Introduction
Mars, our closest planetary analogue, once had a more substantial hydrological cycle (Wordsworth, Reference Wordsworth2016), possibly with oceans (Carr and Head, Reference Carr and Head2003; Redd Reference Redd2020; Scheller et al., Reference Scheller, Ehlmann, Hu, Adams and Yung2021) and lakes shaping its surface and hosting microbial life (Cabrol and Grin, Reference Cabrol and Grin1999). Over the Red Planet's history, this water cycle (Jakosky, Reference Jakosky2021), dynamo (Mittelholz et al., Reference Mittelholz, Johnson, Feinberg, Langlais and Phillips2020), and igneous activity either diminished or virtually disappeared. Present-day Mars has active seasonal changes in ice caps (Becerra et al., Reference Becerra, Smith, Coronato and Rabassa2020), permafrost (Wray, Reference Wray2020) and atmospheric composition (Trainer et al., Reference Trainer, Wong, McConnochie, Franz, Atreya, Conrad, Lefèvre, Mahaffy, Malespin and Manning2019). Undesired terrestrial microbes (Spry et al., Reference Spry, Rummel, Race, Conley, Siegel and Kminek2017), or extant martian life might co-exist on Mars today (Cabrol, Reference Cabrol2021).
We are now using orbiters, landers and rovers to explore these tantalizing possibilities (Smith et al., Reference Smith, Kelley and Basner2020a). The next frontier in space exploration is to return samples from and eventually land humans on Mars. The US National Aeronautics and Space Administration (NASA)'s Perseverance rover is paving the way for the first Mars-sample return (Farley et al., Reference Farley, Williford, Stack, Bhartia, Chen, de la Torre, Hand, Goreva, Herd and Hueso2020); a future mission will also bring back those samples. Added to the existing fleet of rovers and orbiters is the Indian Space Research Organization (ISRO)'s Mangalyaan orbiter (Lele, Reference Lele2014), the United Arab Emirates Mars Mission Hope (Sharaf et al., Reference Sharaf, Amiri, AlDhafri, Withnell and Brain2020) and the China National Space Administration's (CNSA)'s Tianwen-1 orbiter with the Zhurong rover (Wan et al., Reference Wan, Wang, Li and Wei2020). They are resolving the geology of the planet, mostly characterizing the surface and near-surface (to centimetre depths). The European Space Agency (ESA)'s and Roscosmos State Corporation (RSC)'s ExoMars Rosalind Franklin rover will explore the martian subsurface with a specialized payload designed for life detection down to depths of metres. The Japanese Aerospace Exploration Agency (JAXA) will return samples from the martian moon Phobos for launch in 2024 with the Martian Moons eXploration (MMX) sample-return mission (Usui et al., Reference Usui, Bajo, Fujiya, Furukawa, Koike, Miura, Sugahara, Tachibana, Takano and Kuramoto2020). SpaceX has an ambitious programme for human settlement on Mars and the Moon (Reference Bramson, Byrne, Beyer, Cummings, Golombek, Head, Hodges, Levy, Lim and MarinovaBramson et al.) with the development of the Starship vehicle, bringing together a new era of humans in space.
Identifying habitable environments for both microbes (Cockell, Reference Cockell2021) and humans on Mars is a continuous process (Morgan et al., Reference Morgan, Putzig, Perry, Sizemore, Bramson, Petersen, Bain, Baker, Mastrogiuseppe and Hoover2021). An important aspect of the current missions is the increasing internationalization of space research (Baitukayeva and Baitukayeva, Reference Baitukayeva and Baitukayeva2020), providing an opportunity to unite nations in enabling humanity becoming an interplanetary species. Here, we pose questions on Mars relating to its natural history, the development of space technologies, the design of human missions and habitations, planetary protection policy, and impacts of Mars exploration on human society on Earth.
Early Mars
Loss of atmosphere
One of the most-striking differences between ancient and current Mars is that it once had a thicker atmosphere compared to the present day; the Noachian Mars atmosphere was more Earth-like in density. This raises the question of when most of the atmosphere was lost. There are compelling observation-based and theoretical calculations indicating that most of the martian atmosphere escaped into space early in the planet's history (Lammer, Reference Lammer2012; Jakosky et al., Reference Jakosky, Slipski, Benna, Mahaffy, Elrod, Yelle, Stone and Alsaeed2017), when the intensity of extreme ultraviolet (EUV) and the solar wind flux from the young Sun were much higher than today (Ribas et al., Reference Ribas, Guinan, Güdel and Audard2005). Moreover, the martian dynamo disappeared ~4.1 Ga ago (Lillis et al., Reference Lillis, Robbins, Manga, Halekas and Frey2013; Mittelholz et al., Reference Mittelholz, Johnson, Feinberg, Langlais and Phillips2020), leaving present-day Mars with only weak crustal magnetic fields (Johnson et al., Reference Johnson, Mittelholz, Langlais, Russell, Ansan, Banfield, Chi, Fillingim, Forget and Haviland2020). Our understanding of present-day escape of martian atmosphere, and thus our overview of atmosphere losses throughout Mars' history, has improved greatly with observations from NASA's Mars Atmosphere and Volatile EvolutioN (MAVEN) orbiter in conjunction with detailed theoretical models (Dong et al., Reference Dong, Bougher, Ma, Lee, Toth, Nagy, Fang, Luhmann, Liemohn and Halekas2018; Jakosky et al., Reference Jakosky, Brain, Chaffin, Curry, Deighan, Grebowsky, Halekas, Leblanc, Lillis and Luhmann2018).
Atmospheric ion escape rates on Mars significantly varied over time, ranging from ~1027 s−1 at ~4 Ga to ~1024 s−1 at the present epoch (Fig. 1). Figure 1 also shows that the photochemical escape rate of hot atomic oxygen in the martian exosphere lies between ~1026 s−1 at ~4 Ga and ~1025 s−1 today. These simulations are also consistent with the idea that Mars transitioned from an early warmer and wetter world to a desiccated, planet with a frigid surface and tenuous atmosphere. It is noteworthy that space weather events at early epochs of solar evolution, such as Interplanetary Coronal Mass Ejections (ICMEs), could have catastrophically impacted Mars' atmospheric retention. Recently (8 March 2015), an ICME sideswiped Mars and led to a tenfold enhancement in the atmospheric ion escape rate (Dong et al., Reference Dong, Ma, Bougher, Toth, Nagy, Halekas, Dong, Curry, Luhmann and Brain2015), confirming that ICMEs – a highly frequent phenomenon of young stars and hence of the young Sun – could have had a direct effect on the removal of Mars' atmosphere.
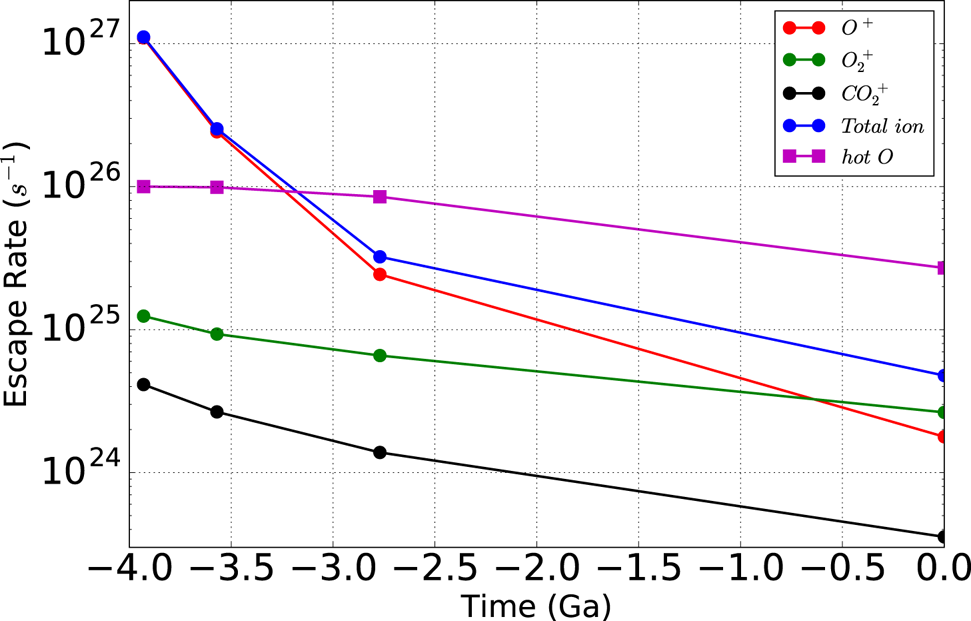
Fig. 1. Calculated atmospheric ion and photochemical (hot exospheric oxygen) escape rates over the martian history (under normal solar wind conditions) (Dong et al., Reference Dong, Lee, Ma, Lingam, Bougher, Luhmann, Curry, Toth, Nagy, Tenishev, Fang, Mitchell, Brain and Jakosky2018).
The habitability of Mars was drastically affected by the loss of the planet's atmosphere. An atmosphere is necessary to maintain surface liquid water, and to protect putative biota from high-energy particles and radiation. The temporal changes of Mars' atmosphere may shed light on exoplanet habitability. Recent numerical and theoretical studies indicate that both magnetized and unmagnetized planets around M dwarfs (stars much smaller and dimmer than our Sun) might be particularly susceptible to the depletion of ~1 bar atmosphere over sub-Gyr timescales due to the high stellar radiation and particle fluxes within habitable zones (Dong et al., Reference Dong, Jin and Lingam2020). Such exoplanets orbiting M dwarfs, as well as those around young solar-type stars (Dong et al., Reference Dong, Huang and Lingam2019), could have been subjected to high atmospheric escape rates early in their history. It is important to take this time-dependence into account when modelling the habitability of Mars or exoplanets.
Evidence for and against tectonics on Mars
Early global tectonic-magmatic processes are part of Earth's geological record and have shaped Earth's crust (Stern, Reference Stern2018). On Mars, radial grabens (Fig. 2) and concentric wrinkle ridges connected to the Tharsis rise (Andrews-Hanna, Reference Andrews-Hanna2020), and Valles Marineris display properties of fault systems (Mège and Masson, Reference Mège and Masson1996; Baker et al., Reference Baker, Maruyama and Dohm2007). A number of smaller tectonic-like features also occur in these regions of Mars. Tectonic processes could account for the alternating magnetized stripes in the southern highlands, possibly due to periodic changes in the magnetic polarity of the martian core, and the gradual spreading at mid-oceanic ridges during crustal formation.
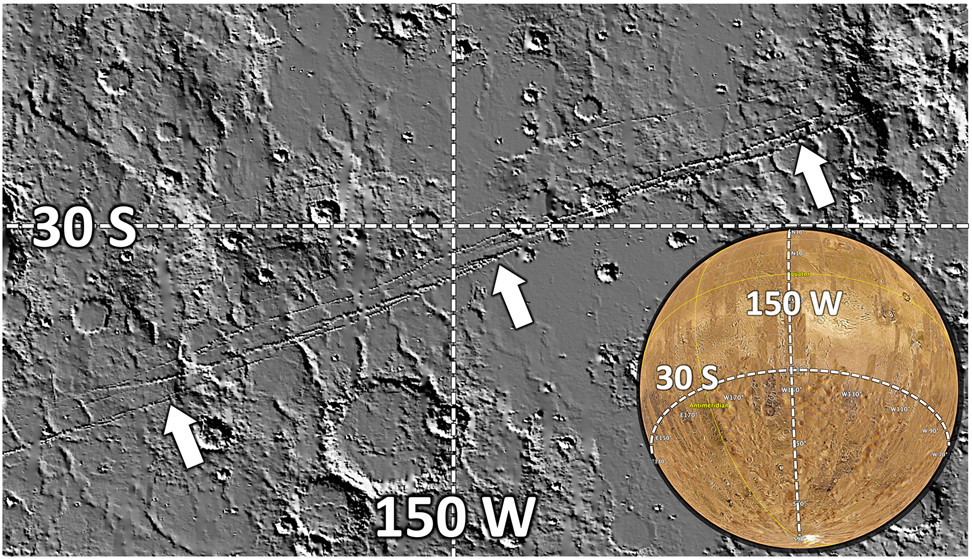
Fig. 2. Radial grabens and fractures in the Sirenum Fossae region of southern Tharsis.
The short length of strike slip faults on Mars may not be related to plate tectonics (Schultz, Reference Schultz1989). Evidence of crustal consumption in the form of subduction zones is also lacking. However, certain faults – abrupt contacts of different rock units and related topographic features – suggest plate boundary-like structural lines (Kidman et al., Reference Kidman, MacLean and Maxwell2014). Such features would have formed early on in Mars' history. Mars is a planet of modest size planet with limited heat within, leading to relatively rapid rates of cooling and a thick crust. Therefore, a considerable amount of energy must have been required to cause large-scale fracturing of the martian crust. In addition, temperatures in the mantle may not have been high enough to maintain global plate recycling. This could also relate to the depletion of Mars' magnetic dynamo, convention currents, and possible magmatic plumes in its early mantle.
NASA's InSight Mars lander mission measured faint seismic activity to shed some light onto the question of Mars' internal structure (Knapmeyer-Endrun and Kawamura, Reference Knapmeyer-Endrun and Kawamura2020). Recent results suggest a larger, less dense and still-molten core (Stähler et al., Reference Stähler, Khan, Ceylan, Duran, Garcia, Giardini, Huang, Kim, Lognonné and Maguire2021). Combined with the relatively thick crust, we should not expect active tectonism. Any large temperature gradients, therefore, must have been created by impacts.
Impact events occurred frequently in Mars' early history. After the cessation of early global impact bombardment, compressive stresses may have occurred for extended periods of time (Watters, Reference Watters1993), producing wrinkle ridges in several volcanic regions (Schultz, Reference Schultz2000). Moderately small, up to 100 m-long extension-and-compressional features (Plescia and Golombek, Reference Plescia and Golombek1986) may have shaped these volcanic plains. However, displacements up to 100 km in size have also been observed (Yin, Reference Yin2012).
Weak plate recirculation does not remobilize as much material from the Mars interior to the surface as occurs on Earth. This produces a low redox gradient; it is the greater redox gradient on Earth that has supported life. However, UV-driven chemistry on the surface of present-day Mars has led to strong redox gradients occurring at depths of centimetres to decametres below the surface. Subsurface measurements, such as those from the ExoMars Rosalind Franklin rover (see Robotic exploration section), are expected to confirm this.
What explanation(s) are there for the hemispheric dichotomy?
The lower density of impact craters in Mars' northern hemisphere is coupled with a difference in topographic height between the two hemispheres. The northern hemisphere is lower-lying, and the most-ancient craters there have been buried beneath regolith (and/or rock) material whereas the ancient craters of the southern hemisphere can still be seen (Bouley et al., Reference Bouley, Keane, Baratoux, Langlais, Matsuyama, Costard, Hewins, Payré, Sautter and Séjourné2020).
The northern lowlands cover about a third of the planet, and in some places, the southern highlands reach more than 30 degrees north of the equator. The boundary between the northern lowlands and the southern highlands is marked by steep erosional slopes, with apparent traces of ancient shorelines (Sholes et al., Reference Sholes, Dickeson, Montgomery and Catling2020), suggesting that the northern lowlands were occupied by an ocean about 3.8–4.1 billion years ago. However, if an ocean existed with a water-depth of several kilometres, the northern lowlands would not have been shielded from impact craters. Why the two hemispheres exhibit dichotomy in their topographic heights is not known (Fig. 3). It has been inferred from studies of the planet's gravitational field that the crust in the north is only about 32 km thick whereas the southern crust is about 58 km thick. The difference in crust thickness should be explained to elucidate the origin of this dichotomy.

Fig. 3. Colour-coded digital elevation map of a latitudinal belt of Mars centred at 24 degrees north. The east-west extent is about 8000 km. Blue-coded elevations are low, whereas green, yellow and red are progressively higher. The total range in this image is about 9 km. The blue, low lying area is north of the dichotomy boundary. It has relatively few superimposed craters, because the surface has relatively young lavas and sediments. The green-yellow-red terrain is south of the dichotomy boundary. This is more ancient and has more craters, despite many of them having been partially erased by erosion. (NASA/JPLK/GSFC/Arizona State University).
It would be tempting to compare Mars' northern lowlands to Earth's thin ocean crust. However, Earth's oceanic crust is produced by seafloor spreading which requires tectonic plates to be moving apart. This type of tectonic process is balanced by convergence elsewhere on the globe (accommodated by subduction, where the edge of one plate descends below the edge of its neighbour). Mars shows no evidence of multiple tectonic plates. In fact, it has been described as a ‘one-plate planet’. This difference should be explained to elucidate the origin of this dichotomy.
There are three distinct theories:
– the low northern hemisphere was excavated by an extremely large impact soon after Mars' formation about 4.5 billion years ago (Andrews-Hanna et al., Reference Andrews-Hanna, Zuber and Banerdt2008; Leone et al., Reference Leone, Tackley, Gerya, May and Zhu2014);
– a persistent upwelling in the southern hemisphere mantle (Harder and Christensen, Reference Harder and Christensen1996) caused partial melting that produced magma which intruded into and erupted onto the planet's earliest crust, resulting in locally enhanced thickness; and
– the southern mantle upwelling was a convection pattern created as a consequence of the northern basin-forming impact (Andrews-Hanna et al., Reference Andrews-Hanna, Zuber and Banerdt2008).
The impact event (first theory) would have created a sink for sediments and a site for lava eruptions, both capable of burying the earliest craters that formed on the floor of the giant basin. The second theory looks to processes in Mars' mantle for an explanation. Maybe in a Mars-sized planet (smaller than Earth), convection patterns are simpler, and the sharpness of the dichotomy boundary resulted from erosion rather than reflecting the edge of an impact basin. The third theory integrates the first two theories. Which theory is correct remains unresolved.
The martian satellites
Phobos and Deimos are the two small natural satellites of Mars with some characteristics similar to asteroids, suggesting that they are objects that were captured in the planet's early history. However, these satellites move in circular orbits that are closely aligned with Mars' equator, which is difficult to explain for captured objects. These orbits are different from that of Earth's Moon, for example, which moves within a more or less ecliptic plane. The orbits of Phobos and Deimos suggest that these satellites formed from large impact ejecta from Mars that then accumulated/coalesced (Bagheri et al., Reference Bagheri, Khan, Efroimsky, Kruglyakov and Giardini2021). Alternatively, these satellites may have formed at the same time as proto-Mars itself. The morphology of Phobos is consistent with the idea that the satellite consists of loosely consolidated materials (a so-called rubble pile). Phobos' shape is near ellipsoidal – this could possibly be a relic of the effects of gravitational, rotational and tidal forces during its formation. Such conditions are met at a distance of ~3.3 Mars radii (our Moon is ~60 Earth radii away), which is where Phobos probably accreted.
Phobos is orbiting at about 9000 km from the centre of Mars, and therefore is affected by strong tidal forces, resulting in orbital decay. Phobos will likely be disrupted into pieces within a rather short timescale, on the order of a few tens of millions of years. Deimos orbits Mars ~23 500 km from the centre (~2.5 times greater than the orbit of Phobos) and is expected to escape from the martian system at some time in the distant future.
The Satellite-Ring Cycle model (Hesselbrock and Minton, Reference Hesselbrock and Minton2017) suggests that the moons of Mars were repeatedly created, destroyed, and then recreated. Following a typical tidal disruption of Phobos, a debris ring will form and spread. Most of this material will eventually impact the surface of Mars, but a fraction will migrate into higher orbits. Once beyond the so-called ‘Fluid Roche limit’, located at about 3.1 Mars radii from the planet's surface, the remaining material will re-accrete to form an offspring moonlet, which will again enter orbital decay. Phobos may be a product of the fourth or fifth generation of the Satellite-Ring Cycle.
Martian water, alteration minerals and methane
Lakes and seas on Mars
Life on Earth potentially originated in the ocean (Martin et al., Reference Martin, Baross, Kelley and Russell2008; Osinski et al., Reference Osinski, Cockell, Pontefract and Sapers2020), and thrives not only in the modern sea but also in >100 million lakes. It may be in for this reason that there is a widespread interest in ancient lakes and seas on Mars, including the ancient lake of the Jezero Crater that is currently being explored by the Perseverance rover. Early reconnaissance of Mars revealed intriguing evidence for many crater lakes (i.e. channels apparently terminating in basins) (Cabrol and Grin, Reference Cabrol and Grin1999; Horgan et al., Reference Horgan, Anderson, Dromart, Amador and Rice2020), and hinted that the northern hemisphere might have once contained a large ocean (Carr, Reference Carr1987). Modern assessments, based mostly on laser altimetry, high-resolution visible imaging, hyperspectral compositional remote sensing, and more sophisticated climate models strongly support the idea that many lakes have existed on Mars. However, they have created more questions than answers regarding a northern ocean.
It is widely accepted that several 100s of lakes existed on Mars, mostly during the Noachian (Cabrol and Grin, Reference Cabrol and Grin2010; Boatwright and Head, Reference Boatwright and Head2021). Evidence of open-basin lakes is relatively easy to identify because of outflowing channels which also indicate the maximum height of a base level at some period in time (Fassett and Head, Reference Fassett and Head2008b). Underfilled, closed-basin lakes are more difficult to characterize because the lake level, volume and lifespan are unclear. Aside from geomorphological evidence for lakes, compositional remote sensing reveals aqueous alteration minerals within specific lacustrine settings (Ehlmann et al., Reference Ehlmann, Mustard, Fassett, Schon, Head, Des Marais, Grant and Murchie2008a; Michalski et al., Reference Michalski, Glotch, Rogers, Niles, Cuadros, Ashley and Johnson2019).
On Earth, lakes are geologically transient and on Mars this was also true. Based on estimates of erosion, sediment mobilization, and delta construction it appears that any individual lake might have only existed for 104–106 years on Mars (Fassett and Head, Reference Fassett and Head2008a; de Villiers et al., Reference de Villiers, Kleinhans and Postma2013). Aqueous mineral assemblages could have formed in similar timescales (Bishop et al., Reference Bishop, Fairén, Michalski, Gago-Duport, Baker, Velbel, Gross and Rampe2018). Placing this in the context of the Noachian period, which lasted ~500 Myr, it is not clear that the existence of lakes implies warm climatic conditions or a substantially thicker atmosphere. It remains a question for debate whether most lakes on Mars formed in relatively short timescales in punctuated climate excursions on an otherwise relatively cold, frozen planet (Wordsworth et al., Reference Wordsworth, Ehlmann, Forget, Haberle, Head and Kerber2018).
One unusual lake that occurred in Mars' Eridania basin (Fig. 4) in the early Noachian could perhaps more accurately be referred to as a martian sea. This water body would have been kilometres deep, and contained as much water as most of the other lakes on Mars at that time combined. Extremely ancient, it contained thick, deep-water clays of likely hydrothermal origins and coastal evaporites, akin to deposits formed in Earth's oceans today (Michalski et al., Reference Michalski, Dobrea, Niles and Cuadros2017).

Fig. 4. A HiRISE false colour (IRB) image of putative hydrothermal seafloor clays in the deepest parts of Eridania basin. Yellow-brown-green clays are cut by a dense network of veins, and partially covered by younger, dark eolian material.
The case for a vast northern ocean is no stronger now than decades ago, but this hypothesis seems interesting and confusing in equal measure (Saberi, Reference Saberi2020). The vast channels that fed the putative ocean did not all occur at the same time (Warner et al., Reference Warner, Gupta, Muller, Kim and Lin2009). It is also not certain that these channels are formed exclusively by the action of water because low-viscosity lava is another possible erosive agent (Leverington, Reference Leverington2011). High-resolution remote-sensing data have been used to search for shorelines as evidence of a northern ocean, but this search has thus-far proved inconclusive. Currently, there is no definitive evidence to indicate that an ancient northern ocean once existed. One problematic issue is that, based on estimates, the amount of water present was insufficient to form a northern ocean, even taking into account the high rates of water loss to space (Carr and Head, Reference Carr and Head2015).
Carbonates on Mars
Mars' surface morphology was likely shaped by a warm and wet past (Carr, Reference Carr1996), though how warm, how wet, and how intermittent these conditions were remains uncertain. Water on the Red Planet would remain in the liquid phase at temperatures maintained by greenhouse gases, mitigating the low intensity of solar radiation on Mars' surface (20–30% lower than that on Earth's surface). It is uncertain whether Mars recycles crustal material (e.g. by plate tectonics) so surface materials ought to remain intact over time. Therefore, the relatively high CO2 pressure (PCO2) in the atmosphere of ancient Mars should be evidenced by isotopic and mineralogical substances in the crust, such as carbonates. Geochemical modelling of evaporating mineral sequences in aqueous environments on early Mars, such as a closed basin lake, would likely indicate the precipitation of various carbonates. These might include siderite (FeCO3), calcite (CaCO3), hydromagnesite (Mg₅(CO₃)₄(OH)₂⋅4H₂O), and magnesite (MgCO3) (Catling, Reference Catling1999). Carbonates on present-day Mars could in this way provide insights into early Mars.
Carbonate-rich outcrops formed through aqueous chemistry have only been detected at a few sites on Mars; by orbital remote sensing and landed missions. These sites include the Nili Fossae grabens that are associated with phyllosilicate, olivine-rich deposits (Ehlmann et al., Reference Ehlmann, Mustard, Murchie, Poulet, Bishop, Brown, Calvin, Clark, Des Marais and Milliken2008b) and the Comanche outcrop that is located within the Gusev crater (16–34 wt.%) (Morris et al., Reference Morris, Klingelhöfer, Schröder, Rodionov, Yen, Ming, de Souza, Wdowiak, Fleischer, Gellert, Bernhardt, Bonnes, Cohen, Evlanov, Foh, Gütlich, Kankeleit, McCoy, Mittlefehldt, Renz, Schmidt, Zubkov, Squyres and Arvidson2010). The Jezero Crater also contains carbonate coexisting with olivine and/or Fe/Mg-smectite-bearing outcrops (Goudge et al., Reference Goudge, Milliken, Head, Mustard and Fassett2017; Horgan et al., Reference Horgan, Anderson, Dromart, Amador and Rice2020).
The scarcity of carbonate outcrops on Mars is currently an enigma. However, calculations may have shed some light on this issue. For example, calculations of aqueous equilibria (Fairén et al., Reference Fairén, Fernández-Remolar, Dohm, Baker and Amils2004) are inconsistent with carbonate formation in an oceanic environment with pH values <6.2, PCO2 from 0.4 to 8 bar, and sulphate- and Fe concentrations of 13.5 and 0.8 mM, respectively. Nevertheless, the possibility that many carbonate-rich outcrops occur that are undetected from orbit cannot be excluded. Some sulphates, not identified by orbital remote sensing, were subsequently discovered by landed missions. The Perseverance, Tianwen-1 and ExoMars Rosalind Franklin rovers may yet reveal martian carbonate outcrops not identified by orbiters.
Carbonate formation by atmospheric processes
Some carbonates of potential atmospheric origin were found by missions to Mars or by martian meteorite studies. For example, calcium carbonate in the soil around the Phoenix landing site (3–5 wt.%) (Boynton et al., Reference Boynton, Ming, Kounaves, Young, Arvidson, Hecht, Hoffman, Niles, Hamara and Quinn2009) could form by the interaction of atmospheric CO2 with liquid water films on the surfaces of dust particles. Thermal Emission Spectrometer (TES) data analysis of dust from 21 regions of the martian surface measured 2–5 wt.% carbonates dominated by magnesite (Bandfield et al., Reference Bandfield, Glotch and Christensen2003), which is also consistent with MiniTES data at the landing site of the Opportunity rover where ~5 wt.% carbonate was identified in the regolith (Christensen et al., Reference Christensen, Wyatt, Glotch, Rogers, Anwar, Arvidson, Bandfield, Blaney, Budney and Calvin2004).
At Gale Crater, Curiosity rover's Sample Analysis at Mars (SAM) tool heated sediment and analysed emissions using gas chromatography mass spectrometry (GCMS). CO2 gas was detected at 450–800 °C, a finding consistent with <1 wt.% Fe-, Mg-rich carbonates (Sutter et al., Reference Sutter, McAdam and Mahaffy2019).
Based on the oxygen-isotope study of carbonates in meteorite ALH84001, Farquhar et al. (Reference Farquhar, Thiemens and Jackson1998) suggested two oxygen-isotope reservoirs; the atmosphere and the silicate planet. At the time of carbonate growth in this meteorite, the cause of an apparent atmospheric oxygen isotope disequilibrium (δ 17O) might be the exchange between the atmospheric CO2 and O (1D) produced by the photodecomposition of ozone. In other words, some carbonate may be generated in the atmosphere by photochemistry.
If carbonate can form from atmospheric CO2 via UVC-induced photochemistry (Farquhar et al., Reference Farquhar, Thiemens and Jackson1998), the energetic electrons resulting from electrostatic discharge (ESD) during martian dust storms (common during the Amazonian) may cause a similar reaction, but through electrochemistry. In a laboratory simulation, Na2CO3, NaClO3 and NaClO4 were generated after only 1 h in an ESD-Normal Glow Discharge (NGD) process on NaCl under 3 mbar CO2 (Wu et al., Reference Wu, Wang, Farrell, Yan, Wang, Houghton and Jackson2018). Furthermore, Na-, K-, Al- and Ca carbonates were identified in electrochemical reactions with chlorides under Mars atmospheric conditions (Wang et al., Reference Wang, Yan, Dyar, Houghton, Farrell, Jolliff, McLennan, Shi and Qu2020a, Reference Wang, Yan, Jolliff, McLennan, Wang, Shi and Farrell2020b).
Fe-oxides
Hematite – ferric oxide (Fe2O3) – is widely distributed across the surface of Mars. Hematite spherules, known as blueberries, have been found in the Meridiani Planum by the Opportunity rover. On Earth, hematite can form in a variety of environments and from a variety of parent materials. It is widely accepted that the occurrence of hematite ‘blueberries’ on Mars indicates that liquid water was once present in Meridiani (Squyres et al., Reference Squyres, Grotzinger, Arvidson, Bell, Calvin, Christensen, Clark, Crisp, Farrand, Herkenhoff, Johnson, Klingelhöfer, Knoll, McLennan, McSween, Morris, Rice, Rieder and Soderblom2004; Golden et al., Reference Golden, Ming, Morris and Graff2008). However, a clear understanding of the formation of these spherules is still a matter of debate. Various explanations have been investigated, such as the transformation of goethite (FeOOH) and other iron hydroxides (Glotch and Kraft, Reference Glotch and Kraft2008; Fu et al., Reference Fu, Jia, Wang, Cao, Ling, Liu, Shi, Wu, Li and Zhang2020), that initially precipitated from aqueous solution and subsequently transformed into hematite. Other theories suggest the role of hydrothermal fluids and seabed water flows depositing the blueberries as observed on Earth (Di Bella et al., Reference Di Bella, Sabatino, Quartieri, Ferretti, Cavalazzi, Barbieri, Foucher, Messori and Italiano2019), or that the formation of hematite spherules is associated with unique surface conditions on Mars, such as ablation of meteorites (Misra et al., Reference Misra, Acosta-Maeda, Scott and Sharma2014) or freezing aqueous suspensions of hematite nanoparticles (Sexton et al., Reference Sexton, Elwood Madden, Swindle, Hamilton, Bickmore and Elwood Madden2017).
Hematite deposits at Meridiani Planum were first discovered by the TES on the NASA orbiter Mars Global Surveyor (Christensen et al., Reference Christensen, Bandfield, Clark, Edgett, Hamilton, Hoefen, Kieffer, Kuzmin, Lane, Malin, Morris, Pearl, Pearson, Roush, Ruff and Smith2000). Strong absorptions at 315, 461 and 560 cm−1 observed by TES are characteristic of coarse, grey hematite (Christensen et al., Reference Christensen, Morris, Lane, Bandfield and Malin2001; Glotch et al., Reference Glotch, Morris, Christensen and Sharp2004). This finding prompted the selection of Meridiani Planum as the landing site for NASA's Mars Exploration Rovers mission (MER) that includes the Opportunity and Spirit rovers (Golombek et al., Reference Golombek, Grant, Parker, Kass, Crisp, Squyres, Haldemann, Adler, Lee, Bridges, Arvidson, Carr, Kirk, Knocke, Roncoli, Weitz, Schofield, Zurek, Christensen, Fergason, Anderson and Rice2003). The images returned by Opportunity revealed the abundant accumulations of spherical balls (<0.5 cm diameter). These spherules are ubiquitous on the surface of the martian landscape, shown as blue in the false-coloured images of the rover's panoramic camera (Fig. 5), and dubbed as ‘blueberries’ (their distribution was considered similar to that of the blueberries in a blueberry muffin) (Squyres et al., Reference Squyres, Grotzinger, Arvidson, Bell, Calvin, Christensen, Clark, Crisp, Farrand, Herkenhoff, Johnson, Klingelhöfer, Knoll, McLennan, McSween, Morris, Rice, Rieder and Soderblom2004). Multiple instruments on Opportunity (MiniTES, Pancam, Mossbauer spectrometer and APXS) confirmed that the mineralogy is hematite (Morris et al., Reference Morris, Klingelhöfer, Schröder, Rodionov, Yen, Ming, de Souza, Wdowiak, Fleischer, Gellert, Bernhardt, Bonnes, Cohen, Evlanov, Foh, Gütlich, Kankeleit, McCoy, Mittlefehldt, Renz, Schmidt, Zubkov, Squyres and Arvidson2006). Fragments of these spherules lack an internal structure (Klingelhöfer et al., Reference Klingelhöfer, Morris, Bernhardt, Schröder, Rodionov, De Souza, Yen, Gellert, Evlanov, Zubkov, Foh, Bonnes, Kankeleit, Gütlich, Ming, Renz, Wdowiak, Squyres and Arvidson2004; Squyres et al., Reference Squyres, Grotzinger, Arvidson, Bell, Calvin, Christensen, Clark, Crisp, Farrand, Herkenhoff, Johnson, Klingelhöfer, Knoll, McLennan, McSween, Morris, Rice, Rieder and Soderblom2004).
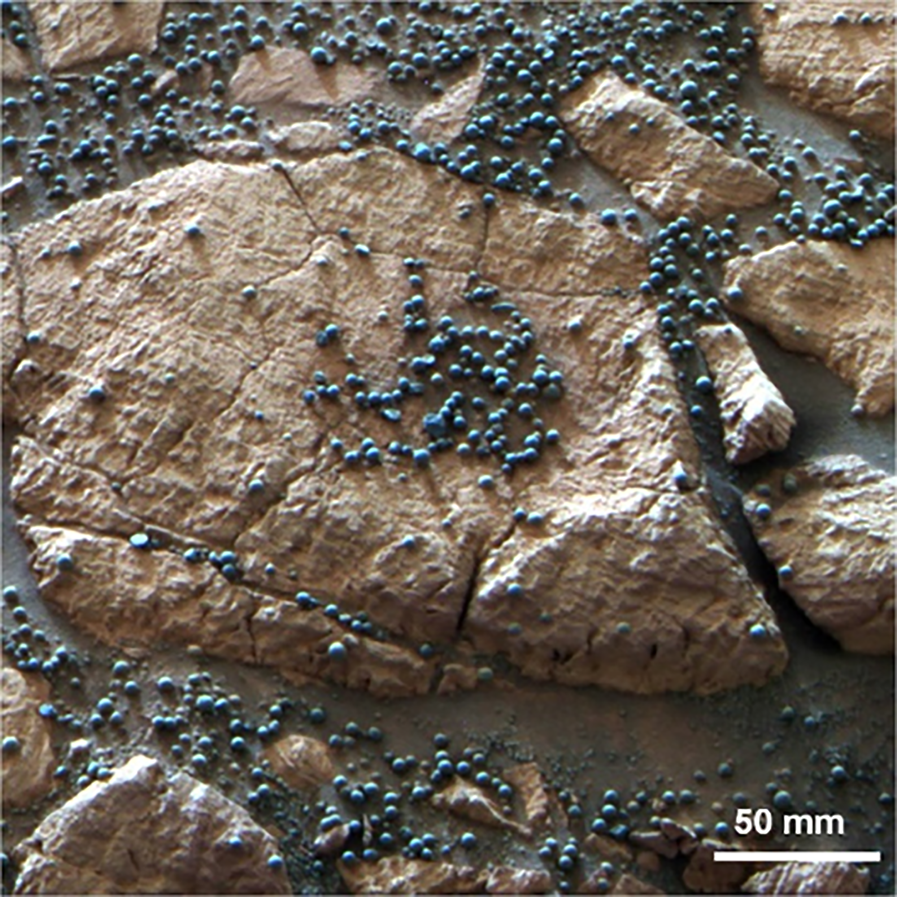
Fig. 5. Martian hematite spherules ‘blueberries’ discovered by the Opportunity rover. Image credit: NASA/JPL/USG.
The occurrences of these blueberries indicate hydrothermal activity in Meridiani Planum. By contrast, Spirit does not find any hematite in Gusev Crater. The Vera Rubin Ridge, also known as the Hematite Ridge, is an erosion-resistant feature within the Gale Crater, associated with a hematite spectral signature according to orbiter-derived data (Fraeman et al., Reference Fraeman, Arvidson, Catalano, Grotzinger, Morris, Murchie, Stack, Humm, McGovern, Seelos, Seelos and Viviano2013). The in-situ X-ray diffraction analysis shows that hematite is present in every Vera Rubin Ridge sample (Rampe et al., Reference Rampe, Bristow, Morris, Morrison, Achilles, Ming, Vaniman, Blake, Tu, Chipera, Yen, Peretyazhko, Downs, Hazen, Treiman, Grotzinger, Castle, Craig, Des Marais, Thorpe, Walroth, Downs, Fraeman, Siebach, Gellert, Lafuente, McAdam, Meslin, Sutter and Salvatore2020) but no blueberries are found within these rocks either.
Fe-oxide concretions found in Utah and Mongolia have been investigated as analogues of martian hematite spherules (Chan et al., Reference Chan, Beitler, Parry, Ormö and Komatsu2004; Yoshida et al., Reference Yoshida, Hasegawa, Katsuta, Maruyama, Sirono, Minami, Asahara, Nishimoto, Yamaguchi, Ichinnorov and Metcalfe2018). Terrestrial concretions have similar spherical shapes and compositions (Chan et al., Reference Chan, Beitler, Parry, Ormö and Komatsu2004; Sefton-Nash and Catling, Reference Sefton-Nash and Catling2008; Yoshida et al., Reference Yoshida, Hasegawa, Katsuta, Maruyama, Sirono, Minami, Asahara, Nishimoto, Yamaguchi, Ichinnorov and Metcalfe2018) though differences between their sizes and mineralogy can occur. Terrestrial concretions (centimetres to metres) are larger than martian blueberries (diameter <6.2 mm) (McLennan et al., Reference McLennan, Bell, Calvin, Christensen, Clark, de Souza, Farmer, Farrand, Fike, Gellert, Ghosh, Glotch, Grotzinger, Hahn, Herkenhoff, Hurowitz, Johnson, Johnson, Jolliff, Klingelhöfer, Knoll, Learner, Malin, McSween, Pocock, Ruff, Soderblom, Squyres, Tosca, Watters, Wyatt and Yen2005; Sefton-Nash and Catling, Reference Sefton-Nash and Catling2008). Goethite and quartz are major minerals (each present at ≤10 wt%) in terrestrial concretions (Yoshida et al., Reference Yoshida, Hasegawa, Katsuta, Maruyama, Sirono, Minami, Asahara, Nishimoto, Yamaguchi, Ichinnorov and Metcalfe2018), whereas martian blueberries are composed of pure hematite.
Hydrous sulphates
A range of hydrous sulphates occur on Mars (Mg-, Fe-, Al- and Ca sulphates) with Mg sulphate the most abundant. Monohydrated Mg sulphate (MgSO4⋅H2O) (identified as kieserite) and polyhydrated sulphates (interpreted as mostly MgSO4⋅4H2O (Wang et al., Reference Wang, Jolliff, Liu and Connor2016)) are the most-abundant hydrous sulphates on the martian surface according to remote-sensing studies (Fig. 6). Several-km-thick layers of monohydrated and polyhydrated sulphates have been observed in the Hesperian-aged equatorial regions, such as the Valles Marineris interior layered deposits (Ehlmann and Edwards, Reference Ehlmann and Edwards2014). Hydrated sulphates have also been found in the Noachian southern highlands (Wray et al., Reference Wray, Murchie, Squyres, Seelos and Tornabene2009; Reference Wray, Milliken, Dundas, Swayze, Andrews-Hanna, Baldridge, Chojnacki, Bishop, Ehlmann and Murchie2011, Wiseman et al., Reference Wiseman, Arvidson, Morris, Poulet, Andrews-Hanna, Bishop, Murchie, Seelos, Des Marais and Griffes2010; Ackiss and Wray, Reference Ackiss and Wray2014).

Fig. 6. Locations on Mars where hydrous sulphates are found by orbital remote sensing and by surface exploration missions. From (Wang et al., Reference Wang, Jolliff, Liu and Connor2016).
Other types of hydrated sulphates, Fe sulphates (e.g. jarosite, hydroxylated ferric sulphates, and szomolnokite) and Al sulphate (alunite) have been identified in highly localized regions of Mars. Large quantities of gypsum occur in the North Polar regions of Mars (Fishbaugh and Head, Reference Fishbaugh and Head2005; Langevin et al., Reference Langevin, Poulet, Bibring and Gondet2005; Fishbaugh et al., Reference Fishbaugh, Poulet, Chevrier, Langevin and Bibring2007; Horgan et al., Reference Horgan, Bell, Noe Dobrea, Cloutis, Bailey, Craig, Roach and Mustard2009), while other Ca sulphates have a more localized distribution (Wray et al., Reference Wray, Squyres, Roach, Bishop, Mustard and Dobrea2010; Ackiss and Wray, Reference Ackiss and Wray2014). The Fe- and Ca sulphates also seem to co-occur with monohydrated and polyhydrated Mg sulphates (Bishop et al., Reference Bishop, Parente, Weitz, Noe Dobrea, Roach, Murchie, McGuire, McKeown, Rossi and Brown2009; Lichtenberg et al., Reference Lichtenberg, Arvidson, Morris, Murchie, Bishop, Fernandez Remolar, Glotch, Noe Dobrea, Mustard and Andrews-Hanna2010; Wray et al., Reference Wray, Milliken, Dundas, Swayze, Andrews-Hanna, Baldridge, Chojnacki, Bishop, Ehlmann and Murchie2011; Weitz et al., Reference Weitz, Dobrea, Lane and Knudson2012; Ackiss and Wray, Reference Ackiss and Wray2014).
In-situ measurements made during surface-exploration missions—Vikings, Pathfinder, Mars Exploration Rovers (MER), Phoenix, and Mars Science Laboratory (MSL)—identified Mg-, Ca- and Fe3+ sulphates at all landing sites (Fig. 6). Additional sulphates were found at landing sites (e.g. in Gusev Crater, by the Spirit rover) whereas none was detected by orbital remote sensing. In particular, hydrous Mg-, Fe- and Ca sulphates were identified in the trenches and tracks made by Spirit rover's wheels (Wang et al., Reference Wang, Haskin, Squyres, Jolliff, Crumpler, Gellert, Schröder, Herkenhoff, Hurowitz and Tosca2006; Reference Wang, Bell, Li, Johnson, Farrand, Cloutis, Arvidson, Crumpler, Squyres and McLennan2008, Johnson et al., Reference Johnson, Bell, Cloutis, Staid, Farrand, McCoy, Rice, Wang and Yen2007; Arvidson et al., Reference Arvidson, Bell, Bellutta, Cabrol, Catalano, Cohen, Crumpler, Des Marais, Estlin and Farrand2010). Furthermore, volatiles (S and Cl) were identified in X-ray amorphous phases in all samples analysed by CheMin (using XRD technology) and SAM (using GC-MS technology) at Gale Crater. Some of these phases might be the alteration products of sulphates.
Remote-sensing observations suggest that most martian hydrated sulphates formed when a relatively large quantity of water was available during the Hesperian period. The chemistry of the hydrous sulphates, being Mg-rich and Fe-, Al-, and Ca-poor, with negligible Na and K, reflects a chemical weathering sequence of basaltic rocks, with higher aqueous dissolution rates for olivine compared to pyroxene, plagioclase and K-feldspar (Hurowitz et al., Reference Hurowitz, McLennan, Tosca, Arvidson, Michalski, Ming, Schröder and Squyres2006; McLennan et al., Reference McLennan, Bell, Calvin, Christensen, Clark, de Souza, Farmer, Farrand, Fike, Gellert, Ghosh, Glotch, Grotzinger, Hahn, Herkenhoff, Hurowitz, Johnson, Johnson, Jolliff, Klingelhöfer, Knoll, Learner, Malin, McSween, Pocock, Ruff, Soderblom, Squyres, Tosca, Watters, Wyatt and Yen2005). During hydrothermal episodes, the Ca sulphate veins found in the Endeavor Crater and Gale Crater may have formed (Squyres et al., Reference Squyres, Arvidson, Bell, Calef, Clark, Cohen, Crumpler, De Souza, Farrand and Gellert2012; Vaniman et al., Reference Vaniman, Martínez, Rampe, Bristow, Blake, Yen, Ming, Rapin, Meslin and Morookian2018). Although most hydrated sulphates have been found in the Hesperian terrains, the largest gypsum deposits discovered to date have been found on the Amazonian-aged north polar dunes, which were formed in a unique local environment (Langevin et al., Reference Langevin, Poulet, Bibring and Gondet2005). They have been interpreted to have either mineralized from local rock–water interactions or formed by the erosion of gypsum-bearing materials in the polar ice cap (Horgan et al., Reference Horgan, Bell, Noe Dobrea, Cloutis, Bailey, Craig, Roach and Mustard2009).
During Mars' more recent past to the present, hydrated sulphates on the surface and in the shallow subsurface have continuously been affected by obliquity changes and the planet's seasonal and diurnal cycles (Laskar et al., Reference Laskar, Correia, Gastineau, Joutel, Levrard and Robutel2004). For some sulphates, their degrees of hydration are dependent on the partial water vapour pressures (PH2O) (Kong et al., Reference Kong, Zheng, Hu, Wang, Ma and Sobron2018). Environmental changes on Mars can induce phase transformations of hydrous sulphates, such as those among neutral, acidic and basic Fe3+ sulphates (Wang and Ling, Reference Wang and Ling2011). Another potentially important process on present-day Mars is the plasma chemistry induced by martian dust activity. Laboratory simulations have demonstrated the dehydration, amorphization, and oxidation of hydrous sulphates (Wang et al., Reference Wang, Yan, Dyar, Houghton, Farrell, Jolliff, McLennan, Shi and Qu2020a).
Hydrated silicates
The identification of and search for clays on the martian surface has been a key aspect of Mars exploration, in terms of their remote identification by the OMEGA (Observatoire pour la Mineralogie, l'Eau, le Glace e l'Activité) and CRISM (Compact Reconnaissance Imaging Spectrometer for Mars) near-infrared orbiting instruments (Murchie et al., Reference Murchie, Mustard, Ehlmann, Milliken, Bishop, McKeown, Noe Dobrea, Seelos, Buczkowski and Wiseman2009), the selection and characterization of landing sites (Carter et al., Reference Carter, Poulet, Bibring, Mangold and Murchie2013), and science activities during landing missions. Phyllosilicates have been detected remotely in layered sediments but are also associated with impact cratering (Turner et al., Reference Turner, Bridges, Grebby and Ehlmann2016). Widespread phyllosilicates, particularly within the ancient highlands, include Fe-rich smectite (NaCa)0.5(Fe2+Mg)6(SiFe3+Al)8O20(OH)4, chlorite (FeMg)5Al(AlSi3)O10(OH)8 and serpentine (Mg, Fe)3Si2O5(OH)4. Fe-rich smectite/saponite (with its characteristic 2.3 μm absorption in CRISM data) was predicted for the Gale Crater central mound – Aeolis Mons (Thomson et al., Reference Thomson, Bridges, Milliken, Baldridge, Hook, Crowley, Marion, de Souza Filho, Brown and Weitz2011) before MSL's landing in 2012. Subsequently, CheMin XRD analyses have confirmed the presence of abundant smectite (e.g. Rampe et al., Reference Rampe, Ming, Blake, Bristow, Chipera, Grotzinger, Morris, Morrison, Vaniman and Yen2017).
Clay mineralogy and the evolution of the martian crust
A key result in understanding the formation of martian phyllosilicates is evidence that the abundance of clay in Gale Crater fine-grained sediments formed through low-temperature, <50 °C diagenesis – detrital grains such as olivine, plagioclase, etc., reacting with dilute groundwater in buried sediments (McLennan et al., Reference McLennan, Anderson, Bell, Bridges, Calef, Campbell, Clark, Clegg, Conrad and Cousin2014, Bridges et al., Reference Bridges, Schwenzer, Leveille, Westall, Wiens, Mangold, Bristow, Edwards and Berger2015). Strong evidence for diagenetic rather than detrital clay origins is given by the textures of sedimentary rocks, e.g. with nodules, veins and ridges (Léveillé et al., Reference Léveillé, Bridges, Wiens, Mangold, Cousin, Lanza, Forni, Ollila, Grotzinger and Clegg2014). This is a likely origin for much of the clay detected in the ancient highlands. On the basis of the MSL results, we now know that the presence of smectite-dominated clays and an association with layered sediments is an indication of an ancient habitable environment and stable hydrological cycle (Grotzinger et al., Reference Grotzinger, Gupta, Malin, Rubin, Schieber, Siebach, Sumner, Stack, Vasavada and Arvidson2015). The landing site of Mars2020, Jezero Crater, is partially filled with clay-bearing ancient deltaic deposits (Goudge et al., Reference Goudge, Milliken, Head, Mustard and Fassett2017). It will be important to ascertain if that clay is also predominantly of diagenetic origin, or, alternatively, if detrital clay input was important.
However, the most-closely studied martian clays to date (prior to the MSR mission) are within the nakhlite martian meteorites (Chatzitheodoridis et al., Reference Chatzitheodoridis, Haigh and Lyon2014). These have siderite, ferric saponite and ferric serpentine-rich veins (Lee and Chatzitheodoridis, Reference Lee and Chatzitheodoridis2016) in their olivine grains and mesostases (Changela and Bridges, Reference Changela and Bridges2010; Hicks et al., Reference Hicks, Bridges and Gurman2014). The saponite and serpentine formation postdates carbonate mineralization and occurred under near-neutral conditions, and at lower temperatures ~50 °C (Bridges and Schwenzer, Reference Bridges and Schwenzer2012). These meteorites may offer a clue about the origin of phyllosilicates associated with impact craters on Mars. Clays like those preserved in the nakhlites may result from impact-induced hydrothermal activity.
Methane on Mars
An understanding of methane in the martian atmosphere has been a challenge for the scientific community since the ´70s, when the Mariner 9 spectrometer IRIS provided the first hints of methane on Mars. The upper concentration limit was estimated at 20 ppb (Mège and Masson, Reference Mège and Masson1996). In 2003, methane detection changed dramatically. Observations made at the Keck Observatory (Keck II Telescope) and the Gemini South Observatory, and the NASA Infrared Telescope Facility (NASA IRTF) in Mauna Kea, Hawaii detected CH4 vibrational bands. In 2004, Krasnopolsky et al. (Reference Krasnopolsky, Maillard and Owen2004), then Formisano et al. (Reference Formisano, Atreya, Encrenaz, Ignatiev and Giuranna2004) reported the ground-based detection of methane in a mixing ratio of 10 ± 3 ppbv during the martian northern summer of 1999 using Fourier Transform Spectroscopy at the Canada–France–Hawaii Telescope.
Subsequently, Formisano et al. (Reference Formisano, Atreya, Encrenaz, Ignatiev and Giuranna2004) measured methane in the martian atmosphere using the Planetary Fourier Spectrometer (PFS) on board the Mars Express spacecraft. The average data from 16 orbits measuring the northern winter hemisphere in 2004 found local variations between 0 and 30 ppbv across the planet. The Mars Express data also showed the methane mixing ratio to slowly decrease from the northern spring to the southern summer with an average value of 14 ± 5 ppbv, and to coincide with the water vapour diurnal cycle on Mars (Geminale et al., Reference Geminale, Formisano and Giuranna2008). The northern mid-summer plume was calculated to contain ~19 000 metric tons CH4 and interpreted to be from different regions of Mars (Mumma et al., Reference Mumma, Villanueva, Novak, Hewagama, Bonev, DiSanti, Mandell and Smith2009). The TES on-board of Mars Global Surveyor over ~3 martian years (Clancy et al., Reference Clancy, Sandor, Wolff, Christensen, Smith, Pearl, Conrath and Wilson2000; Fonti and Marzo, Reference Fonti and Marzo2010) suggested a seasonal cycle of the methane, as well as inter-annual variations – increases over 32 ppbv with peak values over 60 ppm during the northern summer and autumn, and a decrease during the winter. A subsequent study using the Mars Express PFS (Geminale et al., Reference Geminale, Formisano and Sindoni2011) also documented an increase of methane to a mixing ratio of 34 ppbv over the north polar ice cap during the summer, which cannot be explained by global circulation. Geminale et al. (Reference Geminale, Formisano and Sindoni2011) suggested that there could be a methane reservoir associated with the polar ice cap, producing in one year 16 000–24 000 tons CH4, and the methane lifetime could be of the order of 4–6 martian years. Further ground-based observations using the CSHELL (Cryogenic Echelle Spectrograph) and NIRSpec (Near InfraRed Spectrograph) confirmed a far lower maximum limit of methane at 8 ppbv (Zahnle et al., Reference Zahnle, Freedman and Catling2011; Krasnopolsky, Reference Krasnopolsky2012; Villanueva et al., Reference Villanueva, Mumma, Novak, Radeva, Käufl, Smette, Tokunaga, Khayat, Encrenaz and Hartogh2013).
More recently, surface-based measurements of the atmosphere by Curiosity, performed for 605 martian sols in the period 2012–2014, measured methane with a background concentration of 0.69 ± 0.25 ppbv (Webster et al., Reference Webster, Mahaffy, Atreya, Flesch, Mischna, Meslin, Farley, Conrad, Christensen and Pavlov2015). Occasional spikes were also measured up to 7.2 ±2. 1 ppbv in Gale Crater by the Tunable Laser Spectrometer of SAM. This led to the interpretation that the seasonal variation of methane was 0.24–0.65 ppbv from 2014 to 2017 (Webster et al., Reference Webster, Mahaffy, Atreya, Moores, Flesch, Malespin, McKay, Martinez, Smith and Martin-Torres2018). This seasonal variation is also consistent with other atmospheric gas variations in CO2, H2O, O2 and CO (Trainer et al., Reference Trainer, Wong, McConnochie, Franz, Atreya, Conrad, Lefèvre, Mahaffy, Malespin and Manning2019). However, the magnitude of the seasonal variation in the CH4 background is greater than the other long-lived atmospheric volatiles. This variation may be related to geological and/or meteorological effects, such as losses from photochemistry, sputtering, escape or condensation under any of the conditions reached during the current seasonal cycle (Trainer et al., Reference Trainer, Wong, McConnochie, Franz, Atreya, Conrad, Lefèvre, Mahaffy, Malespin and Manning2019). It should be noted that the Curiosity does not have any instrument that can distinguish between biological and geological sources of methane.
The origin of methane
On Earth, 95% methane is produced biogenically, by microbial methanogens (Atreya et al., Reference Atreya, Mahaffy and Wong2007). The process begins with the oxidation of dihydrogen by hydrogenase (equation (1)), followed by the reduction of CO2 (equation (2)) (Thauer, Reference Thauer1998). Methanogens generate energy via the reductive acetyl CoA (Wood–Ljungdahl) pathway, and it is during this process that methane is produced as a by-product (Parkes et al., Reference Parkes, Linnane, Webster, Sass, Weightman, Hornibrook and Horsfield2011). Such microorganisms are classified as chemolithoautotrophs because they make their own source of energy by breaking bonds within inorganic molecules (equation (1) and (2)) and can fix CO2.


Dihydrogen gas in Equation (1) can also form abiotically as in Equation (3), or degas from erupting volcanoes on Earth.

The only way to ascertain the existence of life elsewhere in our Milky Way Galaxy is via spectral signals (Jheeta, Reference Jheeta2013). Methane can be generated both abiotically and biotically. It can be made abiotically by serpentinization (Equation (4)). It should be noted that to produce one molecule of methane abiotically requires 26 molecules of water (Equation (4)). Present-day Mars is extensively depleted of water. This may be the reason why the methane plumes that are produced on Mars are only occasional spikes.

Methanogens have two very important attributes, namely they are thought to be the most-primitive organisms on present-day Earth; and they are anaerobic hardy microorganisms able to withstand extreme temperatures and acidities. Such conditions were probably more common on the early Earth. Martian life may be present below the surface, especially if similarly robust microorganisms are harboured within an anaerobic subsurface lake (Westall et al., Reference Westall, Foucher, Bost, Bertrand, Loizeau, Vago, Kminek, Gaboyer, Campbell and Bréhéret2015). This however requires that these microorganisms can utilize dihydrogen in order to produce methane by the reduction of CO2. In anaerobic lakes, there may not be enough dihydrogen for this process which would seep into the atmosphere and then escape to space. For the detection of life on an exoplanet there needs to be a continuous supply of a biogenic gas such as methane maintained at certain levels rather than sporadic tenuous degassing from a planet's surface, as is the case with Mars. Thus, intermittent plumes of methane are more consistent with an abiotic origin.
Recent investigation on the photochemical synthesis of CH4 by the reduction of CO2 over acidic minerals (Shkrob et al., Reference Shkrob, Marin, He and Zapol2012), including a wide range of clays found on both the surface of Mars and in martian meteorites such as Nakhla (Civiš et al., Reference Civiš, Knížek, Ivanek, Kubelík, Zukalová, Kavan and Ferus2017, Reference Civiš, Knížek, Rimmer, Ferus, Kubelík, Zukalová, Kavan and Chatzitheodoridis2018), can explain not only the formation of methane, but also of perchlorates and chlorinated organic compounds. It can also account for the seasonal variations of methane and carbon monoxide concentrations in the atmosphere (Civiš et al., Reference Civiš, Knížek, Ivanek, Kubelík, Zukalová, Kavan and Ferus2017, Reference Civiš, Knížek, Rimmer, Ferus, Kubelík, Zukalová, Kavan and Chatzitheodoridis2018). Additional abiotic surface sources involve the decomposition of organics delivered to the martian surface by fragments of comets and asteroids (Fries et al., Reference Fries, Christou, Archer, Conrad, Cooke, Eigenbrode, ten Kate, Matney, Niles and Sykes2016), volcanic outgassing (Craddock and Greeley, Reference Craddock and Greeley2009), and slow photo-catalytic decomposition of carboxylated molecules in kerogen by photo-Kolbe reactions with Fe(II) oxides in the martian regolith (Shkrob et al., Reference Shkrob, Chemerisov and Marin2010). Finally, the formation of subsurface methane in high pressure-temperature hydrothermal fluids (Welhan, Reference Welhan1988), deep subsurface aquifers (Hu et al., Reference Hu, Bloom, Gao, Miller and Yung2016), and the serpentinization of olivine (Oze and Sharma, Reference Oze and Sharma2005) cannot be ruled out.
The habitability of Mars
Was Mars ever inhabited?
On the question of whether Mars was inhabited, there are two points that we should consider and factor into the experimental search for martian life. We tend to focus closely on the question whether Mars is or was inhabited. However, although it will be difficult to show a definitive lack of life anywhere on Mars, if we eventually explore many potentially habitable environments and find no evidence of life then this would suggest that the planet has been lifeless (Cockell and McMahon, Reference Cockell and McMahon2019). This finding would be profound. If a planet that was similar to early Earth 4 billion years ago was sterile, yet Earth went on to host about 1029 microbes (Kallmeyer et al., Reference Kallmeyer, Pockalny, Adhikari, Smith and D'Hondt2012), this would be more difficult to explain than finding life on another planet that began in a very similar state to Earth. We should remember not to get too focused on a quest to find life on Mars, but rather maintain our interest in testing the hypothesis of life on Mars, either outcome of which would be important.
However, was there life around to take advantage of these habitable conditions? We do not know enough about the origin of life to be able to provide a definitive answer to this (Michalski et al., Reference Michalski, Onstott, Mojzsis, Mustard, Chan, Niles and Johnson2018), nor do we know, despite promising impact-shock experiments, whether panspermia could have successfully occurred between Earth and Mars if Mars was not suitable for an origin of life (Horneck et al., Reference Horneck, Stöffler, Ott, Hornemann, Cockell, Moeller, Meyer, De Vera, Fritz and Schade2008). Further advances in origins of life research on Earth may well elucidate whether Mars had environments suitable for an origin of life, but even if these insights return affirmative answers, that does not demonstrate that this process did occur on Mars. Advances in our understanding of the origin of life and experimental tests of panspermia in shock experiments can never answer definitively the question posed, only provide information that will help us explain what we ultimately observe on the planet itself. We need to go to Mars to unambiguously detect biosignatures.
What if Mars was never habited?
A habitable, but lifeless Mars would raise important questions. Some people regard such a state as logically impossible – that we cannot define a place as being habitable without the presence of life, but this is incorrect. Microbiologists all the time make agar plates that are habitable to known organisms but are uninhabited (uninhabited habitats). We might find environments on Mars that have all the requirements for known forms of life (such as methanogens), but those environments were never inhabited, either because Mars was always uninhabited, or if it was inhabited, transient habitable environments came and went without being colonized by existing life. Such environments can be demonstrated even on the fecund Earth (Cockell, Reference Cockell2020). These facts do not necessarily bear on the search for biosignatures, but they are important to bear in mind since the paradigm that where there are habitable conditions there is life, which is generally true in most habitable environments on the Earth, may not hold on other planets and clearly would not be true on a planet where there had never been life. Investigating habitable, but uninhabited environments is difficult because one is looking for definitive evidence of the lack of life, which could either be the lack of a biosignature or the presence of some condition that requires a lack of life (such as the presence of some trace element or nutrient that is always depleted by life, and whose presence would truly be an anti-biosignature; however, examples of such signatures are difficult to identify). The search for uninhabited habitats raises these observations and experimental complexities.
At the current time, it is not possible to provide a definitive answer to the question, but we do know that the presence of habitable conditions on Mars makes this question a viable and important one to answer.
Microfossils on Mars
A ‘biomarker’ on Mars could be in the form of microfossils – fossilized individual or aggregates of microbial cells. A challenge for future exploratory missions would be the unambiguous identification of such features in martian bedrock. In the context of the search for life on Mars, or the earliest records of life on Earth, the term ‘microfossil’ is generally understood to mean the material remnants of a microbial cell, with recognizably cellular morphology, most commonly preserved as insoluble organic matter, or ‘kerogen’. In this sense, there can be no ‘microfossil’ without fossil morphology.
Can mineralogy, organic chemistry and/or isotopes act as reliable biomarkers without morphology? The specific clarifying examples of isotopes, organic chemistry and mineralogy as potential exceptions might be addressed as follows. In the most-general sense, it could be argued that all three of these phenomena are rooted in form, that is, a spatial ordering of the physical world by life. From this point of view, the ‘exceptions’ are irrelevant. Certainly, this is true of organic chemistry – purely molecular evidence for biogenicity would occur either as a molecule or set of molecules of sufficient structural complexity that no abiotic mechanism can be established for their synthesis, or a set of molecules with a ‘repeating’ pattern of different, but related structures.
Minerals are defined in large part by their crystal structure. As such, any biological impact on mineralogy is an expression of form. From another point of view, the intriguing model of ‘mineral evolution’ advanced by Hazen et al. (Reference Hazen, Papineau, Bleeker, Downs, Ferry, McCoy, Sverjensky and Yang2008) maintains that much of the mineral diversity on the modern Earth emerged as at least an indirect result of biology (after biologically mediated accumulation of free oxygen in Earth surface environments). Through this lens, any mineral whose evolution required abundant free oxygen is a biosignature. Would the presence of such a mineral, on its own, be sufficient as definitive proof of an inhabited extraterrestrial planet? This is likely not so. It is certainly possible, though, that a very strong case for the past influence of biology could be made on the basis of a terrestrial planet's mineralogy, but mineralogy alone would likely be insufficient. Biomineralization on Earth produces diverse and diagnostic forms (e.g. in carbonates or iron oxides) that are unknown in abiotic systems, but in these cases, chemical formulae and structure of unit cells are the same as minerals formed abiotically.
Considering isotopic fractionation as an expression of form, there are thresholds of isotopic fractionation in certain Earth systems that are generally accepted as strongly – even conclusively – indicative of biology. Sedimentary organic matter with apparent carbon isotope fractionations (i.e. relative to co-occurring carbonate minerals reasonably expected to record the isotopic composition of inorganic carbon at the time of organic synthesis) >40‰, and sulphide minerals with δ 34S well outside the range between −10 and +10‰ are both strong indicators of biology on Earth. The comparative lack of knowledge about carbon- and sulphur cycles on Mars would make any isotopic observation, on its own, more ambiguous. There is a good reason to believe, however, that a similar abiotic fractionation process acted on Earth and Mars, and large isotopic fractionations between co-occurring oxidized and reduced species containing biologically important elements, expressed in a geologic context consistent with habitability, should be considered as strong potential biosignatures.
Organics on Mars
Organics discovered from direct analysis of sediments of the martian surface include chlorine (Ming et al., Reference Ming, Archer, Glavin, Eigenbrode, Franz, Sutter, Brunner, Stern, Freissinet, McAdam, Mahaffy, Cabane, Coll, Campbell, Atreya, Niles, Bell, Bish, Brinckerhoff, Buch, Conrad, Des Marais, Ehlmann, Fairén, Farley, Flesch, Francois, Gellert, Grant, Grotzinger, Gupta, Herkenhoff, Hurowitz, Leshin, Lewis, McLennan, Miller, Moersch, Morris, Navarro-González, Pavlov, Perrett, Pradler, Squyres, Summons, Steele, Stolper, Sumner, Szopa, Teinturier, Trainer, Treiman, Vaniman, Vasavada, Webster, Wray and Yingst2014) and sulphur-bearing macromolecular organics (Eigenbrode et al., Reference Eigenbrode, Summons, Steele, Freissinet, Millan, Navarro-González, Sutter, McAdam, Franz and Glavin2018). In martian meteorites (currently the only martian samples on Earth), nitrogen-bearing organics from the 4-Ga-old martian meteorite, Allan Hills 84 001, are found in carbonates (Koike et al., Reference Koike, Nakada, Kajitani, Usui, Tamenori, Sugahara and Kobayashi2020). Macromolecular reduced carbon in other martian meteorites has also been identified (Steele et al., Reference Steele, McCubbin and Fries2016).
The SAM instrument (Freissinet et al., Reference Freissinet, Glavin, Mahaffy, Miller, Eigenbrode, Summons, Brunner, Buch, Szopa and Archer2015; Steele et al., Reference Steele, McCubbin and Fries2016) onboard the MSL first measured indigenous organics on Mars above background levels. Analyses of the Sheepbed mudstone in Gale Crater – a sediment with ~20 wt.% smectite clay interpreted to represent a lacustrine environment – contains chlorine-bearing organic molecules, such as chlorobenzene (C6H5Cl; 150–300 ppb) and in much smaller amounts, dichloroalkanes (e.g. propane C3H6Cl2, ethane C2H4Cl2 and butane C4H8Cl2) in soil samples from the Cumberland drill hole, interpreted as either indigenous or pyrolyzed by reactions between martian aromatic or aliphatic molecules with oxychlorine phases. Sulphur-bearing organics were also identified by SAM in old mudstone fluviodeltaic sediments from the Murray formation at Pahrump Hills in Gale Crater including thiophenes (i.e. C4H4S, C5H6S and C8H6S) and thiols (CH4S and C2H6S). Aliphatics (chains of one- to five carbons) and aromatic hydrocarbons (benzenes) were among the detected volatiles above the detection limit of the instruments.
Chlorination or sulphurization could be related to abiotic secondary processes on Mars and serve as a means to prevent organics breaking down under intense radiation on the martian surface. Nitrogen-bearing organics in ALH 84001 also have abiotic origins consistent with conditions on Hadean Mars (Koike et al., Reference Koike, Nakada, Kajitani, Usui, Tamenori, Sugahara and Kobayashi2020). More conclusive evidence is expected when the deeper subsurface of Mars is analysed. The Rosalind Franklin rover in Oxia Planum will drill samples 2 m into the subsurface (Martian meteorites and sample return section). The Perseverance rover in Jezero Crater with the Raman and fluorescence SHERLOC spectrometer will search for organics within their mineralogical context. Perseverance is also the first mission caching samples for return back to Earth (Martian meteorites and sample return section). Careful sampling of a variety of geochemical environments and origins will be required. Hydrothermal alteration and vacant areas inside the volume of clays where water activity is higher can not only trap but can also catalyse the synthesis of organics from simpler precursors. Such clays are found in martian meteorites, such as in Nakhla (Chatzitheodoridis et al., Reference Chatzitheodoridis, Haigh and Lyon2014) which contain geochemical micro-environment niches where saponite clays form in similarity with, for example, saponite found in basalts near the Mid-Atlantic Ridge of the Atlantis Massif, in which aromatic amino-acids form abiotically (Ménez et al., Reference Ménez, Pisapia, Andreani, Jamme, Vanbellingen, Brunelle, Richard, Dumas and Réfrégiers2018).
Disambiguating biogenic and abiotic origins
A biogenic interpretation of organic compounds discovered on Mars based on chemical composition alone is ambiguous; organic chemistry is not exclusive to biology. However, homochirality is a uniquely biological trait. For example, if amino acids were discovered on Mars, a left-handed homochirality would indicate a biological origin based on what we know of life's biochemistry on Earth. An organic biomarker could be preserved within a definitive biomorphic structure such as a microfossil (see above). Biogenic organic material also preferentially retains the lighter stable isotope of 12C. Organic material with any of these properties has yet to be discovered on Mars.
On Earth, organic material is mostly biogenic. An example of an exception is the abiotic organic material delivered to Earth by fragments of asteroids and comets as dust and meteorites. Interplanetary dust would have also delivered an order of magnitude more of organic carbon to Mars than to Earth (Flynn, Reference Flynn1996). The surface of Mars is also peppered by impact craters. Elevated organic distributions around impact craters on Mars could trace their meteoric origins (Frantseva et al., Reference Frantseva, Mueller, ten Kate, van der Tak and Greenstreet2018). Higher fractions of stable isotopes heavier than those found in putative biomolecules (e.g. D, 13C and 15N) could also imply origins exogenous to Mars. This means that as well as endogenic processes, exogenous delivery should be considered when disentangling abiotic organic evolution on the surface of Mars.
Putative martian life might have escaped extinction
The endolithic niche is an important target of the search for life elsewhere in the Solar System. Specialized microbes able to exploit this habitat on Earth by developing in the airspaces of rocks are protected from intense solar radiation and desiccation, allowing their population to persist even in the most-extreme terrestrial climates (Walker et al., Reference Walker, Spear and Pace2005). Endolithic microorganisms are often the predominant form of life in hot- and cold deserts (Coleine et al., Reference Coleine, Stajich, de Los Ríos and Selbmann2021), including the McMurdo Dry Valleys of Antarctica characterized by extreme cold- and arid conditions, along with the intense UV irradiation reaching ground level (Onofri et al., Reference Onofri, Selbmann, Zucconi and Pagano2004; Bernhard and Stierle, Reference Bernhard and Stierle2020). Therefore, they have value as Mars analogue sites (Friedmann, Reference Friedmann1982; Selbmann et al., Reference Selbmann, Pacelli, Zucconi, Dadachova, Moeller, de Vera and Onofri2018).
Endolithic life-forms of the Antarctic desert are used as proxies for putative life on planets such as Mars. If putative martian life ever evolved, it may have found a last refuge within the rock pores during the cooling and drying over the early history of the Red Planet (Wierzchos et al., Reference Wierzchos, Cámara, de Los Rios, Davila, Sánchez Almazo, Artieda, Wierzchos, Gomez-Silva, McKay and Ascaso2011).
Implications for interplanetary life transfer
Studies on these endolithic communities are pertinent to the concept of lithopanspermia (i.e. the interplanetary transport of microbial passengers inside rocks). Petrographic analyses of martian meteorites indicate that, if during ejection from Mars these rocks experienced shock in the range from 5 to 55 GPa (Artemieva and Ivanov, Reference Artemieva and Ivanov2004; Stöffler et al., Reference Stöffler, Horneck, Ott, Hornemann, Cockell, Moeller, Meyer, de Vera, Fritz and Artemieva2007) and heating in the range from 40 to 350 °C, some of them have never been exposed to temperatures above 100 °C (Weiss et al., Reference Weiss, Kirschvink, Baudenbacher, Vali, Peters, Macdonald and Wikswo2000; Shuster and Weiss, Reference Shuster and Weiss2005). Therefore, some fragments of martian crust can be boosted into space whilst experiencing only relatively mild pressure/temperature shocks. Entering into the atmosphere of the recipient planet exposes the meteors to additional fragmentation because speeds, reaching 10–20 km s–1, lead to frictional heating melts of the surface. Yet, the short transit time of meteors through the atmosphere, of a few seconds, precludes penetration of heat more than a few millimetres below the surface, limiting the strong heating to <1 mm of depth (meteorite fusion crust) (Fajardo-Cavazos et al., Reference Fajardo-Cavazos, Link, Melosh and Nicholson2005).
Space conditions appear to be the main limit for microbial interplanetary transit; besides, endolithic microorganisms are highly extremotolerant life-forms, able to survive a wide range of injuries, including extremes of temperature, vacuum and high doses of UV and ionizing radiation partially simulating cosmic radiation (up to 60 kGy 60Co) (Selbmann et al., Reference Selbmann, Pacelli, Zucconi, Dadachova, Moeller, de Vera and Onofri2018) or Fe ions up to 1000 Gy (Aureli et al., Reference Aureli, Pacelli, Cassaro, Fujimori, Moeller and Onofri2020). They also are able to withstand periods as long as 1.5 years in Earth Low Orbit (Onofri et al., Reference Onofri, de la Torre, de Vera, Ott, Zucconi, Selbmann, Scalzi, Venkateswaran, Rabbow and Sánchez Iñigo2012; Selbmann et al., Reference Selbmann, Zucconi, Isola and Onofri2015; Billi et al., Reference Billi, Staibano, Verseux, Fagliarone, Mosca, Baqué, Rabbow and Rettberg2019). If shielded and protected by solar irradiation, spores from the (non-endonlithic) bacteria Bacillus subtilis and B. pumilus can survive under Mars-surface simulated conditions (Horneck et al., Reference Horneck, Klaus and Mancinelli2010, Reference Horneck, Moeller, Cadet, Douki, Mancinelli, Nicholson, Panitz, Rabbow, Rettberg, Spry, Stackebrandt, Vaishampayan and Venkateswaran2012; Cortesão et al., Reference Cortesão, Fuchs, Commichau, Eichenberger, Schuerger, Nicholson, Setlow and Moeller2019). Furthermore, Mars' surface is not necessarily biocidal even for non-sporulating microorganisms (Hallsworth, 2021). Sporulation is a process which appeared early in the development of life on Earth (Tocheva et al., Reference Tocheva, Ortega and Jensen2016). In addition to aerobic bacilli, present-day endospore formers include anaerobic bacteria, such as the Clostridium genus. Endospore-forming bacteria are candidates to survive extreme environments during interplanetary transit (Nicholson and Ricco, Reference Nicholson and Ricco2019). The evidences above show that the planets in our Solar System might not be biologically isolated, because potential micronauts (both prokaryotes and eukaryotes) may have undergone and survived interplanetary transfer.
Microorganisms resilient to radiation exposure on the Mars surface
Today, ultraviolet- and ionizing radiation through Mars' tenuous atmosphere would seemingly lead to an inhospitable environment for the survival of many kinds of microorganism on the martian surface. However, under some circumstances microbial cells may be able to survive at/near the martian surface (Hallsworth, Reference Hallsworth2021). Pigmented microorganisms will have better chances of survival because pigments such as melanin are known to be able to absorb/dissipate electromagnetic radiation such as UV and ionizing radiation. The presence of melanin correlates with increased survival and enhanced robustness, resulting in an advantage for melanized organisms over non-melanized ones under harsh conditions. In this regard, melanized fungi are a good example of the survival advantage conferred by melanin. There is a high incidence of melanized fungi in such extreme environments, such as the damaged nuclear reactor at Chernobyl (Zhdanova et al., Reference Zhdanova, Lashko, Redchits, Vasilevskaia, Borisiuk, Siniavskaia, Gavriliuk and Muzalev1991; Dighton et al., Reference Dighton, Tugay and Zhdanova2008) with 80% of the fungal species recovered from the reactor being melanized. Another example are the rocky deserts of Antarctica (Selbmann et al., Reference Selbmann, Zucconi, Isola and Onofri2015). It has also been demonstrated that melanized fungi can survive simulated Mars-like conditions (Onofri et al., Reference Onofri, Barreca, Selbmann, Isola, Rabbow, Horneck, de Vera, Hatton and Zucconi2008) and cosmic radiation while exposed on the Mir Spacecraft (Novikova, Reference Novikova2004). Besides exposure to high doses of ionizing and/or UV radiation, inhospitable environments can deliver additional forms of stress such as salinity, aridity, rapid and extreme temperature fluctuations, as well as little-to-no nutrients. The advantage that melanin presence confers on fungi is based, first of all, on its ability to act as a physical shield, reducing the relative biological effectiveness of ionizing radiation and reducing its potential for damaging living cells. Secondly, melanin protects the organism further by ‘scavenging’ reactive oxygen species generated by ionizing radiation (reviewed in Malo and Dadachova (Reference Malo and Dadachova2019)). Thirdly, melanin can convert solar radiation to heat. Thus, physical and chemical protective qualities of melanin can result in increased cell survival, and maybe even favour metabolic activity (and even growth) of melanized species under some conditions close to those that can occur on the martian surface. Analyses of the martian atmosphere show that the temperature : water-activity regimes would not allow metabolic activity of any known terrestrial microorganisms (Hallsworth et al., Reference Hallsworth, Koop, Dallas, Zorzano, Burkhardt, Golyshina, Martín-Torres, Dymond, Ball and Mckay2021).
Robotic and remote exploration of Mars
Mars Science Laboratory
The MSL Curiosity rover mission achieved a milestone in Mars exploration, landing a car-sized payload on Mars, manoeuvring through Gale Crater, climbing the flank of Aeolis Mons (informally known as Mount Sharp) and performing unprecedented science on the way. Curiosity's traverse path has been determined by both science and safety considerations.
Curiosity's traverse path is determined by science priorities across a variety of spatial and temporal scales. The primary goal for the MSL mission is to explore and quantitatively assess a local region on Mars' surface as a potential habitat for life, past or present. Gale Crater was selected as the landing site for the MSL mission in part because it contains a 5 km tall mound of stratified rocks (known as Aeolis Mons) with intriguing mineral signatures that may record changes in environmental history (c.f. (Milliken et al., Reference Milliken, Grotzinger and Thomson2010; Golombek et al., Reference Golombek, Grant, Kipp, Vasavada, Kirk, Fergason, Bellutta, Calef, Larsen and Katayama2012; Grotzinger et al., Reference Grotzinger, Crisp, Vasavada, Anderson, Baker, Barry, Blake, Conrad, Edgett and Ferdowski2012)). In order to meet this mission objective within Gale Crater, the traverse path is focused on the long-term goal of ascending the lower flanks of Aeolis Mons, with intermediate traverse decisions determined by focused science campaigns, and daily traverse decisions based on opportunistic science.
Curiosity landed on Bradbury Rise, initially mapped as hummocky terrain within the landing ellipse. This area is separated from Aeolis Mons by a large dune field known as the Bagnold Dunes. While the primary scientific goal is to investigate strata at the base of Aeolis Mons, due to traversability constraints the rover had to drive more than 12 km to the southwest to reach a gap in the dunes. The dunes were well-mapped prior to landing, so the general traverse path for the rover to ascend Aeolis Mons was also known since before landing.
While the long-term path has been predetermined, there are intermediate stops along the way based on focused science campaigns. An early example is the exploration of ‘Yellowknife Bay’, a region just to the east of the landing site that represents the intersection of three distinct geologic units as mapped from orbit. Although the long-term traverse path suggested that driving to the southwest was the way to ascend Aeolis Mons, the team decided to divert from the most efficient path to investigate this area first. This decision paid off. Yellowknife Bay lies at the distal extent of a large alluvial fan that extends from the northern crater rim; once the rover investigated the fine-grained fractured outcrops, we discovered that they represent ancient lake deposits. These lake deposits contain all of the chemical ingredients necessary for life and minerals that would have provided a source of energy for primitive organisms, so this campaign resulted in evidence for the first habitable environment explored by the rover (Grotzinger et al., Reference Grotzinger, Gupta, Malin, Rubin, Schieber, Siebach, Sumner, Stack, Vasavada and Arvidson2015). Since then, Curiosity has explored about a dozen key waypoints for more detailed science campaigns. Some of these campaigns were motivated by orbital observations (e.g. Ehlmann et al., Reference Ehlmann, Edgett, Sutter, Achilles, Litvak, Lapotre, Sullivan, Fraeman, Arvidson and Blake2017; Rice et al., Reference Rice, Gupta, Treiman, Stack, Calef, Edgar, Grotzinger, Lanza, Le Deit and Lasue2017; Bennett et al., Reference Bennett, Fox, Bryk, Fedo, Vasavada, Dehouck, Thompson, O'Connell-Cooper, Bristow and Millan2019; Fraeman et al., Reference Fraeman, Edgar, Rampe, Thompson, Frydenvang, Fedo, Catalano, Dietrich, Gabriel and Vasavada2020), while others were based on discoveries on the ground (e.g. Banham et al., Reference Banham, Gupta, Rubin, Watkins, Sumner, Edgett, Grotzinger, Lewis, Edgar and Stack-Morgan2018; Edgar et al., Reference Edgar, Gupta, Rubin, Lewis, Kocurek, Anderson, Bell, Dromart, Edgett and Grotzinger2018; Stack et al., Reference Stack, Grotzinger, Lamb, Gupta, Rubin, Kah, Edgar, Fey, Hurowitz and McBride2019).
Finally, on the short-term scale, daily traverse decisions are influenced by science through selection of the ‘end-of-drive’ location. On any given day, the science team works with the engineers to select drive locations that will provide adequate outcrops for future science analyses, or that provide a good perspective on the stratigraphy. The team is keeping an eye on the long-term path, but this is still a mission of exploration, and is ready to respond to findings as they arise.
Mars 2020
The Mars 2020 mission consisting of the Perseverance rover and Ingenuity drone landed successfully in Jezero Crater. The mission is dedicated towards geology and astrobiology. Ingenuity performed the first ever flight from the surface of another planet.
Jezero is an impact crater ~45 km in diameter, located at 18.4°N, 77.7°E in the Nili Fossae region, forming a fluvial sedimentary basin (Goudge et al., Reference Goudge, Mustard, Head, Fassett and Wiseman2015). The landing site is close to the deltas of rivers that once flooded the crater. Aeolian sediments are also present, laying onto a volcanic floor. Multiple short flooding events composed of a variety of detrital sediments transported from very different provenances are suggested to have deposited the north and western watershed fans, increasing the probability of identifying materials of different origins accumulated around the landing site of Perseverance. This could have astrobiological implications, but is also important for understanding climatic processes on Mars such as the regularity of floods, its connection to periodic climatic epochs, and their possible connection to impact events. Apart from the transported and deposited sediments, authogenic clays might be also located and sampled; it is known that clays can record climatic conditions and also trap and preserve organics that are putative biosignatures, either chemical or textural. The close inspection of the samples in situ or in laboratories after sample return will enable the search for biosignatures or pseudo-biosignatures. Simulations run in the Mojave Desert have shown that PIXL (Planetary Instrument for X-ray Lithochemistry) can resolve trace element patterns that could be used to select samples for geochronology (Martin et al., Reference Martin, Ehlmann, Thomas, Wiens, Hollis, Beegle, Bhartia, Clegg and Blaney2020). The same applies to the identification of primary mineral phases and volcanic glasses. Resolving secondary mineral phases (i.e. carbonates, clays, sulphates) will be important to elucidate past environmental conditions. Combining all the above instruments will be paramount to identifing undisputed life on Mars.
On board the Perseverance rover, there are various optical cameras and microscopes for navigation, analysis and closer characterization. Navigation and remote identification of areas of interest is performed with the Navcam stereo camera, the Mastcam-Z hyperspectral imager and the SuperCam imaging and analytical system (remote Raman and Laser-Induced Breakdown Spectroscopy (LIBS) analysis). Chemical and mineralogical analyses can be performed by all spectroscopy instruments, with the advantage of organic compound detection and mapping with the Scanning Habitable Environments with Raman & Luminescence for Organics & Chemicals (SHERLOC) instrument (Martin et al., Reference Martin, Ehlmann, Thomas, Wiens, Hollis, Beegle, Bhartia, Clegg and Blaney2020). SHERLOC operates with a deep UV laser beam which can be scanned over a small area of a sample, providing mineralogical mapping over a 7 × 7 mm area with a spot size of 100 μm, also captured with the WATSON colour imaging system (Williford et al., Reference Williford, Farley, Stack, Allwood, Beaty, Beegle, Bhartia, Brown, de la Torre Juarez and Hamran2018). At the same time, SHERLOC acquires fluorescence images, enabling the identification and classification of the composition as well as the mapping of the spatial distribution of organic compounds. The detection sensitivity of organics can go down to 10−5 to 10−6 w/w over the entire scanned area. Both instruments are set up to investigate the habitability of the explored area based on the availability of CHNOPS chemistry. Mineral distribution patterns together with organic compounds will enable the identification of putative textural biosignatures.
Tienwen-1
The CNSA's Tienwen-1 spacecraft planetary mission is a threefold mission, involving an orbiter, a lander and the Zhurong rover. The Tienwen-1 lander and Zhurong rover landed on Mars on 14 May 2021. Zhurong deployed from the lander and touched the martian surface on 22 May 2021 in Utopia Planitia, a plain located within the Utopia impact basin (Fig. 7). The geological environment of Utopia Planitia is being studied, with a focus on volatiles and water in the region.
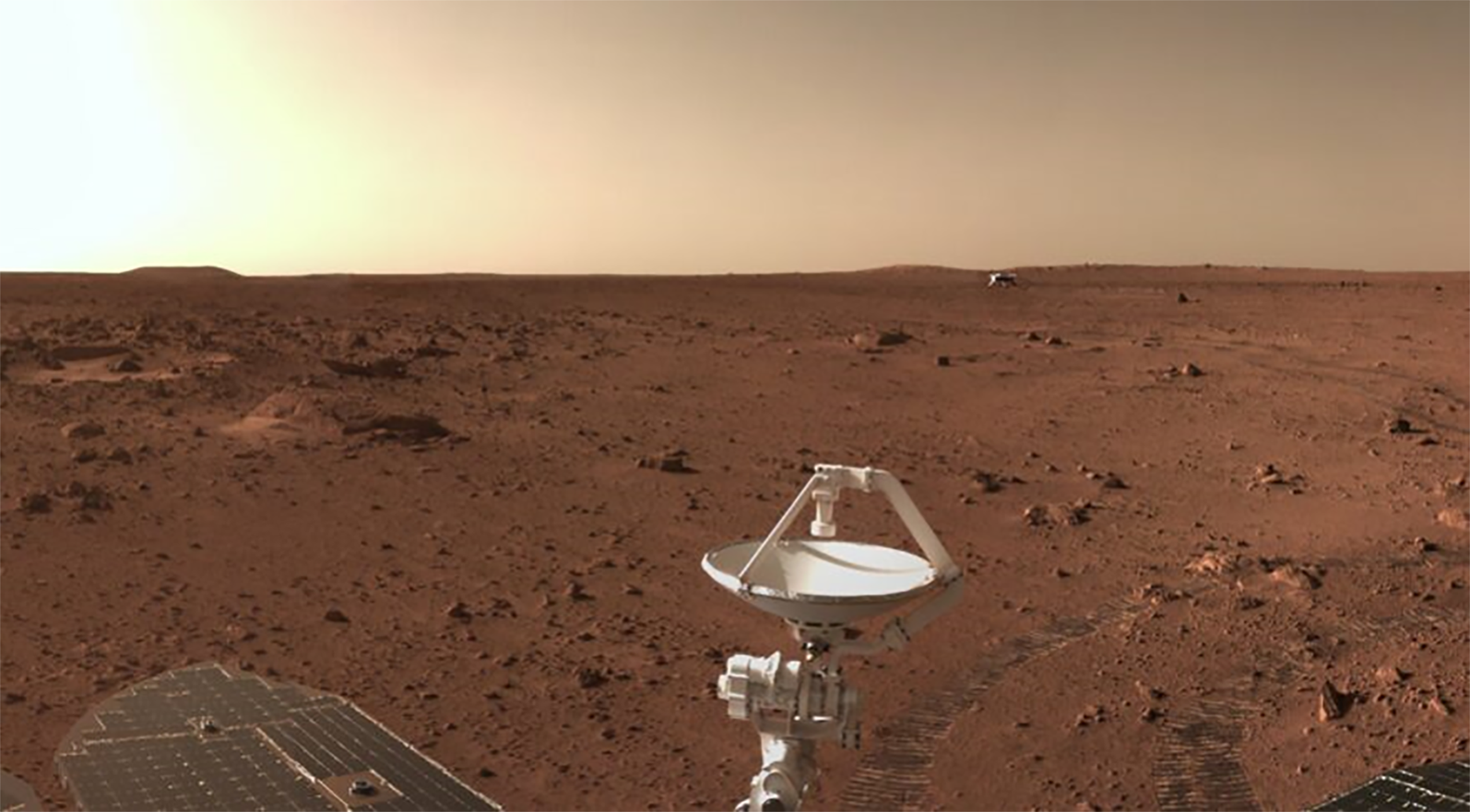
Fig. 7. The landscape of Mars at Utopia Planitia taken with the Zhurong rover's Navigation and Topography Cameras.
Utopia Planitia was formed during the Late Hesperian to Mid-Amazonian period, with mainly volcanic rocks and glacial clastic sediments. This region is known from NASA's Viking 2 landers to have permafrost that has shaped the surface by repeated freezing and thawing cycles. Estimating the amount of water in the martian subsurface is of importance for human missions to Mars.
The orbiter was used to select the landing area, relay data back to Earth, and remotely map Mars. The lander was used to carry the rover and commission it on the surface, but also employs a camera with which it took the first panoramic images to identify safe paths for driving the rover. Video footage was also obtained with a remote camera on the surface. It also has a multispectral imager for mineralogical identification and distribution of the surrounding area. Zhurong also employs a sub-surface penetrating radar to investigate the 3D stratigraphic history of the area (Wang et al., Reference Wang, Feng, Zhou, Dong, Liang, Xue and Li2021), and estimate the consistency of the regolith and the depth of the permafrost. This will provide new insight into the ancient water present on Mars and climatic changes during the Red Planet's history. The magnetic field of the area will be measured with a magnetometer, while a LIBS instrument will analyse rocks, seeking very ancient martian rocks. The magnetic field of the planet will be studied remotely from the orbiter with a second magnetometer and a particle analyser. The rover employs instruments for meteorological studies, such as a microphone returning sounds of Mars.
Mars Orbiter Mission
The ISRO successfully launched the Mars Orbiter Mission (MOM), a probe also known as Mangalyaan, aboard the Polar Satellite Launch Vehicle (PSLV)-C25 on 5 November 2013 to Mars. MOM settled into martian orbit on 24 September 2014 and has been observing Mars ever since, far exceeding its 1-year design lifetime. The primary scientific goal of MOM is to explore the morphology, topography and mineralogy of surface features, and analyse the atmosphere using the indigenously developed instruments (Arunan and Satish, Reference Arunan and Satish2015). The MOM's payload includes a Mars Colour Camera (MCC) for optical imaging (Arya et al., Reference Arya, Moorthi, Rajasekhar, Sarkar, Sur, Aravind, Gambhir, Misra, Patel and Srinivas2015), a Thermal Infrared Imaging Spectrometer (TIS) for surface temperature estimation and mapping composition and mineralogy (Singh et al., Reference Singh, Sarkar, Kumar, Saxena, Rao, Bhardwaj, Desai, Sharma, Patel and Shinde2015), a Mars Exospheric Neutral Composition Analyser (MENCA) to measure in-situ composition of the low-altitude neutral exosphere and its radial distribution (Singh et al., Reference Singh, Sarkar, Kumar, Saxena, Rao, Bhardwaj, Desai, Sharma, Patel and Shinde2015), a Lyman Alpha Photometer (LAP) to determine the deuterium-to-hydrogen abundance ratio of the upper atmosphere (Sridhar et al., Reference Sridhar, Rao, Kalyani, Bhaskar, Chandran, Mahajan, Manja, Gouda, Tayaramman and Amudha2015), and a Methane Sensor for Mars (MSM) to measure total column of methane in the atmosphere (Mathew et al., Reference Mathew, Sarkar, Srinivas, Dutta, Rohit, Seth, Kumaran, Pandya, Kumar and Sharma2015).
The large and highly elliptical orbit of the MOM (261 km Perihelion to 78 000 km Apoareion) has enabled the MCC to image the far-side of Mars' moon Deimos for the first time after more than three decades (Mathew et al., Reference Mathew, Sarkar, Srinivas, Dutta, Rohit, Seth, Kumaran, Pandya, Kumar and Sharma2015). Subsequent analysis of the temporal and on-demand stereo images of the MCC led to the study of haze variability inside Valles Marineris and estimation of the optical depth of the martian atmosphere over the northern and southern walls of the valley (Mishra et al., Reference Mishra, Chauhan, Singh, Moorthi and Sarkar2016). Observations of the MCC have also enabled local-scale dust storm tracking over the Lunae Planum region and the Valles Marineris region (Guha et al., Reference Guha, Panda and Chauhan2019).
MENCA observations have led to significant discoveries including in-situ compositional measurements for three major constituents of the martian exosphere, i.e. amu 44 (CO2), amu 28 (N2 + CO), and amu 16 (O) corresponding to the local evening hours (Bhardwaj et al., Reference Bhardwaj, Mohankumar, Das, Pradeepkumar, Sreelatha, Sundar, Nandi, Vajja, Dhanya and Naik2015). These measurements are considered critical for setting up the important boundary conditions in the thermal escape models (Bhardwaj et al., Reference Bhardwaj, Thampi, Das, Dhanya, Naik, Vajja, Pradeepkumar, Sreelatha, Thampi and Yadav2017). Furthermore, the presence of suprathermal argon atoms (argon-40) has been reported in the martian exosphere using scale height and temperature measurements (Bhardwaj et al., Reference Bhardwaj, Mohankumar, Das, Pradeepkumar, Sreelatha, Sundar, Nandi, Vajja, Dhanya and Naik2015). To establish the boundary conditions for global circulation model calculations, the reflected solar radiance measured by the MSM in two Shortwave Infrared (SWIR) (1.64–1.66 μm) channels has been used to prepare an albedo map of the martian surface (Singh et al., Reference Singh, Mishra and Chauhan2017).
After 5 years, MCC continues to capture intriguing views of Mars contributing significantly to the exploration of its surface. There is much more to be leveraged from the MOM datasets that can be fundamental in developing new insight for the surface and atmospheric evolution of Mars. The identification of scientific knowledge gaps and the optimization of suitable payloads are the key to success of the ISRO's continued planetary exploration programme.
Exomars 2022
The Rosalind Franklin rover of the ExoMars 2022 mission (Fig. 8) will land in Oxia Planum (Quantin-Nataf et al., Reference Quantin-Nataf, Carter, Mandon, Thollot, Balme, Volat, Pan, Loizeau, Millot and Breton2021), an ancient location with strong potential for past habitability and preserving physical and chemical biosignatures (as well as abiotic/prebiotic organics). Oxia Planum is situated on the eastern margin of the Chryse basin, along the martian dichotomy border, and at the outlet of the Coogoon Valles system. At present, the coordinates for the nominal touchdown location are 18.159°N, 24.334°W. The approximately 90 × 4 km dispersion ellipse lies in the lower part of a wide basin, where extensive exposures of Fe/Mg-phyllosilicates (>80% of the ellipse surface area) have been detected with OMEGA and CRISM hyperspectral and multispectral data. The Fe/Mg-rich clay detections are associated with early/middle- to late-Noachian layered rocks (with layering thickness ranging from a few metres to <1 m for several tens of metres) (Mandon et al., Reference Mandon, Parkes Bowen, Quantin-Nataf, Bridges, Carter, Pan, Beck, Dehouck, Volat and Thomas2021). They may represent the southwestern expansion (lowest member) of the Mawrth Vallis clay-rich deposits, pointing to a geographically extended aqueous alteration environment, and perhaps an ocean.
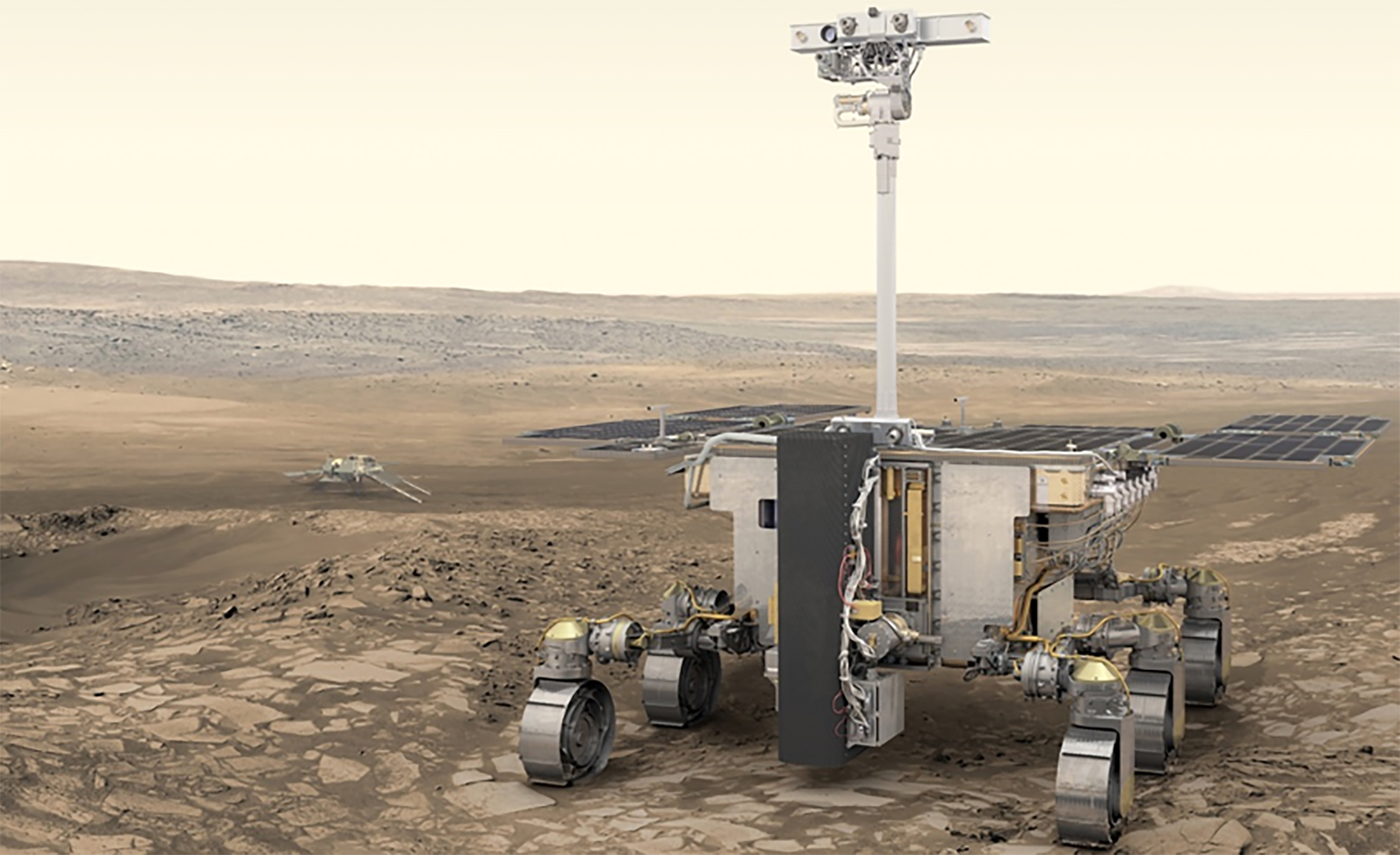
Fig. 8. Artist rendition of the Rosalyn Franklin ExoMars Rover.
Optimizing life detection with ExoMars
The payload of the ExoMars rover has been put together based on the assumption that it is highly unlikely that lifeforms would exist on the surface of Mars today. Therefore, the focus was placed on the identification of traces of past life, from the time when the surface environment was able to host abundant liquid water, i.e. the Noachian (Vago et al., Reference Vago, Westall, Coates, Jaumann, Korablev, Ciarletti, Mitrofanov, Josset, De Sanctis and Bibring2017). With this in mind, ESA decided to concentrate on the detection of physical (textural) and chemical biosignatures. Other possible, but less definitive classes of biosignatures, like isotopic analysis and the investigation of possible metabolites, will not be investigated with the current payload. To maximize the chance of having access to well-preserved biomolecules, the rover is equipped with a drill having a 2-m depth reach. This drill can be used to collect samples from top- and subsurface sedimentary rocks, which are then analysed for mineralogy and organic composition using instruments in the rover's analytical laboratory. The rover exploration strategy is based on a stepwise execution of nested-scale investigations, proceeding from the panoramic visual scale, to the microscopic, and concluding with molecular analyses. The rover's Pasteur payload includes panoramic instruments PanCam (Coates et al., Reference Coates, Jaumann, Griffiths, Leff, Schmitz, Josset, Paar, Gunn, Hauber and Cousins2017), wide-angle and high-resolution cameras; ISEM (Korablev et al., Reference Korablev, Dobrolensky, Evdokimova, Fedorova, Kuzmin, Mantsevich, Cloutis, Carter, Poulet and Flahaut2017), an infrared spectrometer; WISDOM (Ciarletti et al., Reference Ciarletti, Clifford, Plettemeier, Le Gall, Hervé, Dorizon, Quantin-Nataf, Benedix, Schwenzer and Pettinelli2017), a ground-penetrating radar; and ADRON (Litvak et al., Reference Litvak, Mitrofanov, Barmakov, Behar, Bitulev, Bobrovnitsky, Bogolubov, Boynton, Bragin and Churin2008), a neutron detector; a subsurface drill to acquire samples; contact instruments for studying rocks and collected material (CLUPI (Josset et al., Reference Josset, Westall, Hofmann, Spray, Cockell, Kempe, Griffiths, De Sanctis, Colangeli and Koschny2017), a close-up imager; and MaMISS (De Sanctis et al., Reference De Sanctis, Altieri, Ammannito, Biondi, De Angelis, Meini, Mondello, Novi, Paolinetti and Soldani2017), an infrared spectrometer in the drill head); a Sample Preparation and Distribution System (SPDS); and the analytical laboratory, the latter including MicrOmega (Bibring et al., Reference Bibring, Hamm, Pilorget and Vago2017), a visual and infrared imaging spectrometer; RLS (Rull et al., Reference Rull, Maurice, Hutchinson, Moral, Perez, Diaz, Colombo, Belenguer, Lopez-Reyes and Sansano2017), a Raman spectrometer; and MOMA (Goesmann et al., Reference Goesmann, Brinckerhoff, Raulin, Goetz, Danell, Getty, Siljeström, Mißbach, Steininger and Arevalo2017), a Laser-Desorption, Thermal-Volatilization, Derivatization, Gas Chromatograph Mass Spectrometer (LD + Der-TV GCMS).
Martian meteorites and sample return
A few decades ago, it was realized that among meteorites on Earth, about a dozen had textures, young ages, oxygen isotopic ratios, and noble gas compositions consistent with a martian origin. Today there are ~140 martian meteorites. In particular, noble gas abundances trapped in shocked glass from martian meteorites are in a ~1:1 ratio with Mars' atmospheric composition.
As well as being trapped from Mars, noble gases can also form in martian meteorites in situ. They can form from spallation, radioactive decay and fission reactions called cosmogenic, radiogenic and fissiogenic, respectively. Cosmogenic noble gas components, for instance, help us to trace the ejection time of rocks from the martian surface. Radiogenic noble gas nuclides, such as 40Ar produced through the decay of 40K, enable formation ages to be determined. 129Xe from the decay of 129I, an extinct radionuclide with a half-life of 15.7 Myr, can unravel atmospheric evolution. Fissiogenic heavy Xe isotopes (e.g. 134Xe and 136Xe) in martian meteorites record fission reactions of 238U and 244Pu from the martian atmosphere or interior (Smith et al., Reference Smith, Ranjith, He and Zhu2020b).
Cosmic ray exposure (CRE) age is the time a meteoroid spent in interplanetary space from ejection to arrival to Earth. Cosmogenic noble gases (3He, 21Ne,38Ar, 83Kr, 124Xe) produced in the meteoritic material by cosmic ray spallation reactions in specific target elements are measured to determine CRE ages. The ejection time is the sum of CRE age in space and the terrestrial residence time on earth. Terrestrial ages are determined by measuring radionuclides such as 14C, 10Be, 36Cl and 41Ca. As the terrestrial residency times are negligible compared to the CRE ages, CRE ages directly indicate ejection times. Source-crater pairings of different martian meteorites are established on the basis of CRE age groupings and their petrologic affinities (Gladman, Reference Gladman1997). The martian meteorites group into 9–10 different CRE ages. Thus, there were around 9–10 different ejection events on Mars, which produced the current suite of martian meteorites that arrived on Earth.
Source ejecta craters for the different SNC martian meteorites
Martian meteorites are impact ejecta by the bombardment of fragments of asteroids or comets onto the surface of Mars, and the three main types of martian meteorites are shergottites, nakhlites, and chassignites (SNC). If one can identify the source impact crater of martian meteorites,we could more accurately describe its geological evolution, and more effectively plan for future missions.
Finding the source craters for our more than 140 martian meteorites is however very challenging. When we classify a martian meteorite on the basis of its mineral abundances and compositions, or trace element abundances, we are at a level of detail that is very hard to replicate from remote spectroscopy. It may happen for some meteorite samples, but we will learn about Mars from the meteorites as fragments of the crust, but not from known exact locations. Even if we are not sure exactly which craters they were derived from, they still give essential pieces of information about the planet's differentiation and the action of water in the crust.
Noble gas composition of the martian atmosphere
Signatures of the ‘ancient martian atmosphere’ (based on, e.g. the martian meteorite Alan Hills (ALH) 84001) and ‘modern martian atmosphere’ (from, e.g. Northwest Africa (NWA) 7034) can be traced by noble gases. Trapped noble gases (Ar and Xe) in martian meteorites such as shock-produced glasses are in good agreement with MSL measurements on Mars (40Ar/36Ar = 1900 ± 300, 36Ar/38Ar = 4.2 ± 0.1, 129Xe/132Xe = 2.5221 ± 0.0063) (Avice et al., Reference Avice, Bekaert, Aoudjehane and Marty2018). Based on meteorite studies, 40Ar/36Ar on Mars is much higher and 36Ar/38Ar is lower than Earth's atmosphere, indicating enrichment in radiogenic 40Ar compared to 36Ar, possibly due to solar wind-induced sputtering (Atreya et al., Reference Atreya, Trainer, Franz, Wong, Manning, Malespin, Mahaffy, Conrad, Brunner and Leshin2013). The 129Xe/132Xe ratio in martian meteorites and on Mars is elevated due to radiogenic 129Xe. This requires either very early loss from the atmosphere or storage in some reservoir until after atmospheric loss (Swindle, Reference Swindle2002).
Noble gases from the martian surface and interior
Mudstone on the floor of Gale Crater analysed by MSL determined K-Ar age of 4.21 ± 0.35 billion years, representing a mixture of detrital and authigenic components in ancient rocks making up the crater rim (Farley et al., Reference Farley, Malespin, Mahaffy, Grotzinger, Vasconcelos, Milliken, Malin, Edgett, Pavlov and Hurowitz2014). Furthermore, the surface exposure age determined by the cosmogenic isotopes 3He, 21Ne and 36Ar is 78 ± 30 million years, indicating surface exposure rather than primary erosion and transport. This absolute age data enables calibration of relative surface ages for crater chronology.
In addition to the atmospheric components, martian meteorites can also contain trapped martian interior components, which are more challenging to measure with the current Mars landers and rovers. Information we have on martian interior gases are primarily mostly from the meteorite Chassigny. Fluid inclusions in olivine in Chassigny have higher Kr and Xe content, and minimal radiogenic 129Xe, unlike the martian atmosphere (Swindle, Reference Swindle2002). Chassigny has also a much lower Kr/Xe than Mars' current atmosphere and a Xe isotopic composition close to that of the solar wind, suggesting an undegassed interior martian reservoir (Swindle, Reference Swindle2002). In addition to solar Xe, another interior component has been suggested due to the addition of 244Pu fission component from the martian interior (Mathew and Marti, Reference Mathew and Marti2001). In summary, noble gases can be highly diagnostic when unravelling planetary formation and evolution.
The martian surface and interior revealed by martian meteorites
The martian breccias, NWA 7034 and its many pairs, contain the most evolved martian igneous material and a high amount of volatiles including water. They are also the eldest martian samples yet identified. Water released by step-heating of the martian breccia showed concentrations of ~3000 ppm, an order of magnitude above that of any other martian meteorite (Agee et al., Reference Agee, Wilson, McCubbin, Ziegler, Polyak, Sharp, Asmerom, Nunn, Shaheen and Thiemens2013). Its composition is also enriched in alkalis and other volatiles relative to the SNC meteorites. The dating of zircons included within the breccia show ages around 4.4 and 1.7 Ga, suggesting an ancient initial crystallization age followed by a later major disturbance (Humayun et al., Reference Humayun, Nemchin, Zanda, Hewins, Grange, Kennedy, Lorand, Göpel, Fieni and Pont2013; Bouvier et al., Reference Bouvier, Costa, Connelly, Jensen, Wielandt, Storey, Nemchin, Whitehouse, Snape and Bellucci2018). The most-precise analyses of zircons and geochemical modelling predict the existence of an evolved andesitic crust on Mars with a minimum age of 4547 Ma (Bouvier et al., Reference Bouvier, Costa, Connelly, Jensen, Wielandt, Storey, Nemchin, Whitehouse, Snape and Bellucci2018). This would have had to predate the currently basaltic crust on Mars and was likely later destroyed by impacts and resurfacing.
The shergottites
The shergottites make up the highest fraction of martian meteorites. In the current collection of martian meteorites, ~80% are shergottites, including basaltic, olivine-phyric and lherzolitic shergottites (martian Meteorite Compendium) (Wang and Hu Reference Wang and Hu2020), as well as the recently identified augite-rich shergottites, NWA 7635 (Lapen et al., Reference Lapen, Righter, Andreasen, Irving, Satkoski, Beard, Nishiizumi, Jull and Caffee2017) and NWA 8159 (Herd et al., Reference Herd, Walton, Agee, Muttik, Ziegler, Shearer, Bell, Santos, Burger, Simon, Tappa, McCubbin, Gattacceca, Lagroix, Sanborn, Yin, Cassata, Borg, Lindvall, Kruijer, Brennecka, Kleine, Nishiizumi and Caffee2017). Most of the shergottites have crystallization ages ranging from ~600 to ~ 150 Ma by the analyses of mineral isochrons of Rb-Sr and Sm-Nd (Nyquist et al., Reference Nyquist, Bogard, Shih, Greshake, Stoffler and Eugster2001), and in-situ U-Pb dating of baddeleyite and phosphates (Niihara, Reference Niihara2011; Moser et al., Reference Moser, Chamberlain, Tait, Schmitt, Darling, Barker and Hyde2013; Zhou et al., Reference Zhou, Herd, Yin, Li, Wu, Li, Liu, Tang and McCoy2013). Two new augite-rich shergottites were however dated at ~2.4 Ga (Herd et al., Reference Herd, Walton, Agee, Muttik, Ziegler, Shearer, Bell, Santos, Burger, Simon, Tappa, McCubbin, Gattacceca, Lagroix, Sanborn, Yin, Cassata, Borg, Lindvall, Kruijer, Brennecka, Kleine, Nishiizumi and Caffee2017; Lapen et al., Reference Lapen, Righter, Andreasen, Irving, Satkoski, Beard, Nishiizumi, Jull and Caffee2017). The relatively young ages of most shergottites (Váci and Agee, Reference Váci and Agee2020) indicate that they were ejected from the Amazonian Epoch of Mars. On the other hand, the ejection ages of shergottites vary from ~1 to ~5 Ma except for the shergottite, Dhofar 019 at ~20 Ma (Fritz et al., Reference Fritz, Artemieva and Greshake2005). These variations suggest that there may have been several impact events on Mars that eventually delivered material to Earth.
Some work has been carried out attempting to constrain the source craters of the shergottites (Mouginismark et al., Reference Mouginismark, McCoy, Taylor and Keil1992; Treiman, Reference Treiman1995; McFadden and Cline, Reference McFadden and Cline2005; Tornabene et al., Reference Tornabene, Moersch, McSween, McEwen, Piatek, Milam and Christensen2006) and other martian meteorites (Kereszturi and Chatzitheodoridis, Reference Kereszturi and Chatzitheodoridis2016). Some candidate craters have been proposed – examples being the Mojave Crater, forming <5 Ma ago, with a width of ~55 km, on the 4.3 Ga old terrain of Mars. It was proposed as the ejection source for the shergottites due to its very similar reflectance spectra to the shergottite Queen Alexandria (QUE) 94201 and Shergotty in the wavelength range of 1–2.4 cm (Werner et al., Reference Werner, Ody and Poulet2014). However, the size of Mojave Crater (Fig. 9) is one order of magnitude higher than that simulated by the launch dynamics (Head et al., Reference Head, Melosh and Ivanov2002). The very young age of Mojave Crater (Fig. 7) also requires better constraints because of the low accuracy of crater chronology for the young craters without ground-based calibration (Hartmann and Neukum, Reference Hartmann and Neukum2001). Moreover, the host terrain under the Mojave Crater is substantially older than most of the young shergottites. Future martian sample return missions will contribute greatly in clarifying this issue (Beaty et al., Reference Beaty, Grady, McSween, Sefton-Nash, Carrier, Altieri, Amelin, Ammannito, Anand, Benning, Bishop, Borg, Boucher, Brucato, Busemann, Campbell, Czaja, Debaille, Des Marais, Dixon, Ehlmann, Farmer, Fernandez-Remolar, Filiberto, Fogarty, Glavin, Goreva, Hallis, Harrington, Hausrath, Herd, Horgan, Humayun, Kleine, Kleinhenz, Mackelprang, Mangold, Mayhew, McCoy, McCubbin, McLennan, Moser, Moynier, Mustard, Niles, Ori, Raulin, Rettberg, Rucker, Schmitz, Schwenzer, Sephton, Shaheen, Sharp, Shuster, Siljeström, Smith, Spry, Steele, Swindle, ten Kate, Tosca, Usui, Van Kranendonk, Wadhwa, Weiss, Werner, Westall, Wheeler, Zipfel and Zorzano2019).

Fig. 9. Mojave crater (58 km across) on the Xanthe Terra plain of Mars. NASA/Caltech/Arizona State University..
Alteration in the martian meteorites
Alteration minerals and textures have been identified in martian meteorites, namely in the nakhlites. The nakhlites are clinopyroxenites (Treiman, Reference Treiman2005). Alteration mostly occurs along olivine fractures and in mesostases. A martian origin of alteration in the other types of martian meteorites are controversial, although carbonate globules in ALH 84001 are generally accepted as extraterrestrial (Treiman, Reference Treiman2021). Carbonate was first suggested to occur in a martian meteorite by (Carr et al., Reference Carr, Grady, Wright and Pillinger1985) using C isotopic measurements during stepped combustion of Elephant Moraine (EETA) 79001 martian meteorite. Carbonate was later discovered in Nakhla as Mn-rich siderite (Chatzitheodoridis and Turner, Reference Chatzitheodoridis and Turner1990) in an assemblage with Ca-sulphate and halite. Additional evidence came from Gooding et al. (Reference Gooding, Wentworth and Zolensky1991). Since then, the discoveries of alteration phases have been rapidly increasing in number. The list of secondary minerals includes siderite (FeCO3), anhydrite (CaSO4), NaCl, ferric saponite clay (Ca0.25(Mg,Fe)3((Si,Al)4O10)(OH)2 · nH2O), hematite (Fe2O3), oxyhydroxides (ferrihydrite Fe3+10O14(OH)2), serpentine ((Mg,Fe)3Si2O5(OH)4), and opal (SiO2· nH2O). Their discoveries are important for understanding martian weathering processes which have implications for interpreting the paleoenvironment of Mars. Moreover, they may hint at potential ecological niches in the subsurface of Mars, such as vesicles formed in mesostasis formed by bolide impact processes (Chatzitheodoridis et al., Reference Chatzitheodoridis, Haigh and Lyon2014) or for instance networks of microcavities of magmatic clays serving as microreactors for prebiotic chemistry (Viennet et al., Reference Viennet, Bernard, Guillou, Sautter, Grégoire, Jambon, Pont, Beyssac, Zanda and Hewins2021). Similar types of alteration on Mars provide direct comparisons between Earth-based samples and Mars prior to the eventual return of samples from the planet.
Martian Moon eXploration sample return
The Japanese Aerospace Exploration Agency has a Mars moon sample return mission planned for launch in 2024 to Phobos and Deimos; the Martian Moon eXploration Mission (MMX). The orbits of these satellites suggest that they formed either from large impact ejecta from Mars that then accumulated, or at the same time as proto-Mars itself (Bagheri et al., Reference Bagheri, Khan, Efroimsky, Kruglyakov and Giardini2021), although they share morphological and spectral characteristics with asteroids (See Early Mars section). Subsequent to their formation, the martian moons accreted materials transported from Mars, probably delivered from cataclysmic impact events on the planet.
Phobos and Deimos may have therefore been contaminated by microorganisms if there was life in the martian subsurface. The mission will collect samples from Phobos, with successive flybys of Deimos. If putative martian life is transferred to Phobos or Deimos, what unique challenges will arise for a sample return mission? According to previous assessments of microbial contamination risk for samples collected on the martian moons (Fujita et al., Reference Fujita, Kurosawa, Genda, Hyodo, Matsuyama, Yamagishi, Mikouchi and Niihara2019; Kurosawa et al., Reference Kurosawa, Genda, Hyodo, Yamagishi, Mikouchi, Niihara, Matsuyama and Fujita2019), the probability that a single unsterilized particle occurring within samples can be maintained within the internationally agreed acceptable limit of 10−6, provided that the mass of samples is below 100 g, and that they are taken within a depth of several tens of centimetres from the surface. The extant microbial density on the martian moons (if in fact Mars was inhabited) is subject to the rates at which microbes are inactivated by high-speed impacts of martian ejecta on the martian moons and by cosmic radiation after accretion onto the surface. The above assessment has been conducted based on laboratory simulation experiments. However, there still remains uncertainty with these results. For this reason, it is strongly recommended by the Committee on Space Research (COSPAR) that a re-evaluation is made before the return of martian moon samples to Earth. For this purpose, we have started new follow-up experiments to constrain these uncertainties.
Sampling asteroids versus Phobos
The Japanese Aerospace Exploration Agency also returned the samples from asteroid 162173 Ryugu in December 2020 with the Hayabusa-2 spacecraft, the first successful sample return mission to a carbonaceous asteroid. There are several challenges with a sample return mission from the martian moons that differ to the sample return from asteroids. From a technical viewpoint, it is a great challenge implementing a sampling method that complies with the above criteria in the absence of detailed information on the state of the regolith on the martian moons. Although gravity on the martian moons is much smaller than that of Mars or Earth's moon, it is several orders of magnitude larger than that of asteroids (Yang et al., Reference Yang, Yan, Andert, Ye, Pätzold, Hahn, Jin, Li and Barriot2019). This requires a more-tailored propulsion system directed towards the martian moons during touchdown and lift-off. The exhausts from the propulsion systems may disturb regolith on the martian moons, and potentially accrete regolith onto the spacecraft by electrostatic attraction. Regolith scattering can also occur by touchdown of the landing gears and sampling. Adhesion of regolith to the spacecraft will substantially affect the bulk amount of the martian moons samples brought back to Earth, potentially resulting in a higher probability of microbial contamination. In order to avoid such a risk, it is not only necessary to suppress the regolith scattering by optimizing the layout and operation of the propulsion system, by minimizing the touchdown velocity, and by devising the sampling mechanism so that it can quietly collect samples (without creating turbulence), but also to identify the area of regolith adhering to the spacecraft. The spacecraft system and its operation plan for the MMX mission are currently designed according to the above policies with the aid of the analysis on the regolith touchdown, sampling and lift-off dynamics.
Mars 2020 sample caching
Perseverance successfully collected and cached its first rock sample from the martian surface on 1 September 2021. This was the first sample collected for return from the surface of another planet. There are 43 sample containers in a carousel for sample caching in titanium hermetically sealed tubes. Five unsampled witness tubes are included to identify any possible cross-contamination from Earth by the sample caching process. Samples will be deposited locally on the martian surface of the planet to be picked up by a fetch rover or perhaps even delivered by Perseverance in a follow-up mission towards the end of the decade.
The first sample collected by Perseverance belongs to a rock named ‘Rochette’. The sample caching system contains a drill used for abrasion of the surface of rocks to reveal their subsurface, followed by actual core drilling and sampling. The acquired sample is a drill tube of rock that weighs 15 g (core diameter of 1 cm and length of 5 cm). Primary to the selection of samples, analytical information can be acquired with the instruments such as SHERLOC and PIXL. The selection of the samples will be based entirely on the remote detection of areas of interest; once an interesting sample is located, drilling and cashing operations will be performed.
Mars human exploration
What are the essential requirements for humans to live and thrive on Mars?
Eventually, sooner or later, human missions to Mars will occur. If they are to constitute footholds rather fleeting jaunts, martian resources must be utilized to support humans in a lifestyle that is both sustainable and, just as importantly, comfortable. It is clear that sustainable settlement in a martian environment must be systematic and as complete as possible to maximize the use of in-situ resources, and minimize reliance on Earth through a long, expensive, and slow-reacting supply chain. Resource technologies designed to enable the autonomy of humans on Mars (so that they do not rely on Earth), as well as to characterise the martian environment, are under development (atmospheric composition, hydrology, mineralogy, and geological history). A comprehensive review of martian in-situ resource utilization is given in Ellery and Muscatello (Reference Ellery and Muscatello2017) and details on sustainable in-situ resource utilization in Ellery (Reference Ellery2020). Agriculture, for the generation of food, and the recycling of nutrients to substitute for biogeochemical cycles on Earth is essential – of course, martian soil may be utilized or hydroponics and aeroponics provide the means to render agriculture independent of soil – but in all cases, nutrient recycling will be required.
Key technological challenges arising from human space travel to the exploration of Mars include (i) survival during our trip towards Mars in the frame of investigations on the stability and level of degradation of space-exposed biosignatures such as pigments, secondary metabolites and cell surfaces in contact with a terrestrial and Mars analogue mineral environment; (ii) space radiation, because it has been demonstrated that the particle radiation environment on Mars can vary according to the epoch concerned and the landing site selected; (iii) human-safe landing and return systems; (iv) life-support and learning to live sustainably on Mars (in synergy with technologies developed for extreme environments on the Earth and the Moon). However, we still have to address a number of important questions such as what are the limits to interplanetary human travel to Mars, which technologies have still to be developed, and what studies and human simulations should be performed? Substantial research and development is required to provide the basic information for appropriate integrated risk management, including efficient countermeasures and tailored life support. Methodological approaches could include research on the International Space Station (ISS), on robotic precursor missions to Mars, in ground-based simulation facilities, as well as in analogue natural environments on Earth (Foing et al., Reference Foing, Stoker and Ehrenfreund2011a, Reference Foing, Stoker, Zavaleta, Ehrenfreund, Thiel, Sarrazin, Blake, Page, Pletser and Hendrikse2011b).
Resource utilization on Mars
One key resource is the carbon dioxide atmosphere as it has long been proposed that carbon dioxide can be converted into methane using hydrogen feedstock via the Sabatier reaction (Zubrin et al., Reference Zubrin, Muscatello and Berggren2013). Methane may be used as a propellant, which combined with an oxidizer, can provide the basis for refuelling Mars Ascent Vehicles and rover sorties during in-situ exploration activities. Water ice on Mars can provide another resource for life support, hydrogen feedstock, and oxygen for respiration. Acquiring water ice, however, will require extraction from the martian subsurface through drilling/mining techniques. Finally, raw regolith may be exploited by combining with binders (such as plastic or salts) for the construction of habitation shells using 3D printing (Cesaretti et al., Reference Cesaretti, Dini, De Kestelier, Colla and Pambaguian2014). There is much we can leverage from Mars in constructing the internal environment for a martian habitat (Ellery, Reference Ellery2020).
Technologies for resource utilization
To make Mars our home, an industrial capacity from martian materials should be built. Machines will provide the means of production to construct this industrial capacity. We need to build excavator rovers, comminution and beneficiation machines, unit chemical processors, milling machines, 3D printers, assembly manipulators, and other machines that convert raw material into useful products. These machines are all kinematic machines – the fundamental components of the kinematic machine are the electric motor, its control system and its power supply (Ellery, Reference Ellery2016). These technologies will require a basic set of materials including iron (from hematite), nickel, cobalt, tungsten and selenium (from nickel-iron meteorites), silica and silicon (from silicate minerals), plastics (from a mixture of H2 and CO) and acid reagents (from salts). The core unit chemical processor is the Metalysis FFC (Fray-Farthing-Chen) process supported by a range of acid-based and carbonyl-based peripheral processes (Ellery, Reference Ellery2017). Combined with 3D printing technology (Ellery and Muscatello, Reference Ellery and Muscatello2017), this provides a powerful suite of techniques for universal construction capabilities including the construction of electric motors and computational electronics (Ellery, Reference Ellery2016), the latter based on neural network architectures (Prasad and Ellery, Reference Prasad and Ellery2020). Human exploration can leverage these same components to support energy generation through solar concentrator-thermionic conversion and energy storage through motorized flywheels. We can thus construct an industrial infrastructure of machines as the fundamental requirement for self-sufficient habitability.
Bio-materials for in-situ resource utilization
Synthetic biology and applied microbiology can be used to produce resources in space (Rothschild, Reference Rothschild2016). For example, carbonate biomineral-producing microbes are used on Earth in civil engineering, art restoration, soil improvement, bioremediation, and CO2 sequestration (Reddy, Reference Reddy2013; Anbu et al., Reference Anbu, Kang, Shin and So2016), as well as space. Calcite production is common among microorganisms and can be linked with different types of taxa and metabolisms (Boquet et al., Reference Boquet, Boronat and Ramos-Cormenzana1973). Bacteria, such as Streptomyces species, promote the precipitation of calcium carbonate by activating specific metabolic pathways. For biotechnological applications, the genera Bacillus and Sporosarcina are the most studied (Han et al., Reference Han, Wang, Zhao, Tucker, Zhao, Wu, Zhou, Yin, Zhang and Zhang2019), but knowledge gaps remain about the microbes best-suited for each type of application, and how environmental conditions affect the characteristics and yields of biominerals. Terrestrial bacteria found in calcium-rich natural environments on Earth could be used on the Moon or Mars for the production of biobricks for constructing buildings (Santomartino et al., Reference Santomartino, Waajen, De Wit, Nicholson, Parmitano, Loudon, Moeller, Rettberg, Fuchs and Van Houdt2020). Some strains that precipitate calcium carbonate or gypsum (depending on the substrate provided; Cirigliano et al., Reference Cirigliano, Tomassetti, Di Pietro, Mura, Maneschi, Gentili, Cardazzo, Arrighi, Mazzoni and Negri2018) are also able to produce melanin that protects against UV and ionizing radiation.
Biomineralization processes have been proposed as a means of cutting the construction costs on the Moon and Mars (Cockell, Reference Cockell2010; Dikshit et al., Reference Dikshit, Dey, Gupta, Varma, Venugopal, Viswanathan and Kumar2020; Kumar et al., Reference Kumar, Dikshit, Gupta, Jain, Dey, Nandi, Venugopal, Viswanathan, Sridhara and Rajendra2020). More work is needed on the feasibility of the use of Mars dust and regolith for such a proposed in-situ resource utilization, e.g. testing this process under Mars-like conditions. One limitation may be the various salts that are known to inhibit or prevent microbial metabolism, including perchlorates and chaotropic salts, sulphate salts, and those that create multiple stresses and polyextreme conditions (Fox-Powell et al., Reference Fox-Powell, Hallsworth, Cousins and Cockell2016; Hallsworth, Reference Hallsworth2019; Benison et al., Reference Benison, O'Neill, Blain and Hallsworth2021). Work is therefore needed to identify strains tolerant to salt-induced stresses and optimize culture conditions to mitigate against the underlying cellular stress mechanisms.
Human landing site on Mars
At the current time, there is a major community-wide discussion taking place regarding the required/desired attributes of the future human landing site, and possible locations on Mars that have those attributes. At the present stage of the discussion, there are multiple sites actively under consideration. Some key factors include elevation (which has to be below a certain level), minimize landing site hazards such as slopes and large rocks, attributes related to the purpose of mission (such as science), access to water ice, and planetary protection. Some candidate regions for SpaceX's Starship are Phlegra Montes, Erbeus Montes and Arcadia Planitia (Golombek et al., Reference Golombek, Williams, Wooster, McEwen, Putzig, Bramson, Head, Heldmann, Marinova and Beaty2021).
Terrestrial simulations of human missions to Mars
There is a number of ground-based simulation facilities in analogue natural environments on Earth. Building on the EuroGeoMars 2009 campaign (Foing et al., Reference Foing, Stoker and Ehrenfreund2011a, Reference Foing, Stoker, Zavaleta, Ehrenfreund, Thiel, Sarrazin, Blake, Page, Pletser and Hendrikse2011b), the ILEWG EuroMoonMars programme since 2009 has included research activities for data analysis, instrument tests and development, field tests in the MoonMars analogue, pilot projects, training and hands-on workshops, and outreach activities. Field tests have been conducted in ESTEC (European Space Research and Technology Centre) and EAC (the European Astronaut Centre) of ESA, as well as at the Utah's MDRS station (Mars Dessert Research Station), Eifel, Rio Tinto (Spain), Iceland, La Reunion (an island in the Indian Ocean that belongs to the French Republic), LunAres base at Pila Poland and the HISEAS base in Hawaii.
Simulating human experience on Mars using virtual reality
Humans can experience Mars using virtual reality. Current virtual reality engines can elevate the quality and immersion of Mars with the high-fidelity mechanics, interactions and continually evolving design principles.
By using Mars topological and in-situ data from current orbiters and rovers, respectively, extrapolation of a 3D virtual reality martian landscape can be made. All touch points within the experience (locations, mineral outcrops, weather, caves, tools, etc) can be scientifically accurate on a 1:1 scale, and provide transferable knowledge beyond the virtual reality experience (Fig. 10).
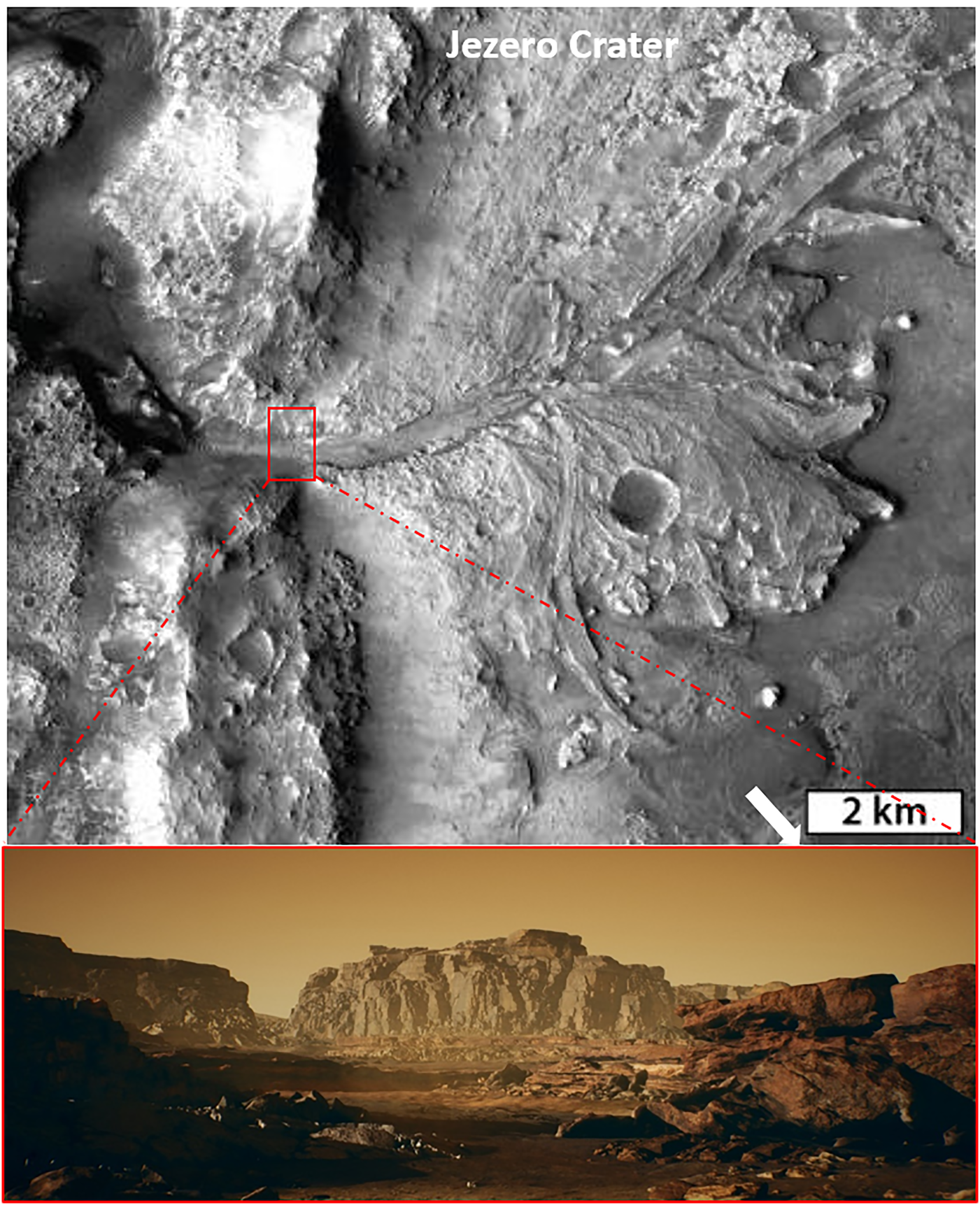
Fig. 10. In-engine virtual reality (VR) terrain of the Jezero Crater looking into the river delta (bottom) extrapolated from Mars Reconnaissance Orbiter imagery (top). Top image courtesy of NASA/JPL/JHUAPL/MSSS/Brown University. With imagery from the Perseverance rover, the VR engine can be refined for more accuracy. Arrowed is the current approximate location of Perseverance.
In virtual reality, humans can navigate the hazards of the martian surface, and use simulated rover playloads, spacesuits and handheld scientific instruments to conduct experiments on the surface of Mars and discover the viability of interplanetary human expansion.
Mars human settlement is ‘ante portas’: are we ready for it?
Human settlement on Mars is the next coming human milestone. However, societal risks emerge on a global scale (Shaghaghi and Antonakopoulos, Reference Shaghaghi and Antonakopoulos2012). Quantitatively estimating how to cope with those radical societal changes primarily invents a way of measuring and monitoring the societal readiness towards Mars human presence, from Earth's and Mars' points of view, and then identifying and remediating the risks that will arise for humanity. Such a tool could be quantitatively expressed with a risk index, such as the Mars Risk Index (MRI), a data-driven index estimated under the MRI Framework which monitors the societal readiness and identifies the risks that arise for humanity. It assists all stakeholders to orientate themselves as Mars human settlement takes shape. It is envisaged that two main sub-indices will allow the evaluation of the MRI:
(a) The Mars Earth Risk Index (μRI:e) that measures Earth's societal readiness shifting towards the Mars human settlement.
(b) The Mars Ares Risk Index (μRI:a) that measures Mars' future societies structures readiness to cope with Earth's existing societal structures.
To explain briefly the MRI's methodology (Fowler et al., Reference Fowler, Reston, Carter, Quinn and Davies2004), the analysis of societal and political risks will take firstly place, based on the relationship between frequency and the number of people suffering from a specified level of harm in a given population from the realization of specified threats. The quantitative risk assessment approach is based on the estimations of the pair of frequency (f) and potential consequences, and the typical number of fatalities (N), resulting in a cumulative frequency (F) of events that has N or more fatalities, visually compared through sets of curves, commonly referred to as F-N curves. With the use of such curves, judgements will be made. To further establish this framework, possible threats, the available assets and the estimated impacts must be taken into account.
Minimizing human risks for settlements on Mars
Human settlement of Mars will be a consorted international endeavour. Its stakeholders will span all societal pillars such as, nations, countries and their governments, international organizations, religious groups, and of course, people (i.e. individuals).
As a consequence of the societal risks that emerge on the global scale, unstable environments initiating domino effects might be created that will shake our societies' structures. It is our responsibility to adapt our societies to this endeavour, which is by far larger than, i.e. the discovery of the New World. MRI can help all stakeholders orientate themselves as Mars human settlement and colonization takes shape, alert them for changes that could mitigate those risks and confront the challenges.
Planetary protection
Mars has been categorized as a planetary protection restricted class Category V, relating to the return of space craft or space components. This means that where scientific opinion is unsure, destructive impact upon return to Earth is prohibited. Containment of all returned hardware which directly contacted the target body, and any unsterilized sample returned to Earth, is also required. The same is also required for the forward contamination of Mars itself.
If either extinct or extant life is discovered on Mars, should the UN resolutions on planetary protection be amended?
If any indications of Mars life are found in early assessments, one has to assume that life continues to exist on Mars until more data are collected.
Currently, the international policy on planetary protection as maintained by COSPAR states explicitly that any decisions about novel mission activities should be based on scientific advice from the COSPAR Member National Scientific Institutions and International Scientific Unions. This includes a reassessment on the status of Mars if evidence for indigenous life is discovered. The current COSPAR guidelines for analysis of samples returned from Mars focus on the potential for false-positive results, because a false positive might result in unnecessarily increased restrictions on Mars exploration.
However, any evidence for past Mars life would significantly increase concerns about the potential for false-negative detections of extant Mars life. If samples from Mars provide evidence of indigenous life in the distant past, it is not valid to assume all Mars life subsequently became extinct. Due to the potential for hazardous interactions between Mars life and Earth life, the detection of past life on Mars would appropriately lead to increased restrictions on the exploration of Mars and return of Mars' material to Earth. The 2020 pandemic of COVID-19 illustrates some potential consequences resulting from false-negative detections of hazardous organisms. Planetary protection was established and needs to continue in effective implementation, to prevent space exploration causing this sort of pandemic scenario. A thorough assessment of various modes of inactivation and containment of martian sampling is ongoing (Craven et al., Reference Craven, Winters, Smith, Lalime, Mancinelli, Shirey, Schubert, Schuerger, Burgin and Seto2021).
Regarding the contamination of Mars itself, should the planetary protection protocol between the Moon and Mars be any different?
The current COSPAR guidelines for missions to Mars are significantly different from those for the Moon, because Mars is considered to provide potentially habitable environments for indigenous Mars life. In addition, some environments on Mars, particularly in the subsurface, could be habitable for Earth organisms. Therefore, stringent limits are placed on the number of Earth organisms permitted on outbound missions to Mars, to reduce the potential for Earth organisms to be delivered to, and persist, on Mars.
There is no concern for contamination of the lunar surface, because the environment of the Moon is known to be uninhabitable by carbon-based life that functions in physical environments similar to the surface of Earth. In addition, for the purposes of planetary protection, Earth and the Moon are considered to be one system, which ensures no travel restrictions between these two bodies will be emplaced.
Planetary protection is inextricably linked with human health
The microbiomes of modern humans living in urban settings are less diverse than those of ancient indigenous peoples as shown by a range of recent seminal studies (De Filippo et al., Reference De Filippo, Cavalieri, Di Paola, Ramazzotti, Poullet, Massart, Collini, Pieraccini and Lionetti2010; Clemente et al., Reference Clemente, Pehrsson, Blaser, Sandhu, Gao, Wang, Magris, Hidalgo, Contreras and Noya-Alarcón2015; Eisenhofer et al., Reference Eisenhofer, Kanzawa-Kiriyama, Shinoda and Weyrich2020; Sprockett et al., Reference Sprockett, Martin, Costello, Burns, Holmes, Gurven and Relman2020; Weyrich, Reference Weyrich2021). Diversity within the human microbiome is reduced when our lifestyle isolates us from contact with biodiverse soils (Blum et al., Reference Blum, Zechmeister-Boltenstern and Keiblinger2019) and the microbiomes of astronauts are known to become impoverished due to isolation and from Earth's biosphere (Sugita and Cho, Reference Sugita and Cho2015). Therefore, we can expect that humans living on Mars will not enjoy optimal microbial biodiversity. The additional use of ethanol-containing (or other) wipes to cleanse the skin further reduces the biodiversity of skin. These factors, along with the use of antibiotics or other drugs that perturb and diminish the microbiome, will reduce the usual competition within the microbiome. Biodiverse, healthy and climax microbial communities are most likely to suppress the proliferation of pathogens or pathogenic events (Cray et al., Reference Cray, Bell, Bhaganna, Mswaka, Timson and Hallsworth2013).
Conversely, damage to microbial communities on and within the human body that are perturbed and diminished results in the creation open habitats (places that are resource-rich and promote proliferation of and competition between microbes) (Cray et al., Reference Cray, Bell, Bhaganna, Mswaka, Timson and Hallsworth2013). These include excessively cleaned skin, wounds and surfaces of the gastrointestinal tract after use of antibiotics. Many of the potentially pathogenic microbes that occur with the human microbiome have a robust stress biology and are therefore selected for by the use of skin disinfectants (Suchomel et al., Reference Suchomel, Lenhardt, Kampf and Grisold2019). They are also more likely to be capable of survival if faecal waste is used as a fertilizer and so can re-enter the body via the oral-faecal route due to consumption of contaminated foods (see below). In the event that any human(s) become(s) infected with a highly contagious microbial pathogen, it has yet to be determined how to prevent an epidemic within the human population of Mars. In the unfortunate event of a human fatality on Mars, there is likely to be a contingency plan to recover the body to Earth. However, the time delay until this occurs raises real questions about planetary protection and safeguarding the health of the other people remaining in the habitation(s).
Indeed, there are ethical, technical and scientific dilemmas about humans and microbes living on Mars that have yet to be resolved. In relation to microbes on Mars, it will be difficult to live with them, yet we cannot live without them.
Microbes for human medicine on Mars
Human Exploration needs to address potential issues related to the health and illness of astronauts. Therefore, a broad range of basic medicines is needed within the payload, as well as the technology to produce them on demand whilst in transit or in the habitation(s) on Mars. This includes any unexpected medicines needed (different from those brought from Earth) and supplementing any exhausted medical supplies. The miniaturization of portable scientific instruments for use during space exploration has also benefited biotechnology on the Earth (especially the development of micro-bioreactors, mini-microscopes, mini-DNA/-RNA sequencer). Many active pharmaceutical compounds are of plant origin but it is expected that if plants grow on Mars, they will be intended for food. Synthetic biology, via the use of metabolically engineered bacteria and yeasts, can be used to produce (some of these are already produced commercially on Earth) complex molecules such as antibiotics and the antimalarial drug (Ro et al., Reference Ro, Paradise, Ouellet, Fisher, Newman, Ndungu, Ho, Eachus, Ham and Kirby2006; Perez-Pinera et al., Reference Perez-Pinera, Han, Cleto, Cao, Purcell, Shah, Lee, Ram and Lu2016; Liu et al., Reference Liu, Cheng, Zhang, Ding, Duan, Yang, Kui, Cheng, Ruan and Fan2018; Malico et al., Reference Malico, Nichols and Williams2020). A culture collection of strains (kept freeze dried in small vials) to use for drug synthesis provides a choice for humans on Mars to be able to select the appropriate microorganism for growth in a micro-bioreactor and produce the required medicine. Currently, 3D printing technology can produce personalized medicine containing one or more active ingredients; this is already a reality on the Earth (Reddy et al., Reference Reddy, Venkatesh and Kumar2020).
Mars-related diseases, unknown on Earth, could arise and so plasticity of medical solutions and synthetic biology capability are required because there are considerable unknowns and astronauts need to have the capacity to respond and adapt to any eventuality. However, microorganisms in nature are metabolically versatile and are, for example, able to degrade chemically diverse hydrocarbons and other substrates (Timmis, Reference Timmis2002; Cray et al., Reference Cray, Bell, Bhaganna, Mswaka, Timson and Hallsworth2013) and produce an indefinite number of secondary metabolites (Cray et al., Reference Cray, Stevenson, Ball, Bankar, Eleutherio and Ezeji2015; Kai, Reference Kai2020). This capacity has value in metabolically engineering strains to produce biofuels and plastics (Nielsen et al., Reference Nielsen, Larsson, Van Maris and Pronk2013; Ko et al., Reference Ko, Kim, Lee, Han, Kim, Park and Lee2020) as well as pharmaceuticals (see above). Another example is provided by Saccharomyces cerevisiae that can produce the precursors of analgesics such as morphine (Pyne et al., Reference Pyne, Kevvai, Grewal, Narcross, Choi, Bourgeois, Dueber and Martin2020) and cannabinoids (Luo et al., Reference Luo, Reiter, d'Espaux, Wong, Denby, Lechner, Zhang, Grzybowski, Harth, Lin, Lee, Yu, Shin, Deng, Benites, Wang, Baidoo, Chen, Dev, Petzold and Keasling2019). Unresolved issues of using microbes on Mars for this purpose are whether mutations might occur, and whether drugs can be produced in sufficient quantities and whether they can be readily purified. Apart from its use in drug production, yeasts might be useful to produce vanilla and raspberry flavours for use as food additives to help avert a sense of homesickness and to add to the joie de vivre.
Mars and society
Society, ethics and theology on Mars exploration
In regard to societal, ethical, and religious aspects of Mars exploration it is useful to distinguish between human exploration and scientific exploration. This is often described as a science versus exploration scenario in space exploration (Metzger, Reference Metzger2016). Human Mars exploration can be understood as an expansion of Earth society, while scientific exploration enables Earth society to learn about its place in the Universe. This perspective has been relevant for philosophers and theologians for centuries. One example of such debate is the question of life on other planets. Thomas Payne as well as John Calvin have discussed the theological aspects of life on other planets (Weidemann, Reference Weidemann2014). For Immanuel Kant, life on other worlds was certain and this ‘knowledge’ informed his philosophical stance.
While scientific exploration of Mars via robotic probes and landers has been going on since the beginning of human space exploration, a human mission to Mars is still far-reaching. It is interesting to note, that the possible presence of life on Mars is one of the main drivers as well as one of the main obstacles in Mars exploration. The debate on planetary protection of Mars and its impact on mission profiles boils down to the ethical question of how we as humans should relate to possible extraterrestrial life. This ethics question has a wide range of answers: from very human-centred to biocentric perspectives that give all life the same value, and even, perspectives that ascribe non-inhabited areas of space the same value as inhabited areas. A religious perspective on this debate boils down to the question ‘what role humans play within God's plan or creation’. If we are at the centre of it, we still might be obligated to care for the rest of the species and not just profit from them. For Mars exploration, this could mean an obligation to care for possible life on the Red Planet. Chris McKay and Robert Zubrin have discussed this in relation to the settlement of Mars (Waltemathe and Hemminger, Reference Waltemathe and Hemminger2019). While McKay argues from a biocentric stance to change the environment of Mars in such a way as to enable indigenous bacterial life to prosper and enable humans to learn about life itself, Zubrin basically argues to freeze indigenous life and keep it in the lab while making the planet liveable for humanity. He employs a strong anthropocentric view of planetary colonization. Brian Green of the University of Santa Clara, in an opinion piece for CNN, questioned both positions from a philosophical as well as a theological point of view, and restructured their discussion to find a middle ground. All these discussions aim at giving the current planetary protection ruling an ethical framework. The current regulations are meant to protect scientific evidence from contamination through exploration. Their rationale is protection of science. One could argue, however, that this rationale needs an ethical and societal framework that includes more than science as a rationale (Sherwood et al., Reference Sherwood, Ponce and Waltemathe2019).
The theological implications of life on Mars and the societal response to the discovery have received scholarly attention (e.g. Vakoch, Reference Vakoch2013). The broader societal benefits of the planned robotic and potentially astronaut missions to Mars however relate to its immense inspirational and educational values and potentials. Such efforts can attract society and in particular young generations to expand the limits of our knowledge, to evoke interest in the study of sciences and related disciplines, draw attention to the values of international, peaceful cooperation in space, and even to the possibility of creating a sustainable human presence in the solar system beyond Earth. Using Mars as a teaching context, engaging students and general public with exploration efforts using the ever-popular Red Planet are all possible directions for science dissemination and popularization, engagement and even for correcting the misconceptions about science and space exploration (Capova et al., Reference Capova, Dartnell, Dunér, Melin and Mitrikeski2018).
What is the motivation for space exploration?
Human exploration of Mars often also has another motivational aspect: survival. Freeman Dyson frames it for interstellar exploration as assurance against even the worst imaginable of natural or man-made catastrophes that may overwhelm mankind within our Solar System (Dyson, Reference Dyson1968). Dyson also argues that a space colony would achieve ‘total independence from any possible interference by the home government’.
William E. Burrows in ‘The Survival Imperative’ (Burrows, Reference Burrows2004) also argues for safeguarding humanity not only through space exploration but also in space. He draws on the ideas of O'Neil and Sagan and points out the pros and cons of their concepts. This can be directly applied to possible Mars settlements. With SpaceX planning such settlements for the near future, this perspective becomes immediate.
If one is to understand the motivation for Mars exploration for safeguarding humanity's future in nearby and distant solar systems to save us from a cataclysmic event that might destroy our habitat on Earth, the religious connotation of Noah's Ark immediately comes to mind. This story that is part of the Jewish, Christian and Muslim tradition (e.g. Gen 6–11 in the biblical text, but equally present in the Quran in different suras) is part of the religious and cultural foundation of European, Mid-Eastern and American societies. Flood mythology, however, can be found in all major religious traditions. As a consequence, Mars exploration scenarios would be well advised to take these religious and cultural connotations into account and relate to their specific challenges and opportunities. Another motivation is establishing new resource bases for humanity, thereby lightening the load on Earth's resources. Robert Zubrin in ‘Entering Space’ builds his argument in a similar way (Harris, Reference Harris2000). Exploration as part of the human condition is something that is also argued in regard to space exploration and therefore to Mars exploration. The concept of ‘Manifest Destiny’, for example, is being invoked by the proponents of space exploration. William E. Burrows writes ‘At the heart of it all, as usual, (were) the core of dreamers…who steadfastly believed it was their race's manifest destiny to leave Earth for both adventure and survival’ (Burrows, Reference Burrows2010).
What has shaped public opinion about Mars?
Public attitudes about Mars are shaped by narrations created on Earth by science fiction authors, scientists, philosophers and politicians (Messeri, Reference Messeri2016). Historically, the Red Planet figures the public imagination about other worlds and its inhabitants. A prominent example is the 1897 novel ‘The War of the Worlds’ by H.G. Wells that has shaped the envisioning of an alien invasion and dominated a century-long narrative history. Today's explorers and science communicators are presented with a challenge of how to disseminate good science, its concepts and results in parallel to the many science fiction stories that are entertaining yet not always science-conformant. Here, for example, the robotic missions designed to find evidence of possible existence of life in habitable niche environments, e.g. Perseverance and Rosalin Franklin, have the capability to capture popular imagination as they seek answers to the questions: was there life on Mars, and what was it like?
Societal benefits of Mars exploration
Human and robotic space exploration responds to the deeply rooted quest of humankind for answering questions on the origins and nature of life in the Universe and extending human frontiers. More down-to-earth considerations are however important driving forces behind the actual decisions for investing in exploration programmes.
In the 1950s, geo-political considerations were the main driver for space exploration. What eventually evolved into a cold war space race to the Moon did and still continues to accumulate massive economic and societal benefits far beyond and above the outcomes initially envisaged. A study commissioned by the European Union in 2015 estimates that 6% of Europe's entire economy (including non-space) is directly dependent on space infrastructure and accounting for around 14 million jobs. Satellites are able to address about 60% of the 57 essential climate variables (ECVs), with several exclusively derived from satellite measurements. ECVs are critically contributing to the characterization of Earth's climate, providing a picture of climate change at a global scale. The Climate Change Initiative of the ESA generates consistent, long-term and global data records for 21 key ECVs.
With the satellite applications fully integrated into society and becoming mainstream, exploration of outer space and Mars still entails significant short-term and long-term benefits for society. This is largely recognized by the International Space Exploration Coordination Group (ISECG), a coordination mechanism of space agencies from 25 countries. Largely building on ISECG's vision and roadmap with horizon goal human exploration of Mars, ESA's European Exploration Envelope Programme (E3P) is implementing a benefits-driven European Space Exploration Strategy, prioritising the scientific, economic, inspirational and international cooperation dimensions of exploration. Earth orbit, Moon, and Mars (ESA's three exploration destinations) should be seen as part of a single coherent programme expanding in a step-wise manner the places where humans will work and live in space.
Earth sustainability
In addition to the discoveries, advancement of science, technological development, and economic return can space technologies address other societal issues and assist in achieving sustainable development on Earth? The United Nations' Office for Outer Space Affairs (UNOOSA) has recognized space as a driver for the sustainable development and acknowledged the unique potential of space technologies in leveraging innovative solutions and technological developments. While the example of the Earth observations and geospatial data have already earned their recognition in contributing to the UN Sustainable Development Goals (SDG, a universal call to action to end poverty, protect the planet and ensure that all people enjoy peace and prosperity by 2030), the actual potential of space applications in supporting the SDGs is much wider. One example is the technology developed for analysing the atmosphere of Mars that now helps to cut greenhouse emissions on Earth (ESA SDG Catalogue 2018). Space activities, among those of ESA and other space agencies, are an important tool serving development (Duvaux-Béchon, Reference Duvaux-Béchon2019). The challenge remains in utilising those technologies and maximising their potential to make life on Earth more productive, clean and sustainable, securing safe future for our planet and human generations to come.
Knowledge transfer from space exploration
The ISS, continuously crewed since 2000, shows the benefits and potential of human activity in low Earth orbit, from basic science to innovation and knowledge fuelling emerging terrestrial applications in fields such as air filtration, protein crystal growth, robotic surgery, water recycling, materials, fluids and combustion (Thumm et al., Reference Thumm, Robinson, Buckley, Johnson-Green, Kamigaichi, Karabadzhak, Nakamura, Sabbagh, Sorokin and Zell2012). The stringent challenges of space exploration to the next destinations are an accelerator for innovation. Exploration missions require the development of cutting-edge technology in areas such as advanced robotics, artificial intelligence and additive manufacturing (3D printing), and to enable next-generation life support, habitation and waste management systems for human exploration. This provides opportunities for other sectors to partner with the space sector on joint research and development. At the same time, scientific discoveries in weightlessness enabled by human exploration are applied widely. The results are touching every aspect of everyday life, from health and medicine, public safety, consumer goods, to energy and the environment, industrial productivity and transportation.
The process of developing a mission such as ESA's ExoMars 2022 mission sending the Rosalind Franklin Rover to search for life in the martian subsurface is already sparking spill-overs to the terrestrial economy. For example, welding techniques developed for the mission are also used to manufacture aluminium cans, with potential to save 12% on raw materials. A report commissioned by the UK Space Agency estimates that Rexam plc, a British-based multinational consumer packaging company and leading manufacturer of beverage cans, could have saved £242 million on raw materials in 2014 had the company implemented the technique. Mars Sample Return ground- and flight elements that ESA is contributing to an international end-to-end sample return capability will eventually allow scientists to analyse samples in terrestrial laboratories. Mars Sample Return will require the development of ground segments (such as the receiving and curation facilities) and a qualified workforce to handle the samples. This has the potential to push the boundaries of European technological achievement, directly creating thousands of high-tech jobs and spin-off industries to the benefit of the wider economy. Through the inspirational and motivational value of aiming high and beyond our current grasp, Mars exploration activities will result in increased participation of young people in STEM education and careers, thereby increasing their contribution to Europe's knowledge economy and capacity to address global challenges in the future. For example, Mars climate was once similar to the Earth's but has gone through massive changes. Importantly, Mars climate research may help better understand the past history and predict future changes in our Earth's climate (Read et al., Reference Read, Lewis and Mulholland2015).
Potential pitfalls of becoming an interplanetary species
If the arguments on the motivation of exploration accurately describe human nature in regard to exploration and discovery, then perhaps space exploration is the ultimate form of human destiny, opening up the borders of our own planet. This can be made compatible with major religious traditions including views on humanity's role in creation. It certainly would serve as a motivation, but also bring with it all the cultural problems that historically, exploration has resulted in on planet Earth. The question arises of how to mitigate the negative effects of such concepts when trying to apply them to space exploration. Currently, a shift in language can be observed, addressing the difficult concepts of the past and the expressions they have shaped. Space colonies are different from space settlements in the potential negative historical connotation of words such as colonization, exploitation or even mining. Crewed vehicles describe the social reality of contemporary space explorations better than manned missions of the past. Grounding exploratory motivation in the space between scientific curiosity and human experience may serve to mitigate the problems. Religious and philosophical traditions can serve to motivate exploration on one hand but also as a careful reminder of the problems that we were, and still are, connected to it. Gaining knowledge about the Universe maybe the more-desirable first step, which also fuels human exploratory drive but with caution derived from more information.
Epilogue
Mars could have been more Earth-like before. The similarities between Noachian Mars (~3.7–4.1 Ga ago) and early Earth have implications for the astrobiology of Mars, not least that traces of extinct martian life might remain at the planet's surface or subsurface. The tenuous atmosphere of present-day Mars evolved from the depletion of a thicker atmosphere. This drove a water cycle hosting numerous lakes and rivers that shaped the Noachian landscape. Whereas Earth evolved plate tectonics, Mars preserved two contrasting landscapes; the rough southern highlands and the smooth northern lowlands. How this ‘Mars dichotomy’ formed remains unanswered.
Evidence for Mars' aqueous past is recorded in surface minerals. Carbonates expand our understanding of carbon- and water cycles, as well as planetary habitability. They are commonplace on Earth but less abundant on the martian surface even though martian conditions could have been warm and wet; favourable for the formation of carbonates (in neutral pH water bodies). Although most carbonate on Mars formed under aqueous conditions (similar to Earth), some carbonates on Mars may have formed via other processes (that do not occur on Earth). Atmospheric CO2 photochemistry, or energetic electrons discharging martian dust particles are examples. Sulphates on Mars are mostly hydrated, supporting their formation under water-rich conditions, mostly during the Hesperian (~3.0–3.7 Ga ago). Hematite, clays, and amorphous hydrated silicates are more widespread, providing further evidence of an aqueous past.
As for martian water, studies of martian methane are motivated by the question of life on Mars. Whereas the mechanisms of biogenic methane formation on Earth have been identified, mechanisms of methane formation on Mars have yet to be elucidated. Methane levels are known to vary on Mars, but its origin remains unclear. Seasonal variation in martian methane can be attributed to either a biological origin or inorganic formation, e.g. via serpentinization, photochemistry or the reduction of CO2 over acidic minerals and clays.
We are yet to ascertain the existence of past or present life on Mars. Habitable environments need to be studied, although a habitable environment does/did not necessarily harbour life. This said, the identificiation of biosignatures on Mars (whether textural, chemical, or isotopic) would confirm the existence of once-habitable environments. However, for many kinds of biosignature, there is no simple way to affirm that ancient life once existed in a specific extraterrestrial location. Microorganisms from Earth might not survive the harsh conditions on Mars surface, and no biomolecules would remain intact without the protection of minerals. However, Mars is not necessarily universally biocidal, and pigmented microorganisms are the most resistant to EUV exposure. The search for biomolecules should be focused in the subsurface of Mars.
A better understanding of martian processes requires the characterization of Mars across diverse spatial and temporal scales. Martian meteorites have been the only martian samples available for study at the microscopic scale. After being recognized as martian only decades ago, we are now exploring where on Mars these meteorites could have been ejected from. Cosmic ray exposure ages, determined via analyses of noble gasses, can indicate ejection times, grouping the martian meteorite ejection events and constraining the number of impact craters associated with them. An impact event that led to the Mojave Crater has been hypothesized to have ejected some of the shergottite martian meteorites. However, determination of martian meteorite source locations remains a challenge even with accurate mineralogical data on both Mars and its meteorites.
Mars exploration will intensify during the coming decade. The increasing number of missions to Mars will improve our understanding of the Red Planet, increasing the likelihood of human habitability. In parallel, advancements made in human space exploration on the ISS and eventually the Moon will catalyse human exploration of Mars. First and foremost, will be to determine where humans could safely inhabit Mars. The elevation of selected sites is important due to the landing risks; atmospheric breaking technologies need to be established. The major technology drivers required to achieve this include resource utilization, i.e. converting CO2 into methane and oxygen for propellant fuel, life- and resource-support technologies, and water extraction from the polar ice and permafrost. Martian regolith and dust can be used with manufacturing technologies to produce human habitations. When sustainable agriculture is achieved, human habitability is finally supported. Further to that, additional risks associated with societal structures and human settlements require quantification and evaluation.
Humanity's encounter with a possibly inhabited world requires careful consideration of the United Nations resolutions on planetary protection. Contamination of Mars could occur from Earth as well as any potential bio-risk to Earth upon human or robotic return from Mars. This is particularly a concern towards the subsurface of Mars that could retain Earth-based organisms if contaminated. Precise countermeasures are required to prevent contamination from vehicles and instruments sent to Mars, and eventually from humans. An accurate characterization of the local martian environment for extant life is necessary prior to any human activities. If any forms of extant life were to be discovered, would it be ethical to tamper with their course of their evolution? Solving such ethical questions whilst sustaining human development on Mars is just as challenging as the technological feat itself.
Current and future Mars missions outline the technological achievements through which economic benefits arise and nations are brought together. Mars exploration will also support STEM education and careers addressing the global challenges we face together on Earth. Mars will ultimately act as a testing ground for humanity becoming an interplanetary species. This frontier is becoming more realistic, despite the technological challenges that need to be solved. These challenges, which involve the conservative and efficient use of resources, could also facilitate improved sustainability and economic prosperity on Earth.
Acknowledgements
HGC was supported by the NSFC ‘Young International Scientist Award’ Grant No. ED was supported by the Canadian Space Agency Grant No. 18FASASB13. 41750110488. MF acknowledges grants nos. 19-03314S of the Czech Science Foundation and ERDF/ESF ‘Centre of Advanced Applied Sciences’ (No. CZ.02.1.01/0.0/0.0/16_019/0000778). FXH was funded by the B-type Strategic Priority Program of the Chinese Academy of Sciences (Grant No. XDB41000000). Lauren Edgar of the United States Geological Survey is thanked for her contribution in the ‘Robotic and remote exploration of Mars’ section of this article. The National Space Science Centre of the Chinese Academy of Sciences and the Space Studies Board of US National Academy of Sciences' National Research Council's 9th and 10th New Leaders in Space forum organizers Dr Quanlin Fan, Chinese Academy of Sciences, China and Dr David Smith, US National Academy of Sciences, USA are also thanked for bringing together some Mars scientists for this article.