Introduction
Thermal plasticity, such as acclimation, acclimatization or hardening responses (terminology reviewed in Hoffmann et al., Reference Hoffmann, Sørensen and Loeschcke2003; Chown and Terblanche, Reference Chown and Terblanche2006), is a phenotypic response by organisms to changes in environmental conditions, that may be adaptive (Huey et al., Reference Huey, Berrigan, Gilchrist and Herron1999; Kristensen et al., Reference Kristensen, Hoffmann, Overgaard, Sørensen, Hallas and Loeschcke2008). From an applied perspective, if thermal plasticity of traits, and acclimation responses more broadly, can be readily manipulated, there may be potential for developing useful applications, such as improving sterile insect technique programs that typically release insects into diverse or variable environmental conditions (Sørensen et al., Reference Sørensen, Toft and Kristensen2013; Terblanche, Reference Terblanche2014; Boersma et al., Reference Boersma, Boardman, Gilbert and Terblanche2019) or enhancing pollination rates for mass-reared, field released insect pollinators. However, there are scenarios where it is costly for organisms to acclimate (Gilchrist and Huey, Reference Gilchrist and Huey2001), observed in both laboratory (Basson et al., Reference Basson, Nyamukondiwa and Terblanche2011) and field assessments (Loeschcke and Hoffmann, Reference Loeschcke and Hoffmann2007; Kristensen et al., Reference Kristensen, Hoffmann, Overgaard, Sørensen, Hallas and Loeschcke2008; Schou et al., Reference Schou, Loeschcke and Kristensen2015). Direct fitness or performance costs may be incurred through maintaining and producing plastic responses, as well as indirectly through information acquisition, lost opportunities and genetic costs, i.e., where the genes promoting plasticity may either have a negative effect on other traits (antagonistic pleiotropy), or modify other genes (epistasis; DeWitt et al., Reference DeWitt, Sih and Wilson1998).
Diverse hypotheses exist for how acclimation may influence performance (reviewed in Huey and Berrigan, Reference Huey, Berrigan, Johnston and Bennett1996) and how these can be distinguished experimentally (Deere and Chown, Reference Deere and Chown2006; Clusella-Trullas et al., Reference Clusella-Trullas, Terblanche and Chown2010). These include the beneficial acclimation hypothesis which predicts that individuals given the opportunity to acclimate will perform better in that environment than individuals that were not given an opportunity to acclimate (Leroi et al., Reference Leroi, Bennett and Lenski1994; Huey and Berrigan, Reference Huey, Berrigan, Johnston and Bennett1996). ‘Colder-is-better’ or ‘hotter-is-better’ are environment-specific hypotheses predicting that acclimation to either colder or warmer conditions, respectively, will consistently improve performance relative to other environmental conditions, regardless of the conditions of the target environment (Zamudio et al., Reference Zamudio, Huey and Crill1995; Huey and Berrigan, Reference Huey, Berrigan, Johnston and Bennett1996). When and where costs associated with plastic responses result in a (mis)match with the environmental conditions is currently not well understood (Niehaus et al., Reference Niehaus, Angilletta, Sears, Franklin and Wilson2012; Kleynhans et al., Reference Kleynhans, Clusella-Trullas and Terblanche2014; Salachan and Sørensen, Reference Salachan and Sørensen2017; Shinner et al., Reference Shinner, Terblanche and Clusella-Trullas2020), but is key to understanding species' performance in diverse environmental conditions.
To control multiple concurrent stressors or diverse, complex environmental variables, tolerance or performance assays are generally conducted using well-established laboratory methods typically under rigorously controlled thermal (or other) conditions. Trait responses are later considered within the context of prevailing field conditions or used for forecasting responses to climate change (reviewed in e.g., Terblanche and Hoffmann, Reference Terblanche and Hoffmann2020). However, there are a handful of studies that have directly examined the correlation between laboratory and field responses by including an assessment of field performance or fitness. These studies often rely on outcomes of the recapture rate of acclimated individuals following mark and release studies into a resource-poor or novel natural habitat (Kristensen et al., Reference Kristensen, Hoffmann, Overgaard, Sørensen, Hallas and Loeschcke2008; Chidawanyika and Terblanche, Reference Chidawanyika and Terblanche2011), or predation/parasitism rate for biocontrol agents, e.g., Trichogramma carverae Oatman & Pinto (Thomson et al., Reference Thomson, Robinson and Hoffmann2001) and Adalia bipunctata L. (Sørensen et al., Reference Sørensen, Toft and Kristensen2013). When these studies have been undertaken they have generally shown a strong association between diverse field performance estimates, with individuals acclimated to the conditions most like those experienced during field assays typically performing better (Kristensen et al., Reference Kristensen, Hoffmann, Overgaard, Sørensen, Hallas and Loeschcke2008; Chidawanyika and Terblanche, Reference Chidawanyika and Terblanche2011; Sørensen et al., Reference Sørensen, Toft and Kristensen2013; Terblanche, Reference Terblanche2014). The costs of not being acclimated, or of being acclimated to the wrong conditions, may not always be observed when estimated under laboratory conditions (e.g. survival assays, Kristensen et al., Reference Kristensen, Hoffmann, Overgaard, Sørensen, Hallas and Loeschcke2008), or evident in field releases under benign conditions (Thomson et al., Reference Thomson, Robinson and Hoffmann2001). It is not always possible to determine if the mismatch or absence of observed acclimation costs in studies of laboratory and field performance is evidence of a lack of correlation between these estimates or the absence of sufficiently stressful conditions in either the laboratory (Kristensen et al., Reference Kristensen, Hoffmann, Overgaard, Sørensen, Hallas and Loeschcke2008) or the field environment (Thomson et al., Reference Thomson, Robinson and Hoffmann2001).
One potential method that has been widely employed to tease out such problems and disentangle the mismatch between laboratory and field estimates of acclimation costs is to conduct experiments in three operational environments simultaneously; field, semi-field, and the laboratory; representing a continuum of decreasing environmental complexity (Terblanche, Reference Terblanche2014; Hoffmann and Sgrò, Reference Hoffmann and Sgrò2017). Inclusion of the semi-field environment for releases conducted within an enclosed, large space but still exposed to several concurrent natural environmental conditions, removes the potential problem of low recapture rates that often occur in mark-release-recapture (MRR) studies due to predation and emigration (Manly, Reference Manly1985). It also provides a viable alternative for examining the relative performance (flight activity/dispersal) of invasive and/or damaging insect species under novel environmental conditions without actively releasing them in the field, potentially facilitating tests of plasticity theory or validating acclimation responses in a climate change context (Terblanche and Hoffmann, Reference Terblanche and Hoffmann2020). The value of a semi-field approach to tests of plasticity and acclimation responses is however not yet clear.
To examine the relative costs and benefits of acclimation on thermal tolerance and performance in an insect pest species and understand better the impact of thermal acclimation on field performance, we performed laboratory assays of common thermal tolerance estimates (heat knockdown time [HKDT], chill coma recovery time [CCRT] and critical thermal limits, [CTLs]), together with MRR under semi-field (greenhouse) and field conditions using the Mediterranean fruit fly, Ceratitis capitata (Wiedemann) (Diptera: Tephritidae). While other studies have used semi-field performance estimates to assay the potential costs of acclimation, this is the first study to take such a multifaceted approach. Previously, Esterhuizen et al. (Reference Esterhuizen, Clusella-Trullas, van Daalen, Schoombie, Boardman and Terblanche2014) tested if thermal acclimation experienced during the pupal stage (15, 20, 25 and 30°C) influenced the flight ability of adults at these same four test conditions. They found marked improvements in low-temperature flight ability with low-temperature acclimation, and overall, strong support for the ‘colder-is-better’ hypothesis (sensu Deere and Chown Reference Deere and Chown2006). Esterhuizen et al. (Reference Esterhuizen, Clusella-Trullas, van Daalen, Schoombie, Boardman and Terblanche2014) also examined sex, body mass, wing morphology, and physiological and biochemical rates as potential explanatory factors of varying flight performance, but these were generally unresponsive or lacked the ability to predict the large performance changes observed. Given this foregoing information from an across life-stage approach, we built upon this work and conducted acclimation regimes at three different thermal conditions (20, 25 and 30°C) during the adult life stage to utilize the multifaceted method for comparison. We also incorporated a variety of different environmental descriptors (mean, maximum, minimum temperature and season of release) into the MRR analyses to determine if a similar set of environmental variables explained the patterns of trap capture response seen in semi- and field assays that may best describe performance in this species. We predicted that thermal-tolerance assays will show similar patterns of benefits and costs of acclimation to field assays, while the semi-field MRR will provide a more simplified setting to quantify the costs of thermal history on performance, relative to the field release environment. We also therefore expected that the patterns of trait thermal acclimation response would be strongest in the lab and weakest in the field setting, but with a broadly similar overall direction of effects.
Materials and methods
Study species
Ceratitis capitata pupae were obtained from a large, outbred population, regularly supplemented with wild individuals, and maintained at Citrus Research International (CRI) in Nelspruit, South Africa (further details of insect culture conditions are provided in Nyamukondiwa and Terblanche, Reference Nyamukondiwa and Terblanche2009). Experimental adults were maintained in square mesh cages (40 × 40 × 40 cm) with access to food (sugar crystals) and water ad libitum. Insects were held in three climate chambers (LE-509, MRC, Holon, Israel) at one of three environmental conditions (20, 25 and 30°C). In all cases, the 25°C ‘pre-treatment’ group served as a handling control and is common good practice in thermal acclimation studies; this ensures that handling did not inadvertently induce some sort of stress response might be misinterpreted as being a thermal acclimation response. Adult acclimation consisted of rearing larvae under standard laboratory conditions (25°C) before placing 2 day-old adults in one of the three thermal environments for 5 days prior to testing. Seven day-old adult flies were then used for assessing thermal tolerance and performance. No individuals were used for more than one experiment.
Critical thermal limits
The methodology employed to assess CTLs, notably the starting temperature and rate of increase/decrease employed, can significantly influence trait estimates (Chown et al., Reference Chown, Jumbam, Sørensen and Terblanche2009; Terblanche et al., Reference Terblanche, Hoffmann, Mitchell, Rako, le Roux and Chown2011). As the influence of these conditions on estimates of thermal limits has already been well documented in this species (Nyamukondiwa and Terblanche, Reference Nyamukondiwa and Terblanche2010), a standard protocol for CTL estimates was chosen. Flies were placed singly into a double jacketed chamber, or ‘organ pipes’ connected to a programmable, circulating fluid bath (cw410-wl, Huber, Germany) containing 50% propylene glycol: water mixture. A thermocouple attached to a digital thermometer was placed into the central chamber to monitor the real-time change in temperature during each experiment. Both critical thermal maxima (CTmax) and critical thermal minima (CTmin) were started from a set point of 25°C before the temperature was increased/decreased at a rate of 0.25°C/min. Critical thermal limits were identified at the physiological end-point of the flies, determined as the temperature at which the individual flies lost coordinated muscle function and could no longer respond to gentle prodding using a fine thermally inert plastic rod. Twenty individuals of mixed sex from each temperature acclimation were assessed per trait. Sex was not identified for each individual as this has been shown to have little effect of CTLs in C. capitata (Nyamukondiwa and Terblanche, Reference Nyamukondiwa and Terblanche2009).
Heat knockdown and chill coma recovery time
Following protocols outlined in Weldon et al. (Reference Weldon, Terblanche and Chown2011), flies were placed singly into vented 7 ml plastic vials before being placed into each assay. For HKDT, flies were placed onto a heated thermal stage attached to a circulating fluid bath (as above). This stage was constructed of an enclosed Perspex® box fixed on top of an aluminium base and water was circulated within the base to achieve a target stage surface temperature of ~43°C. HKDT was recorded as the time following placement on the thermal stage that each fly became incapacitated, i.e. lost their righting ability.
CCRT was determined by submerging flies in vented 0.65 ml microcentrifuge plastic tubes sealed in a Ziploc bag into a programmable fluid bath containing 100% ethanol at 0°C for one hour. The flies were then returned to 25°C and the time taken for each to regain consciousness, coordination and mobility, i.e. to stand erect unassisted, was recorded. Twenty individuals of mixed sex from each temperature acclimation were assessed per trait.
Semi-field performance
Eight separate greenhouse releases were conducted to assess field performance under controlled conditions, but spanned a range of ambient temperatures from 18–32°C. One thousand adult flies that had acclimated to one of three temperatures (20, 25 or 30°C; approx. 330 flies each) were dusted with fluorescent powder (pink, blue and yellow) randomised prior to each release. The flies were released at one end of a 15 × 3 m enclosed greenhouse and allowed to move without human interference towards an attractant (BioLure®; ammonium acetate, trimethylamine hydrochloride and 1,4-diaminobutane; Chempac Pvt. Ltd., Paarl, South Africa) at the opposite end (following Steyn et al., Reference Steyn, Mitchell and Terblanche2016). Counts of individuals that reached the attractant (within 1.5 min) were used for subsequent analysis. A Thermochron iButton® (Dallas Semiconductors, Model DS1920; 0.5°C accuracy) data logger was suspended from the ceiling to record ambient temperature and relative humidity throughout the trial. Care was taken to avoid releasing on rainy and/or overcast days to minimize varying light intensity.
Field performance
Four field releases were conducted, two during summer (February) and two in autumn (May) at Stellenbosch within the Western Cape region of South Africa. Approximately 3000 flies per site were acclimated as adults (20, 25 or 30°C), dusted with fluorescent powder (pink, blue and yellow) randomised prior to each release. The flies were transported and released from a central location at the Stellenbosch University experimental farm at Welgevallen [33°56′ S, 18°52′ E]. Traps were hung in a rectangular pattern around a single central release point with three traps (15 m apart) in each external row and two traps in the middle row (30 m apart) (following Chidawanyika and Terblanche, Reference Chidawanyika and Terblanche2011). All traps were hung at ~1.5–2 m (following Martinez-Ferrer et al., Reference Martinez-Ferrer, Campos and Fibla2010), oriented along the rows and secured to reduce wind-related movement. Traps were checked daily for 5 days to record new captures. Two types of Ceratitis traps (bucket and delta) were baited with BioLure® and a small block of Dichlorvos DDVP was placed at the bottom of each trap to kill the attracted flies. Ambient microclimate temperature and humidity were recorded throughout the course of each release-recapture experiment using three shaded iButtons® with a 5-min sampling frequency for 5 days located on the ground, mid-tree height and the upper canopy at the central release point.
Statistical analyses
Thermal tolerance
The relative effect of acclimation temperature (20, 25, and 30°C; Tacc) on laboratory thermal tolerance estimates was conducted using generalized linear models (Gaussian distribution, identity link function) following log10 transformation to account for over-dispersion in R (ver. 3.1.0; R Development Core Team, 2014). In all cases, we use the 25°C group as a reference group against which the other treatments are compared.
Semi-field and field performance
The relative importance of the identified environmental descriptor variables, season, mean recorded temperature (Tmean) during recapture period (from iButton data), Tmin, Tmax and acclimation temperature (Tacc), on the relative recapture rate for the semi-field and field assays were assessed using the RandomForestRegressor package in SciKit Learn in Python (v.3.8.3), following similar implementation by Boersma et al. (Reference Boersma, Boardman, Gilbert and Terblanche2019).
To compare between different estimates and days (particularly for the semi- and field releases), trait results were calculated as a difference between treatment and the reference 25°C groups (i.e. normalized) which act as a control for handling as well as other unknown factors and is aimed to eliminate potential cohort effects among different release experiments. Performance was determined based on the flight activity/dispersal of the flies, measured as the number of individuals recaptured. This relative performance was calculated separately for each release day by dividing the values for the 20 and 30°C groups by the values obtained by those acclimated at 25°C for any given day. This allowed the variation (i.e. different cohorts or possible differences in rearing or handling) between the different release days to be controlled effectively while showing the direction of any potential plastic responses (Chidawanyika and Terblanche, Reference Chidawanyika and Terblanche2011; Boersma et al., Reference Boersma, Boardman, Gilbert and Terblanche2019). All results were presented as relative tolerance or a relative number of recaptures (unless otherwise stated) and 25°C was set as the control or reference group for comparisons in the subsequent analyses following e.g. Chidawanyika and Terblanche (Reference Chidawanyika and Terblanche2011). All analyses were performed using full factorial generalized linear models with Gaussian distribution and identity link function in SAS (Enterprise guide 5.1, SAS 9.1, SAS Institute, Inc., North Carolina). Fly recapture data were analysed as ‘recapture ratios’; the proportion of flies caught in an acclimation group relative to the control group. Due to the high number of zeros in the recapture data (most notably in the field releases) the data was transformed using log10 (n + 1). Using generalized linear models (Gaussian distribution, identity link function) in SAS and scaling data by deviance to account for over-dispersion, the effect of mean temperature (within the day/hour of recapture) and acclimation temperature on the relative field and semi-field recapture numbers were examined. Models were also fitted separately for each acclimation treatment to gain a better understanding of the relationship between environmental temperatures and recapture rate. TableCurve 2D (version 5.01.02) (SYSTAT Inc, San Jose, California, USA) was used to fit multiple linear and non-linear equations (following Steyn et al., Reference Steyn, Mitchell and Terblanche2016).
Results
Laboratory assays
The magnitude of the effect of acclimation temperature on CTLs was lower than for HKDT and CCRT, particularly for CTmax (fig. 1a). However, in general, the colder acclimation temperature resulted in lower cold tolerance (CTmin and CCRT) and vice versa for heat tolerance (CTmax and HKDT). The two CTLs (fig. 1a, b) shared the same significant factors (lower relative tolerance for 20°C than the 30°C group) in the analyses (table 1).
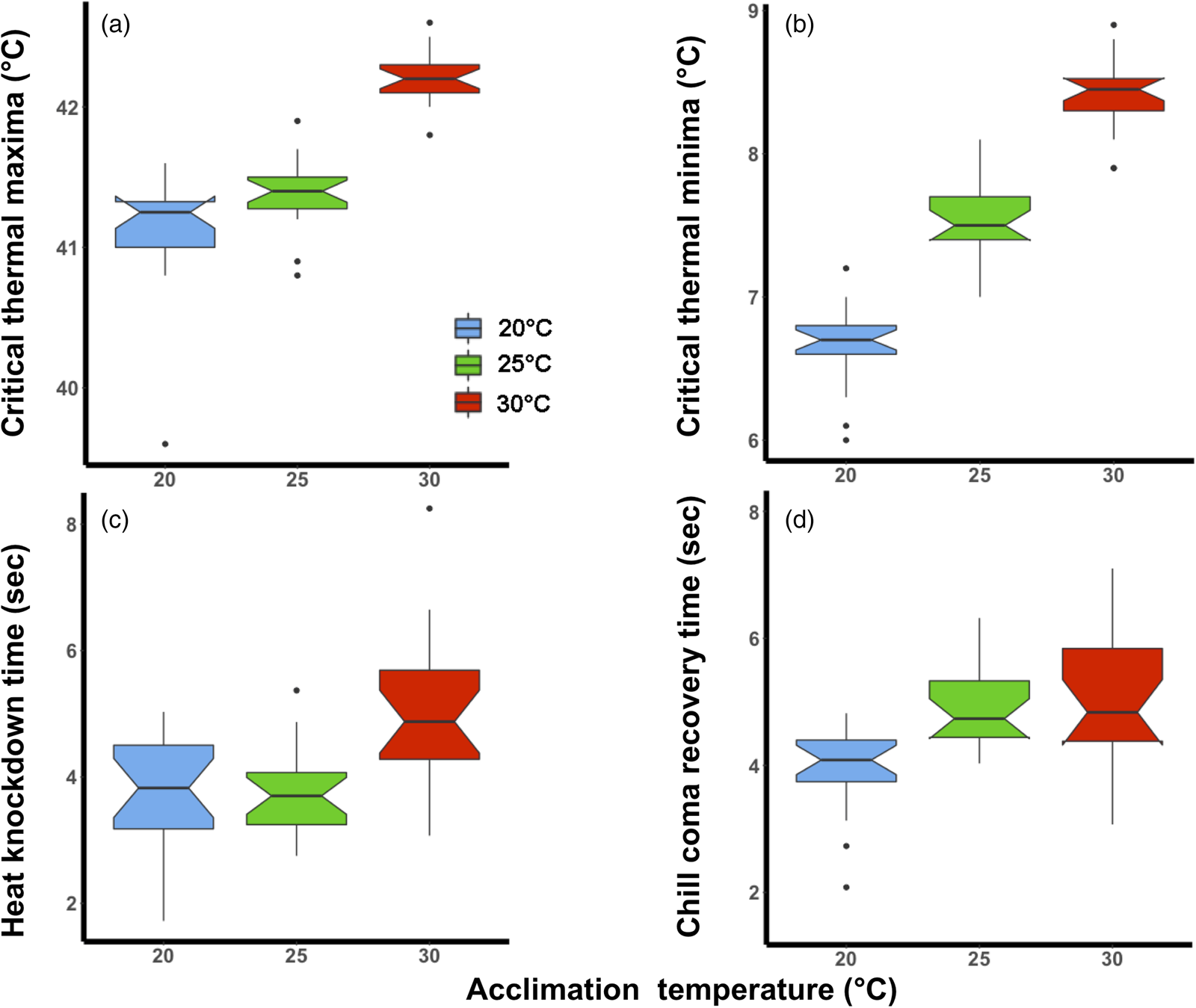
Figure 1. Laboratory thermal tolerance estimates of Ceratitis capitata following acclimation to three environmental temperatures (20, 25, or 30°C) at the adult life stage; (a) critical thermal maxima, (b) critical thermal minima, (c) heat knockdown time and (d) chill coma recovery time. The box plots are coloured according to acclimation treatment, blue (20°C), green (25°C) and red (30°C). The notch on the boxplot illustrates the 95% confidence interval.
Table 1. Summary of results of generalized linear models (Gaussian distribution, identity link function) investigating the effect of acclimation temperature (20, 25, and 30°C; Tacc) on laboratory-based thermal tolerance estimates, critical thermal maxima (CTmax), minima (CTmin), heat knockdown time (HKDT) and chill coma recovery time (CCRT) for Ceratitis capitata

SEM, standard error of the mean.
The 25°C (control) acclimation treatment was set as the base (reference) level for these analyses.
Factors in bold indicate significance at P = 0.05.
The 30°C acclimation significantly increased the flies' HKDT. However, the 20°C acclimation did not significantly differ from the control indicating the direction of the response was different for these treatments (table 1, fig. 1c). The 20°C acclimation significantly decreased the CCRT, while the 30°C acclimation did not differ from the control (table 1, fig. 1d).
Semi-field and field assays
The variable Tacc was the most important for explaining the relative recapture rate, ranking between 38–40% for both semi-field and field assays (fig. 2). However, after this point, there was a marked difference in the influence of the different predictors on the variance structure between the two environments. For the semi-field releases, Tmax was the next largest contributor, ranking at 37%, followed by Tmean at 17% (fig. 2a). However, for the field releases, the Tmin and Tmean environmental variables were next most important, ranking approx. 22% each followed by Tmax at 15% (fig. 2b).

Figure 2. Feature importance analysis using Random Forest regressions examining the effect of environmental descriptor variables on the relative recapture rate (recapture ratio) of Ceratitis capitata following mark-release-recapture in either semi-field (panel A) or field (panel B). Environmental descriptors are; Tmean, mean recorded temperature during recapture period; Tacc, adult acclimation treatment experienced prior to release (thermal history); Season, time of year (i.e. summer or winter) when the release was conducted.
Under semi-field conditions, there was no significant predictor of fly recapture numbers, even after the recapture number was standardized by the control (i.e., estimated as a recapture ratio) (table 2). Therefore, acclimation had no effect under semi-field conditions. There appeared to be a negative association between the recapture ratio of both the cold (20°C) and hot (30°C) acclimation treatments and the mean temperature during the recapture period in the semi-field (fig. 3b), but likely non-linear. After performing TableCurve 2D line-fitting analysis, the best-fit relationship between the recapture ratio and Tmean was identified as a decaying exponential curve (fig. 3b) for 20°C (R 2 = 0.650, P = 0.016) and 30°C (R 2 = 0.630, P = 0.018) acclimation treatments. Despite the generalized linear model analysis showing an absence of improvement in acclimation treatment relative to the control group (table 3), flies from both acclimation groups were recovered more frequently at temperatures below 25°C.

Figure 3. Relationship between mean ambient temperatures recorded during recapture period and the recapture ratio of Ceratitis capitata following mark-release-recapture after acclimation for semi- (a, b) and field (c, d) conditions, respectively. Data were transformed to log(n + 1) in order to account for zero recapture, and recapture ratios determined relative to the 25°C control group. Each acclimation group's data were fitted separately (i.e. 20 and 30°C), with solid lines indicating significant responses and dashed, non-significant. Fitted model equations for the recapture ratio treatment groups (b, d) with bold values are indicative of significant effects.
Table 2. Summary of results of generalized linear model (GLM) analyses (Gaussian distribution, identity link function) investigating the effects of acclimation temperature (20, 25, 30°C; Tacc) on the absolute numbers of recaptured flies and the proportion of recaptured flies relative to the intermediate control group (25°C; recapture ratio)

SEM, standard error of the mean.
In all cases investigated, the effect of acclimation temperature (Tacc) and field temperature (Tmean) were the continuous variables, and recapture ratios or absolute number captured as the dependent variable. The Wald's χ 2 was used to test for significant differences between acclimation groups.
Factors in bold indicate significance at P = 0.05.
Table 3. Summary results of generalized linear models (Gaussian distribution, identity link function) of the effect of mean temperature recorded during recapture period (Tmean) and acclimation temperature (20, 25, and 30°C; Tacc) on recapture ratio of Ceratitis capitata following release under semi-field and field conditions

AIC, Akaike information criterion; SEM, standard error of the mean.
The 25°C (control) temperature was set as the base level for the analysis. Data from the semi-field and field were analysed separately before being compared in the ‘comparison’ analysis.
Factors in bold indicate significance at P = 0.05.
Under field conditions, there was a significant effect of field temperature (estimated from iButton recordings) and its interaction with acclimation (table 2). When the relationship between mean temperature and recapture ratio was calculated for each acclimation treatment independently, the model fitted to the cold acclimation was significantly positive (slope = 0.06), whereas for the warm-acclimated flies it was not significant (table 3). This may indicate a potential benefit for cold acclimation as the temperature increases.
The overall analysis of both semi-field and field methods simultaneously found there was a significant difference between the two (table 3). Thus, there was no universal, consistent effect of acclimation on the recapture ratio when compared between the different environmental operational methods employed in this study.
Discussion
We found marked effects of adult thermal history on thermal performance and acclimation responses that might be largely expected given the results of previous studies (Loeschcke and Hoffmann, Reference Loeschcke and Hoffmann2007; Kristensen et al., Reference Kristensen, Hoffmann, Overgaard, Sørensen, Hallas and Loeschcke2008; Chidawanyika and Terblanche, Reference Chidawanyika and Terblanche2011). Under laboratory conditions, such thermal acclimation responses in traits of CTmax, CTmin, HKDT and CCRT were well established previously for C. capitata in e.g., Nyamukondiwa and Terblanche (Reference Nyamukondiwa and Terblanche2010) and Weldon et al. (Reference Weldon, Terblanche and Chown2011), respectively. We therefore do not discuss these kinds of responses extensively here, but they serve as (i) confirmation that we could replicate earlier findings and (ii) that there is a general positive effect on stress resistance traits under controlled conditions within the adult life-stage, albeit with variation among traits or metrics of heat and cold stress, a finding that is in keeping with the broader literature (reviewed in e.g. Terblanche et al., Reference Terblanche, Hoffmann, Mitchell, Rako, le Roux and Chown2011; Terblanche and Hoffmann, Reference Terblanche and Hoffmann2020). However, the direction and magnitude of acclimation responses, and their field relevance, is by no means a foregone conclusion, especially for dispersal responses or locomotor performance of insects (Chown and Terblanche, Reference Chown and Terblanche2006; Ghalambor et al., Reference Ghalambor, McKay, Carroll and Reznick2007; Clusella-Trullas et al., Reference Clusella-Trullas, Terblanche and Chown2010; Ferrer et al., Reference Ferrer, Mazzi and Dorn2014; Sgrò et al., Reference Sgrò, Terblanche and Hoffmann2016; Terblanche and Hoffmann, Reference Terblanche and Hoffmann2020). For the handful of studies that reported field effects of thermal acclimation on ectotherm performance, most showed general support of the ‘beneficial acclimation hypothesis’, and either costs and benefits depending on the specific conditions (Kristensen et al., Reference Kristensen, Hoffmann, Overgaard, Sørensen, Hallas and Loeschcke2008; Chidawanyika and Terblanche, Reference Chidawanyika and Terblanche2011), or no costs (Thomson et al., Reference Thomson, Robinson and Hoffmann2001). By contrast, in our study we found marked differences between the acclimation responses detected between the three major operational environments employed.
Many studies have implied that performance and tolerance estimates measured in the laboratory are adaptive and would provide an advantage under field conditions (Huey et al., Reference Huey, Berrigan, Gilchrist and Herron1999; Wilson and Franklin, Reference Wilson and Franklin2002), yet fewer studies have actively tested this (but see Loeschcke and Hoffmann, Reference Loeschcke and Hoffmann2007; Kristensen et al., Reference Kristensen, Hoffmann, Overgaard, Sørensen, Hallas and Loeschcke2008; Chidawanyika and Terblanche, Reference Chidawanyika and Terblanche2011). Indeed, few studies have examined the costs and benefits of plastic physiological responses in the field (reviewed in Sgrò et al., Reference Sgrò, Terblanche and Hoffmann2016) and much scope for field validation of acclimation responses remains (Terblanche and Hoffmann, Reference Terblanche and Hoffmann2020). Those that have done so typically report contrasting evidence, from strong costs and benefits (Kristensen et al., Reference Kristensen, Hoffmann, Overgaard, Sørensen, Hallas and Loeschcke2008; Chidawanyika and Terblanche, Reference Chidawanyika and Terblanche2011), to some context-specific benefits and weak or no costs (Thomson et al., Reference Thomson, Robinson and Hoffmann2001). While gaining an understanding of the relative costs and benefits of thermal acclimation using natural systems under a diverse set of environmental conditions would be optimal, this is not always experimentally feasible. However, the effect that using laboratory estimates has on overall outcomes and conclusions surrounding field benefits of trait plasticity remains to be better explored and reported. Broadly, there is rather poor understanding of laboratory acclimation responses and how transferable they are to the field and thus any evolutionary fitness implications, or for manipulating performance in the context of applied pest management objectives, such as SIT (Boersma et al., Reference Boersma, Boardman, Gilbert and Terblanche2019).
In agreement with results reported in the same species but using an across-life-stage approach (Esterhuizen et al., Reference Esterhuizen, Clusella-Trullas, van Daalen, Schoombie, Boardman and Terblanche2014), that focused on laboratory flight performance estimates under controlled thermal conditions, we detected significant effects of thermal acclimation and ambient temperature on MRR estimates under field conditions in the adult stage. In this earlier study, Esterhuizen et al. (Reference Esterhuizen, Clusella-Trullas, van Daalen, Schoombie, Boardman and Terblanche2014) found that flies were able to fly more readily at all temperatures, and especially in cold condition trials at 15°C, when they were cold-acclimated but not when they were warm-acclimated. Moreover, cold acclimated flies performed equally well at warm conditions compared to warm-acclimated flies (Esterhuizen et al., Reference Esterhuizen, Clusella-Trullas, van Daalen, Schoombie, Boardman and Terblanche2014). We find support for the ‘beneficial acclimation’ hypothesis in the laboratory for traits of thermal limits. In the semi-field environment, both cold and warm acclimated flies performed better than the control, but only at ambient temperatures below 25°C. The results in laboratory and semi-field conditions were not extended into the field (table 4). However, it did agree with the laboratory flight assay results of Esterhuizen et al. (Reference Esterhuizen, Clusella-Trullas, van Daalen, Schoombie, Boardman and Terblanche2014) where they documented strong support for the ‘colder-is-better’ hypothesis. Moreover, these performance changes in Esterhuizen et al. (Reference Esterhuizen, Clusella-Trullas, van Daalen, Schoombie, Boardman and Terblanche2014) consistently found improvements in flight performance for reasons that are mechanistically unclear, but were not related to changing body size or wing loading, and was a pattern observed similarly in both sexes of C. capitata, regardless of the test temperature conditions.
Table 4. Summary of results from our assays according to the treatment group's relative performance

Arrow direction indicates relative performance, up means higher than the control and down means lower than a control group. The equal sign indicates performance equivalent to that of the control group.
It is perhaps broadly expected that as environmental conditions become more complex, and more factors interact with the organism's biology, then so too will the environment influence recapture rates. The assumption that semi-field conditions provide a valuable null model, since there are fewer uncontrollable variables than in the field setting, against which field data can be compared, forming a continuum of environmental complexity from the laboratory to the field setting (see discussion in Terblanche, Reference Terblanche2014), may not be accurate, or at least not in the case of C. capitata here. We found here that the semi-field results provided support for a stimulatory influence of cold or warm acclimation on low temperature performance, rather than being strictly confirmatory of either the laboratory or the field results. This was illustrated by considering the contribution of the environmental descriptors assessed during the field MMR assays in the feature importance analysis (fig. 2). In contrast to the field tests, the semi-field assays showed a marked influence of Tmax and very little effects of all other environmental variables measured, reflected in the absence of discernible costs or reductions in performance of acclimation below 25°C (fig. 2). Despite both temperature treatments performing similarly (and better than the control group) in the semi-field releases, these effects were substantially different in the field estimates. In the field, the recapture rates of the warm acclimated group did not differ from that of the control group and was recaptured less frequently than the cold acclimated group, irrespective of ambient temperature. The benefit that cold acclimation provided shifted from favourable at lower temperatures in the semi-field, to providing a weak benefit as temperatures increased in the field. This highlights that acclimation costs and benefits may only be realized under specific operational conditions and that the fundamental impact of temperature on performance should always be assessed under diverse environmental temperatures, even when other environmental variables are controlled or perhaps invariant.
Increased recapture under semi-field conditions was found for both warm and cold-acclimated flies compared to the 25°C control flies at cooler ambient conditions (<25°C). We suggest that this incongruency was influenced by the disruption of a number of natural dispersal cues and processes under semi-field conditions; effects that typically remained minor in both the laboratory and the field assays. Insects rely on a variety of dispersal and navigational cues, such as pheromones and polarized light (Cardé and Willis, Reference Cardé and Willis2008; Reppert et al., Reference Reppert, Gegear and Merlin2010; Warren et al., Reference Warren, Weir and Dickinson2018), body condition (Skórka et al., Reference Skórka, Nowicki, Kudłek, Pępkowska, Śliwińska, Witek, Settele and Woyciechowski2013), behavioural drive (Steyn et al., Reference Steyn, Mitchell and Terblanche2016), morphology (wing loading, Esterhuizen et al., Reference Esterhuizen, Clusella-Trullas, van Daalen, Schoombie, Boardman and Terblanche2014) and physiological performance (Clusella-Trullas et al., Reference Clusella-Trullas, Terblanche and Chown2010; Steyn et al., Reference Steyn, Mitchell and Terblanche2016). Many of these factors have been shown to jointly determine realized dispersal in the field (reviewed in Clobert, Reference Clobert2012). Moreover, intrinsic traits of the organism can interact strongly with environmental factors, such as temperature, to influence dispersal ability (Harrison et al., Reference Harrison, Woods and Roberts2012; Dillon and Dudley, Reference Dillon and Dudley2014). The stimulating cues from the attractant employed in our study may not have been as attractive to our control group flies (reared at 25°C) as they are more likely to have already mated compared to those from the less optimal conditions at 20 or 30°C (e.g. Anton et al., Reference Anton, Dufour and Gadenne2007). In addition, the attractant lure's influence may interact with navigational cues obtained from polarized light that are perhaps also disrupted in the greenhouse (Horváth et al., Reference Horváth, Kriska, Malik and Robertson2009). Additional effort is needed to undertake field and semi-field MRR assays in other species to further understand the source of these striking differences in acclimation responses between traits and under diverse conditions. Future work should focus on including diverse metrics in the semi-field and field environment, for instance, time to first capture, total number captured, size of flies captured and rate of capture per hour are just some examples of results that can be readily obtained with the field methods used here. Furthermore, if flies are able to be kept alive after capture, various biochemistry assays, ‘-omics’, or metabolic activities can also be scored thereby providing another dimension to this type of work.
The reduction in recapture ratio of acclimated flies at temperatures above 25°C that we observed in the semi-field experiments was not observed for the 20°C acclimation treatment in the field assays. Improved overall performance for the 20°C acclimated group relative to the control group and the 30°C treatment group was especially evident under warmer conditions. This pattern was perhaps unexpected given the semi-field results, but indicated that cross-tolerance effects may have occurred under warmer temperatures. The prior exposure to the cold for short periods in the adult life stage may have induced the expression of heat shock proteins (HSPs), as found in cross-tolerance studies in another fly species, Belgica antarctica (Benoit et al., Reference Benoit, Lopez-Martinez, Elnitsky, Lee and Denlinger2009), or perhaps through other physiological mechanisms such as metabolic rate differences. If HSPs, which are known to improve heat tolerance in almost all organisms examined (reviewed in Feder and Hofmann, Reference Feder and Hofmann1999) and in C. capitata too (Mitchell et al., Reference Mitchell, Boardman, Clusella-Trullas and Terblanche2017), were involved in this instance, then the effect should have been more obvious in the warm-acclimated group. The absence of any benefit of heat acclimation prior to heat exposure was somewhat unexpected and understanding the mechanistic (cellular) basis of cross-tolerance in this species could be a useful line of future investigation as shown for Ceratitis rosa (Gotcha et al., Reference Gotcha, Terblanche and Nyamukondiwa2018).
In conclusion, this study showed two striking results that were of broader interest to field tests of the impact of thermal acclimation. First, different operational environments produced distinctly different outcomes, suggesting a highly context-specific nature to how thermal acclimation influenced traits of thermal performance or tolerance. In consequence, care is required when testing adaptive hypotheses of phenotypic plasticity. Second, despite the apparent continuum of environmental complexity among operational environments (laboratory < semi-field < field), thermal performance estimates may not follow the same trend. This result suggested that the laboratory and semi-field environment may not provide reliable, transferable outcomes for tests of phenotypic plasticity theory or acclimation responses. Obtaining accurate estimates of the direction and magnitude of phenotypic plasticity, and its associated costs and benefits, is central to improving predictions of species' responses to global climate change (Chevin et al., Reference Chevin, Lande and Mace2010; Sgrò et al., Reference Sgrò, Terblanche and Hoffmann2016) and for improving biological control techniques (such as SIT) where success or efficacy is dependent on high quality, mobile insects (Boersma et al., Reference Boersma, Boardman, Gilbert and Terblanche2019). Thermal tolerance and performance traits estimated in the laboratory and semi-field may not provide an accurate estimate of thermal acclimation responses in the field, and caution is needed when transferring outcomes across traits, diverse environmental conditions and study systems.
Acknowledgements
We are grateful to Welgevallen Experimental Farm and Citrus Research International for logistical support. VMS was supported by the National Research Foundation (NRF) and the Centre for Invasion Biology. KAM was supported by the Claude Leon Foundation. CN was supported by Hortgro Science. JST and this work were supported by the NRF Incentive Funding for Rated (IFR) researcher's scheme, Hortgro Science and the International Atomic Energy Agency Coordinated Research Programme on dormancy responses. We are grateful for comments on the work by Dan Hahn, Chris Weldon and the anonymous referees.
Conflict of interest
None declared.