FDV 6 in Period 1 soils and the decrease in both size and abundance of charcoal (black punctuations) in Period 3 soils at both localities. Notches mark the top of the sample. Each sample is ~50 mm wide.
Soils within lithostratigraphic Unit I roughly correspond to the period of most intensive land use by Ancestral Pueblo people (Period 1). Charcoal concentrations were at their highest levels during Period 1 at both FDV 6 and FDV 10 (Table 4), although they varied significantly due to varying depositional energy (Figs. 4 and 5). Pine pollen at both localities was generally within the range of historical ponderosa pine forests at the time. Grass and ruderal pollen increased over time within Unit I at FDV 6 (from 21% to 49.3%) and fluctuated at FDV 10 (from 6.9% to 29.2%). Zea pollen was recovered from the ACb11 soil at FDV 10. Phosphorus was generally low at both Forestdale Valley localities but was lower at FDV 6 than at FDV 10, which may be due to the fluctuating water table putting phosphate into solution and leaching it from the locality. Sporormiella spores were absent entirely from FDV 10, and only found in traces from two pollen samples from FDV 6 for this period.
Soils ACb3 through ACb6 at FDV 6 and ACb2 through ACb6 at FDV 10 are roughly assigned to land-use Period 2, with the lowest-intensity use of the areas. In these strata, charcoal declined and became less variable at both Forestdale Valley localities, although the fine-grained overbank deposits would have been suitable for higher charcoal concentrations, hence our higher predicted than actual charcoal concentrations. Pine pollen was essentially unchanged from the Unit I ranges until ACb4, when it declined at both localities for the rest of the record, corresponding to an increase in the abundance of grass and ruderal pollen. Phosphorus declined slightly in the unoccupied period at FDV 10, but without the impact of leaching at FDV 6 it increased slightly. Sporormiella spores were more common at FDV 6 and at very high levels (6.9%) from a pollen sample in ACb6 (immediately after Pueblo depopulation) that otherwise had very low pollen abundance. The only measurable Sporormiella spores in the entire record at FDV 10 were also from the ACb6 soil immediately after Pueblo depopulation.
Soils ACb1 and ACb2 belong to the period of intensive Apache land use (Period 3), wherein charcoal remained essentially the same or declined from the Period 2 at FDV 6 and FDV 10, even when accounting for grain-size differences that would predict lower charcoal concentrations. Phosphorus was variable but increased in both Forestdale localities. Pine pollen and grass and ruderal pollen continued the pattern established by the formation of ACb4 with lower pine pollen and elevated grass and ruderal pollen. While Sporormiella was absent from Apache-era deposits at FDV 10, concentrations at FDV 6 increased at that time.
DaW 14 was a 2.55-m-deep trench cut into an alluvial channel fan within a meander bend of Day Wash (Figs. 1 and 2). The exposed strata contain two lithostratigraphic units that include massive weakly sorted upward-fining sets with intercalated charcoal beds (Unit I; soil ACb10) underlying upward-coarsening sets of weakly separated sandy alluvial soils indicated by diffuse, irregular boundaries between units; micromorphological observations of homogenization and biological activity; and SOM measurements (Unit II; A to ACb9). Micromorphological observations indicate that Unit I accumulated very rapidly and included the preservation of unweathered ashes visible in thin section (Roos, Reference Roos2008; Fig. 7). Radiocarbon dates point to the deposition of Unit I in the mid- to late AD 1400s (Period 2). These dates were assumed to be contemporaneous in the Bayesian calibrations. Unit II accumulated more slowly than Unit I but still relatively rapidly, with both depositional and pedogenic features present in soil thin sections. Radiocarbon dates suggest that Unit II formed between AD 1500 and AD 1910, assuming modern downcutting occurred here contemporaneous with its occurrence in Forestdale.

Figure 7. Photomicrographs of unweathered ashes (gray rhombs in plane-polarized light; left] and with high order interference colors in cross-polarized light; right]) from Unit I at Day Wash 14 (DaW 14). These are indicative of very rapid deposition in the postfire environment to preserve these features.
Because deposition at DaW 14 began in the AD 1400s, there is no stratigraphic evidence for intensive Ancestral Pueblo land use, which would have ended there by AD 1325 (Mills et al., Reference Mills, Van Keuren, Stinson, Graves, Kaldahl, Newcomb, Mills, Herr and Van Keuren1999b). Unit I deposits formed during Period 2, when the area was less intensively used (Fig. 8). Unit I had the highest charcoal concentrations of any of the records, although this varied with depositional energy. Even accounting for grain-size variability, these charcoal concentrations were comparable to the modern high-severity fire deposits (see Table 4). Pinus pollen was also at the highest levels in any record in association with the first organic-rich charcoal bed within this unit, at levels comparable to modern high-severity fire deposits (>90%). By contrast, soil P concentrations were relatively low, although this could have been impacted by postdepositional leaching, as at FDV 6. A spike in Sporormiella coincides with the uppermost charcoal bed near the top of this deposit.

Figure 8. Stratigraphic plot for Day Wash 14 (DaW 14) showing grain size, organic matter, carbonate, charcoal, phosphorus, Pinus pollen, Amaranthaceae + Asteraceae + Graminae pollen, Sporormiella, the location of radiocarbon and micromorphology samples, and rounded Bayesian-calibrated age ranges. Blue areas indicate rough assignments of soil strata to cultural periods based on an age–depth model of Bayesian-calibrated radiocarbon ages. Samples with pollen grain counts below 50 are displayed in figure as gray dots but not included in our pollen interpretations.
Charcoal concentrations were lower in the Unit II–Period 2 strata, although they were higher than comparable strata at Forestdale and track grain-size predicted concentrations very closely. Soil P increased slightly, while pollen assemblages remained essentially unchanged from the top of the ACb10 soil at values consistent with a pine forest. Charcoal continued to decline in the Western Apache period, although this largely tracked changes in grain size. The most notable changes in the intensive Western Apache period (Period 3) were spikes in soil P and grass and ruderal pollen. Sporormiella spores, while low in concentration, show up in two pollen samples largely coincident with these spikes in soil P and ruderal pollen during the Western Apache period.
The surface soils at FDV 6, 10, and DaW 14 correspond to the period after the establishment of the Fort Apache Indian Reservation in 1870 (Period 4). These deposits have increased Pinus pollen, high levels of Sporormiella spores, and the lowest levels of charcoal concentrations, while FDV 6 and 10 have high levels of soil P.
DISCUSSION
Compared with the other periods, Period 1 at Forestdale had abundant charcoal, slightly higher phosphorus, and stable forest vegetation and domesticate pollen, which suggests that Pueblo and Apache residents of the area before AD 1400 used fire to manage their local landscape by adding extra burned area but not significantly impacting vegetation composition (Fig. 9; Table 5). This latter feature makes sense if the primary uses of fire were agricultural, perhaps something akin to the burn-plot farming hypothesized by Sullivan (Sullivan, Reference Sullivan1982; Roos et al., Reference Roos, Sullivan, McNamee and Dean2010). In this area of low-nutrient soils, low-intensity burning of surface biomass would have recycled nutrients critical for agriculture (DeBano et al., Reference DeBano, Neary and Ffolliott1998; Giardina et al., Reference Giardina, Sanford and Døckersmith2000). Moderate phosphorus concentrations in the alluvial sediments coincident with high levels of charcoal may indicate that much of the phosphorus generated by these low-intensity fires was taken up by cultigens and not remobilized to alluvial deposits (Giardina et al., Reference Giardina, Sanford and Døckersmith2000). At Forestdale, the biggest change when Ancestral Pueblo people migrated away from the area was a decline in charcoal and probably phosphorus (Tables 4 and 5). In other words, even in the absence of Pueblo burning, fires persisted, as corroborated by Mogollon Rim–wide and local Forestdale fire-scar chronologies (Weaver, Reference Weaver1951; Kaib et al., Reference Kaib, Swetnam and Morino2000). A spike in Sporormiella immediately after Pueblo depopulation in Period 2 indicates that there may have been a recovery in local herbivores once hunting pressure was removed (Schollmeyer and Driver, Reference Schollmeyer and Driver2013; Jones, Reference Jones2015).

Figure 9. Plots of charcoal and Sporormiella (left), and phosphorus and grass and ruderal pollen (right) for Day Wash 14 (DaW 14; top), Forestdale Valley 6 (FDV 6; middle), and Forestdale Valley 10 (FDV 10; bottom) between AD 1100 and AD 1900 using age–depth models of Bayesian-calibrated radiocarbon ages. Blue areas indicate cultural periods.
Table 5. General patterns of paleoecological proxies for each locality over time. All periods/proxies with high or anomalously high values are in bold.

At Day Wash, however, the earliest deposits look very much like more recent high-severity fire deposits both in morphology and in the paleofire proxies. Rapid sedimentation with anomalously high charcoal concentrations and extraordinarily high unburned organic matter and Pinus pollen are all consistent with high-severity fire deposits (compare Unit I in Fig. 8 with Fig. 3c). There is a spike in Sporormiella within these deposits as well that could either be a reflection of the herbivore rebound from fifteenth-century Pueblo depopulation or a local postfire benefit to deer (Odocoileus hemionus) or elk (Cervus canadensis) populations from the mid-1400s high-severity fire (Lewis et al., Reference Lewis, LeSueur, Oakleaf and Rubin2022). Like at Forestdale, charcoal was abundant in Unit II deposits in association with normal forest pollen assemblages, probably indicative of a lightning-driven surface fire regime after the mid-1400s high-severity fire, suggesting that the AD 1400s fire was not large enough to remove the entire canopy, much like the 1974 Day Burn, which only burned a portion of the watershed and was followed by ponderosa pine regeneration.
Western Apache economic and subsistence strategies focused on broad-spectrum hunting and gathering over large areas and included a wide variety of fire uses to promote hunting and gathering resources as well as to support gardening (Buskirk, Reference Buskirk1986; Roos, Reference Roos, Scheiber and Zedeño2015). We expected the most intensive Western Apache land use during Period 3, wherein charcoal declined but soil P and grass and ruderal pollen increased substantially at both Forestdale localities and at Day Wash. The elevated phosphorus and ruderal pollen would suggest increased fire activity that promoted these disturbance-loving taxa in the understory, but the charcoal is ambiguous (Fig. 9). Charcoal is sensitive to both changes in burned area but also to changes in fuel type (Marlon et al., Reference Marlon, Bartlein and Whitlock2006). Grass and herbaceous fine fuels combust more completely and produce less macroscopic charcoal (as measured here, >250 μm), so it is possible that increased burning in these fuel types may not have increased macroscopic charcoal production even as it did increase ash-related nutrients and favored disturbance-loving plants. Coincident with these increases in fire proxies are increases in Sporormiella at DaW 14 and FDV 6, which may indicate either large game benefiting from Apache cultural burning or, more likely, the keeping of domesticated livestock by Apaches (Basso, Reference Basso1998). Apaches used fire for many purposes, including to promote economically important plants represented by the ruderal pollen (Buskirk, Reference Buskirk1986; Roos, Reference Roos, Scheiber and Zedeño2015). The increases in Sporormiella may mean that some of this burning was to improve pasture for livestock. The establishment of these patterns for Period 3 clearly began when ACb4 was formed at both FDV 6 and FDV 10, earlier than what is marked by the conservative age–depth estimates for Period 3 on Figures 4, 5, and 9, consistent with Antonio de Espejo's observations of intensive Apache land use before AD 1583 (Forbes, Reference Forbes1960, p. 59).
The contrasts between the fire proxies during different cultural periods suggest that the eastern Mogollon Rim area experienced four different fire regimes over the last 1000 years. During Pueblo and Apache occupation (Period 1), lightning and horticultural burning may have provided the greatest amounts of area burned in surface fires over that time. When Pueblo people migrated away after AD 1400 and Apache use was light, fires persisted, but the substantial reduction of human ignitions reduced burned area or fire frequency. When Apache land use increased in intensity by the mid-sixteenth century, frequent surface fires in increasingly fine fuels promoted more grass, forb, and ruderal understory plants. Regional tree-ring records suggest that the Apache period had higher fire frequencies than much of the Southwest (Kaib et al., Reference Kaib, Swetnam and Morino2000). The fourth fire regime of the last millennium is the most contrastive with the other three. Beginning as early as the AD 1870s with settler colonialism and the suppression of traditional land use, fire has been largely excluded from Mogollon Rim forests until recent decades. The long fire-free intervals have increased stand density and made forests more vulnerable to high-severity, tree-killing fires. Two of the largest fires in Arizona's recorded history have been in the eastern Mogollon Rim region and the adjacent White Mountains. The 2002 Rodeo-Chediski and 2011 Wallow fires burned 380,000 ha of forest, much of this at high severity, including part of our study area. Before these megafires, the 1974 Day Burn burned at high severity, killing most trees in 1000 ha of our study area after at least six decades without fire. The basal deposits at Day Wash indicate that a high-severity fire event analogous to the 1974 Day Burn happened in the middle to late AD 1400s. Day Wash had been unoccupied by Ancestral Pueblo people for more than a century at that point, perhaps hinting that the absence of intensive Indigenous burning may have left some forests vulnerable to high-severity fire.
Paleoclimate patterns pertinent to ancient fire activity support these interpretations that the fire regimes described here are anthropogenic rather than driven by climate. In pine forests across the western United States, drought is a potent driver of burned area (Abatzoglou and Williams, Reference Abatzoglou and Williams2016); the 10-year average of the Palmer Drought Severity Index (PDSI; Cook et al., Reference Cook, Seager, Heim, Vose, Herweijer and Woodhouse2010; Gille et al., Reference Gille, Wahl, Vose and Cook2017) was dynamic across the Mogollon Rim region, but there are no clear long-term differences between the cultural periods (Fig. 10a). By contrast, long-term trends in temperature indicate clear differences between Period 1, which largely includes the warmer Medieval Climate Anomaly (MCA) and Periods 2 and 3, which include the Little Ice Age (LIA; Salzer et al., Reference Salzer, Bunn, Graham and Hughes2014; Fig. 10b). Paired two-sample t-tests identified no significant differences in PDSI across the periods, but Periods 2 and 3 were significantly cooler than Period 1 at P ≤ 0.05. Cooler temperatures during Period 3 may have enhanced fuel accumulation (Marlon et al., Reference Marlon, Bartlein and Whitlock2006), but this is actually in contrast to the evidence for lower charcoal deposition.

Figure 10. (a) Plots of Palmer Drought Severity Index (PDSI; Cook et al., Reference Cook, Seager, Heim, Vose, Herweijer and Woodhouse2010), (b) regional temperature (Salzer et al., Reference Salzer, Bunn, Graham and Hughes2014), and (c) intervals between regional fire years in a model of climate-driven surface fire activity (Roos and Swetnam, Reference Roos and Swetnam2012) with the cultural periods used in this study and the possible evidence for high-severity fire in Day Wash in the mid-1400s. Blue areas indicate cultural periods, and pink bars indicate probable high-severity fire in Day Wash.
Fire activity in the Southwest has been influenced by more than just fire-year drought. Significantly wet years before the fire year were important to build sufficient and continuous fuelbeds. Roos and Swetnam (Reference Roos and Swetnam2012) used dendroclimatic methods to calibrate a regression model of fire activity (percentage of regional tree-ring sites recording a fire) using up to three prior years and fire-year moisture. This reconstruction showed that conditions suitable for widely spreading fires were frequent during both the MCA and LIA but that the transition between the two may have seen unusually long fire-free intervals (Fig. 10c). These modeled climate drivers were equally common across the two most intensive land-use periods (Periods 1 and 3), indicating that the differences in fire proxies in the intensive-use periods cannot be explained by changes in climate drivers. However, longer fire return intervals may have been important in Period 2, when there is evidence for an increase in fire severity at DaW 14.
CONCLUSIONS
Ethnographic, paleoecological, and tree-ring evidence indicates that Ancestral Pueblo and Apache people managed fire on their more intensively used cultural landscapes, albeit in culturally variable ways (Buskirk, Reference Buskirk1986; Roos et al., Reference Roos, Swetnam, Ferguson, Liebmann, Loehman, Welch and Margolis2021). Anthropologists and ecologists increasingly recognize that Indigenous people serve keystone roles in ecosystems (Bliege Bird and Nimmo, Reference Bliege Bird, Bird, Fernandez, Taylor, Taylor and Nimmo2018; Ellis et al., Reference Ellis, Gauthier, Klein Goldewijk, Bliege Bird, Boivin, Díaz and Fuller2021). Indigenous burning, fuelwood harvesting, seed dispersal, and predation all have impacts on ecosystem composition, structure, and diversity across scales. In detailed ecological and ethnographic studies, Indigenous burning had important positive benefits on between-patch biodiversity and prey abundance (Bliege Bird et al., Reference Bliege Bird and Nimmo2018). The legacies of Indigenous land use may still be discernible in global biodiversity hot spots today (Ellis et al., Reference Ellis, Gauthier, Klein Goldewijk, Bliege Bird, Boivin, Díaz and Fuller2021), especially when research goals are foregrounded in reference to Indigenous presence and knowledge systems.
Small-patch burning by Indigenous people produces pyrodiversity that can have important ecological consequences. Differences in the spatial pattern of fire can allow fire-sensitive taxa to coexist with flammable ones (Trauernicht et al., Reference Trauernicht, Brook, Murphy, Williamson and Bowman2015). Even when fire frequencies are roughly similar between Indigenous and lightning-dominated fire regimes, cultural burning can modulate fire–climate relationships (Bliege Bird et al., Reference Bliege Bird, Codding, Kauhanen and Bird2012; Swetnam et al., Reference Swetnam, Farella, Roos, Liebmann, Falk and Allen2016; Taylor et al., Reference Taylor, Trouet, Skinner and Stephens2016; Carter et al., Reference Carter, Brunelle, Power, DeRose, Bekker, Hart and Brewer2021).
The seeming redundance of Indigenous burning with existing lightning fire regimes has sometimes been used to dismiss Native American burning as ecologically unimportant (Vale, Reference Vale2002). As demonstrated here, ancient landscapes that were subject to Native American fire management produced different fire regimes than lightning-only regimes but were also culturally variable. Different cultural applications of landscape burning had variable impacts on more than just burned area, including the types of fuels burned and the ecological consequences of that burning.
This study highlights important temporal and spatial diversity in the pyrogeography of the Southwest and elsewhere. Across the Mogollon Rim and elsewhere in the Southwest (Roos et al., Reference Roos, Swetnam, Ferguson, Liebmann, Loehman, Welch and Margolis2021), there were different fire regimes at different places and times across scales related to lightning density, topography, and prior disturbance as well as the intensity of human use, population density, and cultural practices of the local residents (Taylor et al., Reference Taylor, Trouet, Skinner and Stephens2016; Roos et al., Reference Roos, Swetnam, Ferguson, Liebmann, Loehman, Welch and Margolis2021). Particular local patterns should not be overgeneralized or projected across scales, because human behavior is spatially heterogeneous and scale dependent (Roos, Reference Roos2020). Rather, scientists, managers, and policy makers need to recognize and appreciate this cross-scalar form of geographic pyrodiversity in research design and for place-based management.
Data availability statement
All pollen and geoarchaeological data are available via Neotoma (https://www.neotomadb.org). Dataset ID nos.: 54690 (FDV 10), 54686 (FDV 6), and 27241 (DaW 14).
Acknowledgments
We acknowledge that the Mogollon Rim region is the traditional territory of the Ndée (Apache) people and the ancestors of Hopi, Zuni, and other Pueblo peoples. Although archaeology and historical documents provide evidence for particular types and intensities of land use and settlement, we respect that Elders describe Ndée presence in the area since time immemorial and that cultural connections to the land for Apache and Pueblo people extend deep into the past and are ongoing. This research was supported by International Arid Lands Consortium Grant 05R-09. We are grateful to the White Mountain Apache Tribal (WMAT) Council for permission to work on Apache land, and Apache-Sitgreaves National Forests, Jeremy Haines, John Welch, Greg Hodgins, Mark Altaha, Vance Holliday, Barbara Mills, Paul Goldberg, and Jeff Dean for guidance and support. Tom Swetnam and Chris Guiterman provided input on earlier versions of this article. Alejandro Figueroa aided with lab work. This project was approved by a WMAT Council resolution in May 2005, and this article underwent a Type I review by the WMAT pursuant to that resolution. The authors declare no competing interests.