INTRODUCTION
During geological, geomorphological, soil, and quaternary investigations, buried fossil-soil layers are often found in the landforms, sediment, or soil profiles studied. These fossil soil layers in a stratigraphic section often provide irreplaceable information about past environmental events and changes. Different absolute dating methods help to understand the time of surface development, paleoclimatological and environmental changes in these investigations. The most widely used methods for determining the timing of environmental changes are optically stimulated luminescence (OSL) and radiocarbon (14C) dating. There are an increasing number of studies that use these methods in sandy aeolian environments. Both methods (OSL and 14C) can be applied to the development of aeolian sand dunes (Gardner et al. Reference Gardner, Mortlock, Price, Readhead and Wasson1987; Bitinas et al. Reference Bitinas, Damušyte, Hütt, Jaek and Kabailiene2001; Nyári et al. Reference Nyári, Kiss and G2007; Kiss et al. Reference Kiss, Sipos, Mauz and Mezosi2012; Buró et al. Reference Buró, Sipos, Lóki, Andrási, Félegyházi and Négyesi2016, Reference Buró, Lóki, Gyori, Nagy, Molnár and Négyesi2019; Miao et al. Reference Miao, Wang, Hanson, Mason and Liu2016; Bosq et al. Reference Bosq, Bertran Pa, Kreutzer, Queffelec, Moine and Morin2018; Moska et al. Reference Moska, Jary, Sokołowski, Poręba, Raczyk, Krawczyk, Skurzyński, Zieliński, Michczyński, Tudyka, Adamiec, Piotrowska, Pawełczyk, Łopuch, Szymak and Ryzner2020, Reference Moska, Sokołowski, Jary, Zieliński, Raczyk, Szymak, Krawczyk, Skurzyński, Poręba, Łopuch and Tudyka2021).
If these soils contain sufficient amounts of macroscopic charcoal or other macrofossils for 14C dating, 14C ages may provide the most accurate information on the timing of soil formation (Goble et al. Reference Goble, Mason, Loope and Swinehart2004; Buró et al. Reference Buró, Sipos, Lóki, Andrási, Félegyházi and Négyesi2016, Reference Buró, Lóki, Gyori, Nagy, Molnár and Négyesi2019; Miao et al. Reference Miao, Wang, Hanson, Mason and Liu2016; Aleksandrovskii et al. Reference Aleksandrovskii, Ershova, Ponomarenko, Krenke and Skripkin2018). Charcoal fragments usually are one of the best age markers, in the case of an eolian deposited environment. Charcoal samples represent the short growth period of a plant, and their 14C ages determine the time of the fire events in the past. Charcoal usually should give very reliable age and information about landscape and landforms development, nevertheless fossil soils do not always contain sufficient charcoal remains.
However, it is often the case that fossil soil layers do not contain enough charcoal or macrofossils for 14C dating. In these cases, 14C dating from different fractions (i.e., soil organic matter, humic acid, humic, etc.) of the bulk sediment has been used in several studies as a last resort, although their reliability is still a matter of debate (Scharpenseel and Becker-Heidmann Reference Scharpenseel and Becker-Heidmann1992; Wang et al. Reference Wang, Amundson and Trumbore1996; Trumbore Reference Trumbore2000; McGeehin et al. Reference McGeehin, Burr, Jull, Reines, Gosse, Davis, Muhs and Southon2001; Pessenda et al. Reference Pessenda, Gouveia and Aravena2001; Mayer et al. Reference Mayer, Burr and Holliday2008; Holmquist et al. Reference Holmquist, Finkelstein, Garneau, Massa, Yu and MacDonald2016; Cheng and Fu Reference Cheng and Fu2020).
Several studies have investigated the reliability and usefulness of the two-step combustion method, for bulk 14C age determination in case of different paleo environment. In this process, the same sample is combusted at low (400°C) and subsequently at high (800–900°C) temperatures. At the low temperature, the non-charred volatile organic component is combusted. This fraction is usually younger in 14C age than the high temperature fraction. The 14C age of the high temperature fraction is derived from the charred fraction organic component of the soil organic matter. This fraction is usually older than the low temperature fraction (McGeehin et al. Reference McGeehin, Burr, Jull, Reines, Gosse, Davis, Muhs and Southon2001; Mayer et al. Reference Mayer, Burr and Holliday2008; Zhu et al. Reference Zhu, Cheng, Yu, Yu, Kang, Yang, Jull, Lange and Zhou2010; Cheng and Fu Reference Cheng and Fu2020). In those studies, the results were usually consistent and in good agreement with the other types of 14C and optical stimulated dating methods, but very sensitive to the younger organic matter infiltrating from overlying horizons.
In this present study, we also investigated the reliability of the two-step combustion method for 14C dating of soil bulk samples, because in previous research, it was reported often the fossil soils of the Hungarian sand dunes did not contain enough charcoal to determine the 14C age (Borsy et al. Reference Borsy, Csongor, Félegyházi, Lóki and Szabó1981). Furthermore, in recent years, we have encountered several sand dunes in the Nyírség (NE Hungary) blown sand area, where we found fossil soils, but they also did not contain enough macroscopic charcoal even for AMS 14C dating (Buró Reference Buró, Sipos, Lóki, Andrási, Félegyházi and Négyesi2016). Lack of 14C ages from a site is a major disadvantage, as it means there is no information about the time of the landscape evolution, the periods of the sand movements and the development of the paleosols and landforms. In our previous study (Buró et al. Reference Buró, Lóki, Gyori, Nagy, Molnár and Négyesi2019) we have already noted that the soil organic carbon (SOC) of fossil soils from the Nyírség blown sand area might give useful 14C ages. Thus, in this present study, our aim was to determine the reliability and limitations of the different fractions of the soil organic carbon (SOC) 14C ages in this blown-sand sampling area. The SOC 14C age of fossil soils were compared with the charcoal ages from the same fossil soil horizon, and their (SOC and charcoal) age reliability was verified independently by the OSL method.
Sampling Sites
The Nyírség, which was formed on the alluvial deposits of the Tisza and Bodrog Rivers and their tributaries, is the second largest blown-sand dune area in the Carpathian Basin and in Hungary. Around 25 ka ago, fluvial processes terminated in this area (Borsy Reference Borsy1991; Lóki Reference Lóki2006). The alluvial fan dried out and wind became the dominant geomorphic agent. The first significant sand movement was during the Upper Pleniglacial and the Late Glacial (Borsy et al. Reference Borsy, Csongor, Félegyházi, Lóki and Szabó1981; Borsy Reference Borsy1991; Lóki et al. Reference Lóki, Hertelendi and Borsy1994; Buró et al. Reference Buró, Sipos, Lóki, Andrási, Félegyházi and Négyesi2016). In these periods, the main deflation (deflation depression, deflation hollows, etc.) and accumulation landforms (sand hummock, parabolic dunes, asymmetric parabolic dunes, etc.) developed. During warmer and humid periods, where the paleoenvironmental conditions were favorable, soil formation occurred, mainly during the Bölling-Allerod phase (Gábris Reference Gy2003; Buró et al. Reference Buró, Lóki, Gyori, Nagy, Molnár and Négyesi2019).
According to the Köppen−Geiger climate classification (Kottek et al. Reference Kottek, Grieser, Beck, Rudolf and Rubel2006), a warm summer and humid continental climate is typical for the area. The mean annual temperature is 9.6–9.8°C and the average annual rainfall is 550–650 mm (Borsy Reference Borsy1961; László et al. Reference László and Bottyán Zs2016).
This area is characterized by the following soils: Lamellic Arenosols, Luvisols, Gleysols, Cambisols, and Phaeozems (Novák et al. Reference Novák, Négyesi, Andrási and Buró2014). The main texture types of the soils are sand, sandy loam, and loamy sand. The carbon content of the sand dunes of Nyírség is very low, higher organic carbon content can be found only in the recent top soil horizon and in fossil soils (Novák et al. Reference Novák, Négyesi, Andrási and Buró2014; Buró et al. Reference Buró, Sipos, Lóki, Andrási, Félegyházi and Négyesi2016).
The study area is situated within the forest-steppe zone and potential natural vegetation could be oak-forest steppes. Planted forests, ploughed lands and grasslands are the main land-use forms. Recently, there has been reforestation using different non-native species (Robinia pseudo-acacia, Quercus rubra, etc.) (Borhidi and Sánta Reference Borhidi and Sánta1999; Zagyvai and Bartha Reference Zagyvai and Bartha2015).
METHODS
Sampling
From the Nyírség blown sand area, 8 stabilized sand dunes were chosen for sampling (Table 1; Figures 1 and 2). These dunes contain clearly observable fossil soil layers (Figure 2).
Table 1 Major properties of the sampling sites.
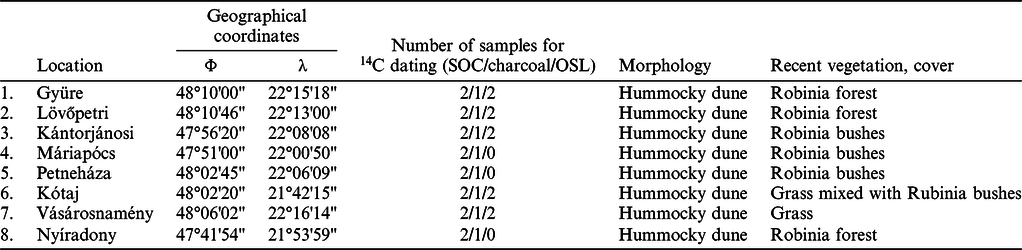
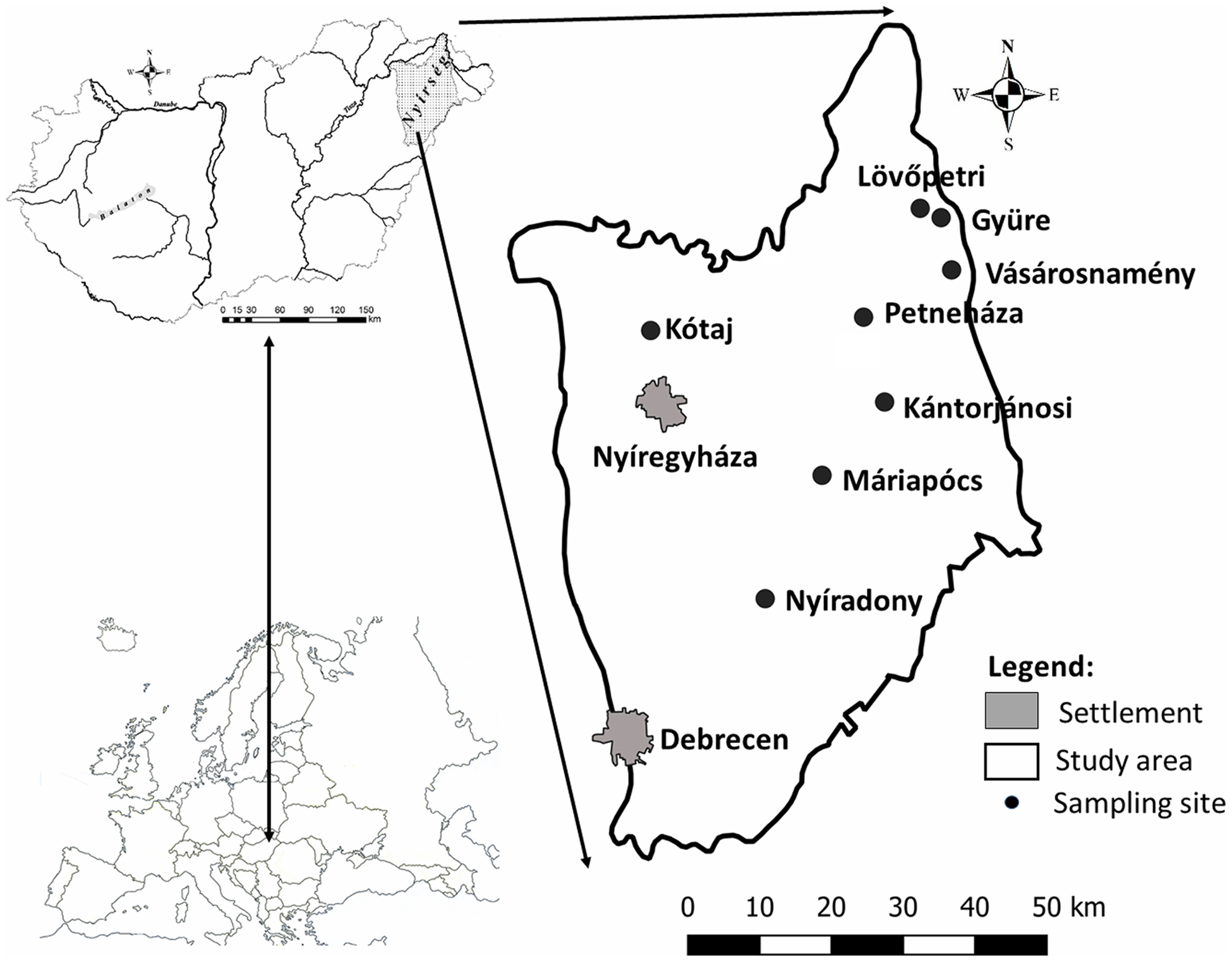
Figure 1 Locations of the sampling sites in the Nyírség (Hungary).
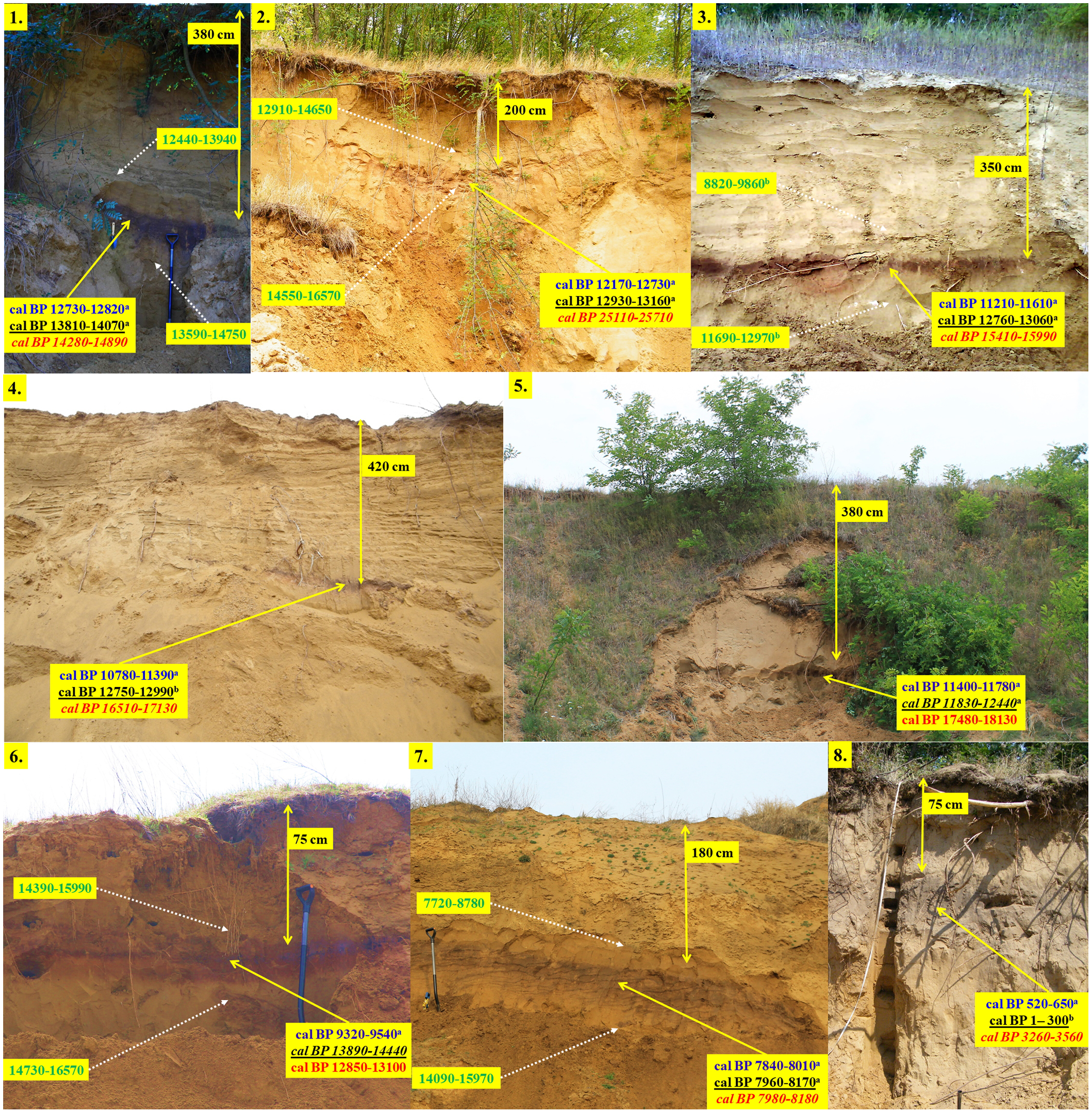
Figure 2 Sections of the sand quarries studied: 1: Gyüre, 2: Lövőpetri, 3: Kántorjánosi, 4: Máriapócs, 5: Petneháza, 6: Kótaj, 7: Vásárosnamény, 8: Nyíradony. (aPublished in Buró et al. Reference Buró, Lóki, Gyori, Nagy, Molnár and Négyesi2019; bPublished in Buró et al. Reference Buró, Sipos, Lóki, Andrási, Félegyházi and Négyesi2016.)
For accelerator mass spectrometry (AMS) 14C measurements, we collected soil samples from the fossil soil horizon (Table 1; Figures 1 and 2) at 8 different locations. For 14C AMS age determination, charcoal samples were also collected from all 8 outcrops (Table 1; Figures 1 and 2). The charcoal and soil samples came from the same horizon.
From 5 locations, OSL samples were also collected 50 cm below (Lower OSL) and above (Upper OSL) the investigated fossil soils to control the 14C results (Table 1; Figures 1 and 2). Some of these ages have already been published in Buró et al. (Reference Buró, Sipos, Lóki, Andrási, Félegyházi and Négyesi2016, Reference Buró, Lóki, Gyori, Nagy, Molnár and Négyesi2019).
Radiocarbon Dating
14C AMS analysis of charcoal and bulk soil samples were performed at the Hertelendi Laboratory of Environmental Studies (HEKAL) AMS laboratory, Debrecen, Hungary (Molnár et al. Reference Molnár, Janovics, Major, Orsovszki, Gönczi, Veres, Leonard, Castle, Lange, Wacker, Hajdas and Jull2013a).
From the soil samples, roots and other microscopically identifiable plant remains were removed. Then, inorganic carbonates of the bulk soil samples (2–3 g) were removed by leaching in 2 mL 1M HCl at 75°C, for at least 2 hr. The samples were washed with distilled water until neutral pH then dried in a hot oven at 60°C. Dry samples were measured into quartz reaction tubes and attached to a dedicated vacuum line for on-line combustion (Jull et al. Reference Jull, Burr, Beck, Hodgins, Biddulph, Gann, Hatheway, Lange and Lifton2006; Molnár et al. Reference Molnár, Janovics, Major, Orsovszki, Gönczi, Veres, Leonard, Castle, Lange, Wacker, Hajdas and Jull2013a).
First, samples at low temperature (400°C) were combusted with high purity oxygen and then CO2 was extracted. This is labeled as the low-temperature (LT) non-charred fraction of SOC (i.e., LT-SOC), which is derived from younger, non-charred organic matter, volatile, and other organic components of soils (Zhu et al. Reference Zhu, Cheng, Yu, Yu, Kang, Yang, Jull, Lange and Zhou2010; Cheng and Fu Reference Cheng and Fu2020).
Second, the same sample was also combusted at high temperature (800°C). This fraction is labeled the “HT” charred fraction of SOC (i.e., HT-SOC). The organic matter and carbon of this component is considered to originate from the lattice of minerals (Zhu et al. Reference Zhu, Cheng, Yu, Yu, Kang, Yang, Jull, Lange and Zhou2010; Cheng and Fu Reference Cheng and Fu2020). This fraction represents carbon stored over longer time in the clay mineral fraction.
The LT-SOC 14C age is normally younger than the HT-SOC (Cheng and Fu Reference Cheng and Fu2020).
Due to the rather low organic content of the bulk soil samples (typically 0.1–0.6%), we generally combusted 1–2 g of each bulk sample. In every case, combustion resulted at least 0.2 mg C/sample for both fractions (LT and HT), which allowed preparation of normal graphite targets for the AMS 14C analyses.
Charcoal macrofossil fragments were treated using the standard acid-base-acid (ABA) method, i.e., a sequence of 1M HCl, distilled water, 1M NaOH, distilled water, and then 1M HCl at 75°C, for 1–2 hr each step (Jull et al. Reference Jull, Burr, Beck, Hodgins, Biddulph, Gann, Hatheway, Lange and Lifton2006; Molnár et al. Reference Molnár, Janovics, Major, Orsovszki, Gönczi, Veres, Leonard, Castle, Lange, Wacker, Hajdas and Jull2013a). After the final acid wash, the sample was washed with distilled water until neutral pH and then freeze-dried. Dry samples were measured into quartz reaction tubes and attached to a dedicated vacuum line for on-line combustion.
For charcoal, the two-step combustion method was also applied in the presence of high-purity oxygen gas: first the sample is combusted at low temperature (400°C) and the CO2 gas was extracted and afterwards in a second combustion step at high temperature (800°C), a second CO2 sample was collected from the same sample (Jull et al. Reference Jull, Burr, Beck, Hodgins, Biddulph, Gann, Hatheway, Lange and Lifton2006; Molnár et al. Reference Molnár, Janovics, Major, Orsovszki, Gönczi, Veres, Leonard, Castle, Lange, Wacker, Hajdas and Jull2013a).
The CO2 gas sample products from both steps were collected and purified separately using an on-line combustion system and later converted to graphite using the sealed tube graphitization method (Rinyu et al. Reference Rinyu, Molnár, Major, Nagy, Veres, Kimák, Wacker and Synal2013). IAEA–C9 (fossil wood) standards were treated and measured in parallel to the samples to check the quality of the sample preparation.
All 14C measurements were made on the graphitized samples using a compact 14C AMS system (Environ MICADAS) (Synal et al. Reference Synal, Stocker and Suter2007; Wacker et al. Reference Wacker, Bonani, Friedrich, Hajdas, Kromer, Němec, Ruff, Suter, Synal and Vockenhuber2010) at HEKAL (Molnár et al. Reference Molnár, Rinyu, Veres, Seiler, Wacker and Synal2013b). NIST SRM 4990C standards and borehole CO2 blanks were used for normalization of the AMS measurements. The results were corrected for decay of the standard and the effect of δ13C isotopic fractionation (Synal et al. Reference Synal, Stocker and Suter2007). For data reduction of the measured values, we used the “BATS” software (Wacker et al. Reference Wacker, Bonani, Friedrich, Hajdas, Kromer, Němec, Ruff, Suter, Synal and Vockenhuber2010).
Conventional 14C ages were converted to calendar ages using Calib 8.1.0 software (Stuiver and Reimer Reference Stuiver and Reimer1993) and the IntCal20 calibration curve (Reimer et al. Reference Reimer, Austin, Bard, Bayliss, Blackwell, Bronk Ramsey and Butzin2020). Calibrated ages are reported as age ranges at the 2-sigma confidence level (95.4%).
Luminescence Dating
Optically stimulated luminescence (OSL) measurements were performed in the Luminescence Laboratory of the Department of Geoinformatics, Physical and Environmental Geography, University of Szeged. Sample preparation was made under subdued yellow light, provided by low pressure Na-lamps. The samples were first dry sieved to obtain the 90–150 µm grain size fraction. Carbonates and organics were then removed using 10% HCl and 10% H2O2, respectively. Quartz grains were separated with heavy liquid flotation (LST Fastfloat), by setting densities to 2.63 and 2.68 g/cm3. Finally, the quartz extract was etched in 40% HF for 45 min. The treated quartz grains were mounted on 1-cm-diameter steel discs using a 6-mm mask.
The laboratory equivalent of the naturally absorbed dose was measured by a RISØ DA-20 TL/OSL luminescence reader equipped with a calibrated 90Y/90Sr beta source, blue (470 nm) and infrared (870 nm) LEDs, and an EMI ET9107-type photomultiplier tube with a Hoya (U-340) blue filter in front of the detection window (Bøtter Jensen et al. Reference Bøtter-Jensen, Thomsen and Jain2010). Equivalent doses (De) were determined by applying the single aliquot regeneration (SAR) protocol. OSL stimulation was performed at 125°C (heating rate: 5°C/s) for 40s, blue LEDs were set for 90% power release (Murray and Wintle, Reference Murray and Wintle2003, Wintle and Murray, Reference Wintle and Murray2006). Optimal preheating conditions were identified on the basis of preheat and dose recovery tests. For most of the samples, a 10 s preheat at 200°C and a cut heat of 160°C was applied. The purity of quartz extracts was monitored by measuring the IR/OSL depletion ratio at the end of the SAR measurements (Duller Reference Duller2003). Dose response curves were fitted by using a single saturating exponential function in the Analyst v4.57 software. Appropriate aliquots for De calculation were selected by applying standard rejection criteria given by Murray and Wintle (Reference Murray and Wintle2000). The statistical parameters of single aliquot De distributions were run through the decision process advised by Arnold et al. (Reference Arnold, Bailey and Tucker2007) to identify the appropriate age model for sample De calculation (Galbraight et al. Reference Galbraith, Roberts, Laslett, Yoshida and Olley1999). In most of the cases De distributions did not refer to incomplete bleaching (see Figure 4), and therefore, the minimum age model was applied only for one sample (Table 3). Sample De values were calculated using the Luminescence Package of R (Kreutzer et al. Reference Kreutzer, Schmidt, Fuchs, Dietze, Fischer and Fuchs2012), while De distributions were plotted using abanico plots (Dietze et al. Reference Dietze, Kreutzer, Burow, Fuchs, Fischer and Schmidt2016).
Table 2 Summary of the OSL and radiocarbon ages.
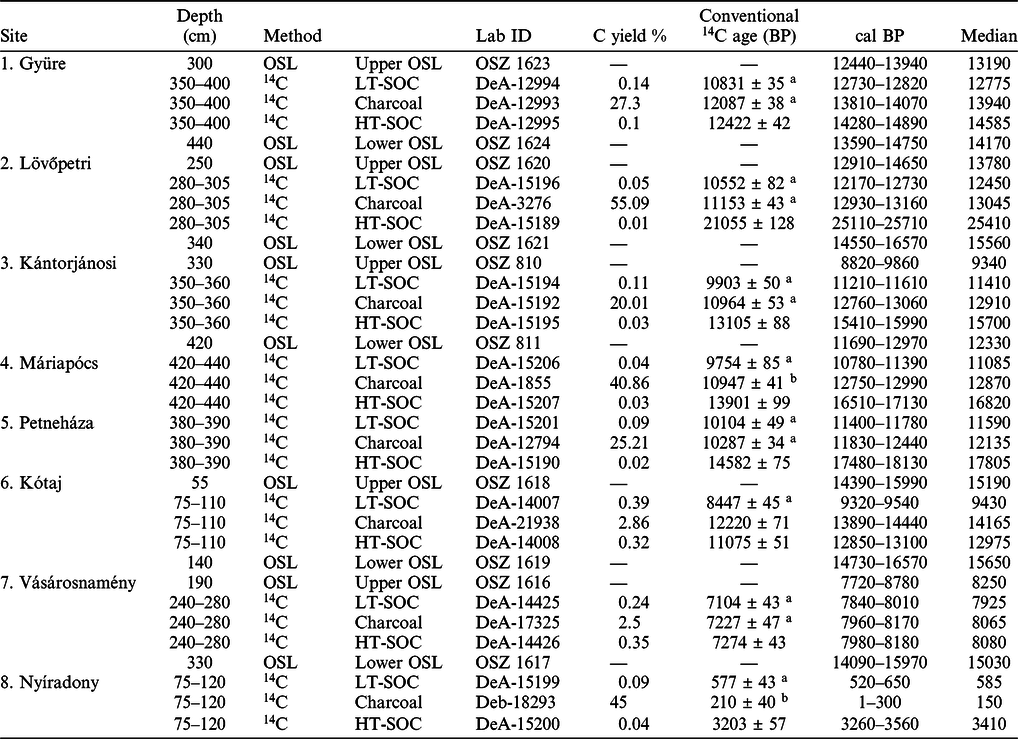
a Published in Buró et al. (Reference Buró, Lóki, Gyori, Nagy, Molnár and Négyesi2019).
b Published in Buró et al. (Reference Buró, Sipos, Lóki, Andrási, Félegyházi and Négyesi2016).
Table 3 Summary of De statistics. Values exceeding age model decision thresholds proposed by Arnold et al. (Reference Arnold, Bailey and Tucker2007) are underlined. Selected sample De is highlighted in bold.
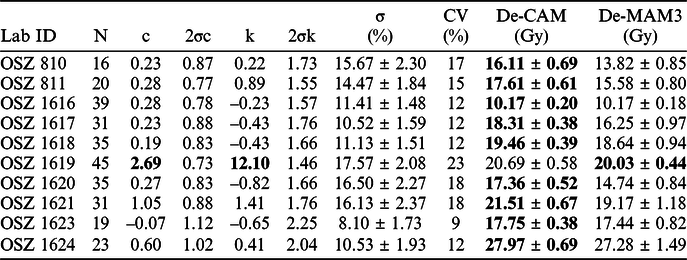
N: number of aliquots passing rejection criteria; c: skewness; σc: standard error of skewness; k: kurtosis; σk: standard error of kurtosis; σ: overdispersion value, CV: coefficient of variation, CAM: Central Age Model, MAM3: 3-parameter minimum age model.
The environmental dose rate (D*), i.e., the specific activity of the U and Th chain and 40K in the sediment samples, was determined with high resolution gamma spectrometry (Canberra XtRa Coaxial Ge detector). The conversion factors of Liritzis et al. (Reference Liritzis, Stamoulis, Papachristodoulou and Ioannides2013) were used to calculate dry dose rates. Corrections due to β attenuation were made after Brennan (Reference Brennan2003). Wet dose rates for the different radioactive components were calculated on the basis of in-situ and estimated water contents, using the equations of Aitken (Reference Aitken1985). The cosmic dose rate was assessed from the burial depth, altitude and geographic position of samples (Prescott and Hutton Reference Prescott and Hutton1994).
Ages, calculated as the ratio of De and D*, are reported with a 1-σ confidence level (Table 4). For better comparability OSL ages were converted to 14C calibrated BP ages. For better comparability, all 14C data are reported as calibrated BP ages. Chronology calculations and discussion was made using the median values of the ages.
Table 4 Dose rate, equivalent dose and OSL age results of the investigated sand samples.
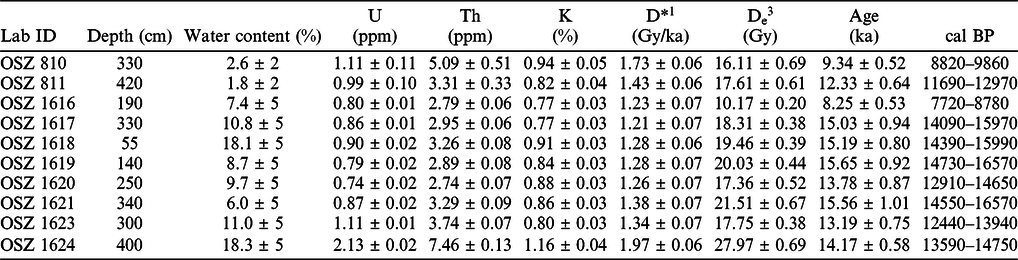
D*: total dose rate; De: equivalent dose.
RESULTS AND DISCUSSION
The primary aim of this study was to compare 14C and OSL ages of different soil layers, and to establish the reliability of the LT and HT-SOC 14C ages in dating of fossil soils of alluvial fans in a blown-sand area. Bulk soil and charcoal 14C ages were controlled by OSL age determination and also by 14C age data obtained from organic macrofossil fragments form the same soil horizons. The results of 14C and the OSL analyses are shown in Figures 2 and 3 and summarized in Table 2.

Figure 3 Calibrated BP values of the different type of ages from each sampling sites.
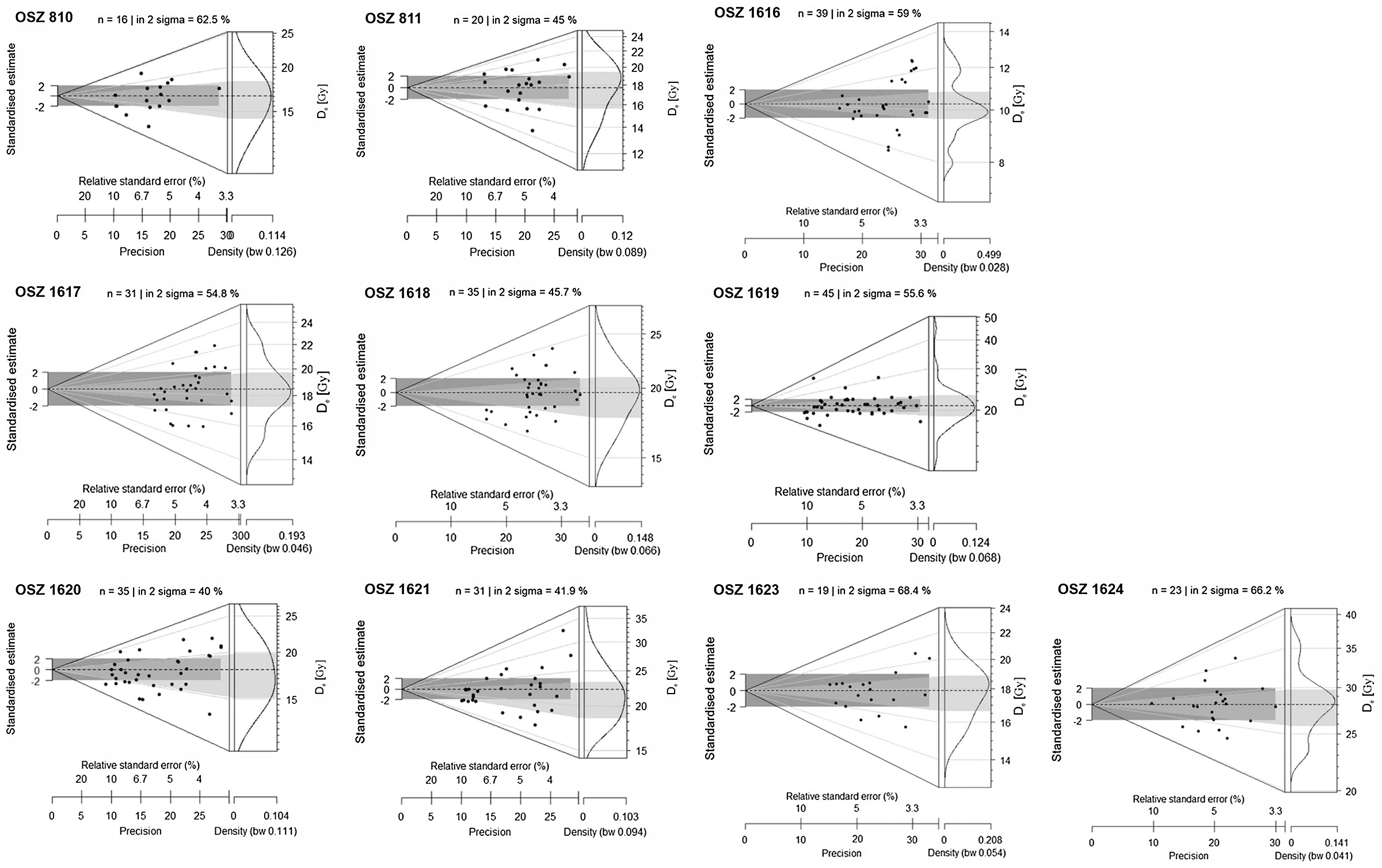
Figure 4 Distribution of single aliquot De results, shown on abanico plots (Dietze et al. Reference Dietze, Kreutzer, Burow, Fuchs, Fischer and Schmidt2016).
The different types of 14C ages (LT-SOC, HT-SOC and charcoal macrofossil) ideally should fall between the OSL ages, as the OSL data indicate the burial age of the neighboring sand layers which enclose the paleosol. Charcoal fragments in a layer represent a short period of time, as it is linked of the lifetime of a plant. The plants that may be destroyed by fire have lived and grown on the (previously formed) soil. Thus, the charcoal fragment age should fall between the LT-SOC and HT-SOC bulk 14C ages of the soil organic matrix. Normally, SOC carbon is a result of longer time carbon integration into the soil during formation. The charred fraction of the SOC (HT-SOC) might be older than the non-charred fraction of SOC (LT-SOC), as the charring processes take time in the soil (McGeehin et al. Reference McGeehin, Burr, Jull, Reines, Gosse, Davis, Muhs and Southon2001; Mayer et al. Reference Mayer, Burr and Holliday2008; Cheng and Fu Reference Cheng and Fu2020). Therefore, the different ages are expected to be in the following chronological order: Upper (thus younger) neighboring layer OSL age, LT-SOC age of the middle position soil layer, Charcoal age (short lived, contemporary with LT- or between LT- and HT- SOC ages), HT-SOC (as charring in soil needs longer time) and finally the Lower (thus older) neighboring layer OSL, if all assumptions are correct above, but we note exceptions below.
Charcoal Reference Ages
In the case of charcoal samples, only the H-fraction ages were reported as in Újvari et al (2016). Charcoal 14C ages are applied as reference ages, as those are normally used in soil layer 14C dating. This approach is also supported by our new results.
Charcoal versus LT- and HT-SOC
Charcoal ages (0.2–14.2 kyr) (n = 8) are convincingly well separated from soil ages, older than LT-SOC and younger than HT-SOC 14C ages (Figures 2 and 3; Table 2).
There are two exceptions. The first is Kótaj, where the charcoal is older by 1.2 kyr than the HT-SOC and with 4.7 kyr than the LT-SOC. The charcoal 14C age is the correct one, as confirmed by the OSL data (Figures 2 and 3; Table 2).
Presumably, the LT- and HT-SOC 14C ages are problematic, as will be explained further in the section “Non-Charred Soil Organic Carbon (LT-SOC) 14C Ages.”
The other exception is Nyíradony, where the charcoal age much younger (0.4 and 3.3 kyr), than the soil LT- and HT-SOC 14C ages. This difference appears to be due to anthropogenic influences, which will be also discussed below in the section “Non-Charred Soil Organic Carbon (LT-SOC) 14C Ages.”
Charcoal versus OSL
The charcoal 14C ages (8.1–14.2 kyr) (n=5) are in good agreement with the Upper OSL (8.3–15.2 kyr) and the Lower OSL (11.4–15.6 kyr) data and this result gives realistic ages (Figures 2 and 3; Table 2). In case of and Kántorjánosi this is clear, while at Gyüre, Kótaj, Lövőpetri and Vásárosnamény are within errors, where the charcoal older than the OSL age (Upper OSL) of the covering sand layer. However, at Kótaj, the Upper OSL (15.2 kyr) data is 1.1 kyr older than the charcoal age (14.1 kyr). These charcoal ages are close to the Upper-OSL ages. Here, we assume that the charcoal age was determined a fire event, as a result soil formation ended and the blown-sand movement took place again, by which time the soils were buried. The time of burial of the fossil soil with sand is given by the Upper-OSL age.
Reviewing the charcoal (8.1–14.2 kyr) and Lower OSL (12.3–16.1 kyr) (n=5) data, it seems at Gyüre, Lövőpetri, Kótaj and Vásárosnamény the charcoal is clearly younger, but in the case of Kántorjánosi it is younger but consistent within the error (Figures 2 and 3; Table 2).
The charcoal samples investigated give a reliable age which are consistent with the independent OSL data. Based on these results, we can assert that these charcoal data can be used well as reference age markers. This material is one of the best age markers in the Nyírség blown sand area, because in this aeolian environment, there is no risk of removal of the charcoal pieces by erosion (Mayer et al. Reference Mayer, Burr and Holliday2008) or redeposition in fluvial environments.
Non-Charred Soil Organic Carbon (LT-SOC) 14C Ages
LT-SOC versus OSL
The LT-SOC ages (7.9–12.8 kyr) (n=5) of the fossil soils from the 5 localities are closer to the Upper OSL (8.3–15.2 kyr) data (Figures 2 and 3; Table 2). In case of the Kántorjánosi outcrop, the LT-SOC age was significantly older than the Upper OSL ages. The LT-SOC ages of Gyüre and Vásárosnamény may be older than Upper OSL but are within error. In these sampling locations, the median values of the Upper OSL ages are slightly older (0.5–0.3 kyr) than the LT-SOC ages. This phenomenon may be caused by the much higher error of OSL compared to the 14C ages. This can explain also the fact that at Lövőpetri LT-SOC age was younger and slightly outside of the range of the Upper OSL age.
The LT-SOC (7.9–12.8 kyr) ages (n=5) were significantly younger in these five cases than the Lower OSL (12.3–16.0 kyr) ages (Figures 2 and 3; Table 2).
The results seem contradictory in the case of Kótaj, because the Upper OSL age is much older than the LT-SOC age of fossil soil, with a difference of 5.7 kyr. We believe this may be due to incomplete bleaching of the quartz grains during the last re-deposition.
However, since the Upper OSL age and the 14C age of charcoal are in agreement, within error, and the Upper OSL is younger than the charcoal (Figure 3; Table 2), it is more likely that the measured LT-SOC age appears to be younger than its true age. This may be due to that a soil solution leaching down the soil profile, transporting younger organic matter and carbon from the surface into the deeper layers of the fossil soil. This process modifies the 14C age of the SOC and would make the LT-SOC age appear younger than the true LT-SOC age. This phenomenon is much more likely to occur in shallow fossil soils than in deeper ones. In this location, the fossil soil layer is only at 75 cm deep (Figure 2).
LT-SOC versus Charcoal
Comparing the age of the LT-SOC fraction (0.6–12.8 kyr) (n=8) with the charcoal ages (0.2–14.2 kyr), it appears that the LT fraction is younger at each sampling location, except for Nyíradony (Figures 2 and 3; Table 2).
The results show that the LT-SOC ages are in a good agreement with our hypothesis, that they should be younger than the charcoal data, with only one exception (Nyíradony). The LT-SOC fractions appear to be in better agreement with the OSL ages, which suggests that these results may be a better reflection of the fossil soil ages, specifically the burial time of the fossil soils (Mayer et al. Reference Mayer, Burr and Holliday2008).
In the case of Nyíradony, the 14C age of the charcoal is younger (0.4 kyr) than the LT-SOC fraction. The development of this buried fossil soil stopped due to anthropogenic disturbance, since in the 18th and 19th centuries, large forest areas were destroyed, due to the extension of arable lands into the Nyírség (Frisnyák, Reference Frisnyák2002). At Nyíradony, the surface was burned (Buró et al. Reference Buró, Sipos, Lóki, Andrási, Félegyházi and Négyesi2016) and this soil layer was covered by blown sand. The younger, 150-year-old charcoal can be introduced into the fossil soil as a result of agricultural activity (ploughing).
From the different results, it appears that the sampling locations can be divided into two groups. The first category includes those sections (Gyüre, Lövőpetri, Kántorjánosi, Máriapócs, Petneháza, Vásárosnamény) where the buried soils extend deeper into the profile. In those cases, the LT-SOC 14C ages are in good agreement with the charcoal and Upper-OSL ages. The other group includes those sections (Kótaj) where fossil soils lie shallowly into the profile. In these places, the LT-SOC ages are much younger than the sand cover and charcoal ages above them.
The carbon content of fossil soils can also significantly affect their 14C ages. Low carbon soils (<0.1%) can and do give real ages in the case of LT-SOC fraction (e.g.: Lövőpetri, Máriapócs, Petneháza, and Nyíradony) (Table 2), but they are extremely sensitive to the younger organic matter infiltrating from the overlying layers (Mayer et al. 2001; Zhu et al. Reference Zhu, Cheng, Yu, Yu, Kang, Yang, Jull, Lange and Zhou2010; Cheng and Fu Reference Cheng and Fu2020). This could have happened in Kótaj as well, despite the carbon yield is 0.39%.
Furthermore, soils or fossil soils may be affected by a reservoir effect (Wang et al. Reference Wang, Amundson and Trumbore1996), but this appears to be a small effect in the investigated outcrops. Based on our results, it is not necessary to make any correction for the reservoir effect, as was done by Tóth et al. (Reference Tóth, Prónay, Braun, Nagy, Pethe, Tildy, Buró, Kertész, McIntosh and Molnár2018).
Charred Soil Organic Carbon (HT-SOC) 14C Ages
HT-SOC versus OSL
Comparing the HT-SOC (8.1-25.4 kyr) 14C age with the Upper OSL (8.3–15.2 kyr) data (n=5), the HT-SOC age in three out of five locations is clearly older than the Upper OSL at Gyüre, Lövőpetri and Kántorjánosi, (Figure 2; Table 2). In case of Vásárosnamény, the two age results are similar, within the error, and the HT-SOC 14C age is older than the Upper OSL. The most notable exception is Kótaj, because the HT-SOC younger with 3.2 kyr, not older, than the Upper OSL data.
Comparing the HT-SOC 14C ages (13.0–25.4 kyr) with the Lower OSL (12.3–16.1 kyr) (n=5), it appears that the HT-SOC age is younger in error in case of Gyüre, and clearly younger even in the case of Vásárosnamény and Kótaj (Figures 2 and 3; Table 2). But, in two out of five locations, Lövőpetri and Kántorjánosi, HT-SOC age is unrealistically older (9.8 and 3.4 kyr) than the Lower OSL age of the sand layer under the fossil soil (Figures 2 and 3; Table 2).
Of course, in the case of Kótaj due to the unexpectedly old Upper OSL age, the difference (2.2 kyr) with the HT-SOC age is also unrealistic (Figures 2 and 3; Table 2).
This is because the fact that fossil soil is shallow in the profile (75 cm). At this depth, it can easily occur that younger organic matter (humic acid) moved down the soil profile, recent top soil layer to the fossil soil, rejuvenating the paleosol LH-SOC and HT-SOC ages, despite the fact that the HT-SOC age appears to be correct based on the results.
The HT-SOC age of Vásárosnamény (8.1 kyr) is a little younger (0.2 kyr) than the Upper OSL (8.3 kyr) (Figures 2 and 3; Table 2), and older than the Lower OSL with 6.9 kyr. These three 14C ages were relatively close to each other due to the development of the sand dune. This can be explained by the fact that the previous soil surface was eroded to the buried soil, due to changes in local environmental conditions and then a short period of soil formation took place in this area. This rapid soil formation was followed a sand movement period. The fossil soil was buried by blown sand in the same Atlantic phase (Buró et al. Reference Buró, Lóki, Gyori, Nagy, Molnár and Négyesi2019).
HT-SOC versus Charcoal
For seven of eight cases, the “HT” charred fraction ages (3.4–25.4 kyr) of the soil organic carbon (HT-SOC) (n=8) are older than the charcoal (0.02–14.2 kyr) (Figures 2 and 3; Table 2). However, in case of Kótaj, the charcoal (14.2 kyr) ages are older with 1.2 kyr, than the HT-SOC (13.0 kyr) data. In the case of Kótaj, the difference was already explained previously.
The 14C age of HT-SOC and charcoal is almost the same in the Vásárosnamény profile. This can be explained by a short and rapid soil development period which was mentioned earlier. Here, the soil formation was interrupted by a fire event, which indicated the charcoal pieces and their 14C age.
The paleosol 14C ages determined from the charred fraction of soil organic carbon (i.e., HT-SOC) appear much older and unrealistic than the OSL ages (i.e., Lower OSL) of the underlying sand layer and the charcoal data in some cases. This phenomenon has already been described by Scharpenseel and Becker Heidmann (1992), who showed that old carbon is bound by clay. This phenomenon is particularly striking in case of Lövőpetri and Petneháza, but it also cannot be ruled out at Máriapócs and Petneháza.
Presumably, this clay was derived from older organic matter that formed earlier and transported from another area. The soil profiles are currently affected by wind erosion, which would also have occurred in the past (Borsy Reference Borsy1961; Lóki et al. Reference Lóki, Hertelendi and Borsy1994; Zou et al. Reference Zou, Li, Cheng, Wang, Zhang, Kang, Liu and Zhang2018; Sirjani et al. Reference Sirjani, Sameni, Moosavi, Mahmoodabadi and Laurent2019; Webb et al. Reference Webb, Kachergis, Miller, McCord, Bestelmeyer, Brown, Chappell, Brandon, Herrick, Karl, Leys, Metz, Smarik, Tatarko, Van Zee and Zwicke2020).
CONCLUSION
In this study, our aim was to determine the reliability and limitation of 14C ages of SOC of the paleosol from the Nyírség blown sand area, and we compare these results with independent OSL methods. Our new soil 14C results are in a good agreement with the previously published charcoal age data from the Nyírség. The 14C data show variable agreement with OSL ages. Each sample type should be dated in the following order: Upper OSL, LT-SOC, Charcoal, HT-SOC, Lower OSL but there is exception.
The 14C age of charcoals is reliable and consistent, and we conclude that charcoal is one of the best age markers. Charcoal 14C ages are determined by the time of past fire events, which represent a short period of the soil development time.
The 14C age of the non-charred fraction (LT-SOC) of the Nyírség fossil soils gives a reliable, credible age, despite the low carbon content. This interpretation was confirmed by the charcoal and the independent OSL ages.
In general, our observation is, that LT-SOC ages are younger than the charcoal fragments, in almost every case, except Kótaj, the SOC-LT fraction gave ages only hundreds of years younger (average difference: 700 yr ± 500 yr) (Buró et al. Reference Buró, Lóki, Gyori, Nagy, Molnár and Négyesi2019).
If buried soils do not contain charcoal, macrofossil, or any other macroscopic remnants that would be appropriate for 14C dating, then we can apply the SOC 14C age of the paleosol, but only the low-temperature fraction (i.e., LT-SOC) is significant. The non-charred soil organic carbon (LT-SOC fraction) 14C ages may represent either the burial time or/and the end of development of the fossil soil layer.
However, these data should always be considered carefully, because younger organic matter infiltrating from the topsoil layers into the shallow fossil soils, which is also facilitated by the sandy porous texture of the dunes, can distort the LT-SOC age of fossil soils, as in the case of Kótaj.
The HT charred fraction of the soil organic carbon (SOC) gives variable results compared to charcoal dates and OSL, sometimes unrealistically older than expected. The HT-SOC 14C ages are more hectic and significantly older than charcoal ages, furthermore it has a large standard deviation (average difference: 3300 yr ± 3300 yr). They give good results in some cases, but there are other cases when they are extremely older (e.g., Lövőpetri, Petneháza). Furthermore, in five of the eight locations, the HT carbon content of the samples was very low (<0.05 m/m%). Therefore, the HT-SOC results cannot be considered always useful, HT-SOC 14C ages are not recommended for geochronological study use.
In case of the buried fossil soils of the Nyírség Alluvial fan, it is not necessary to make correction for the reservoir effect, as this phenomenon is not significantly reflected in the results.
Finally, it appears that the combination of OSL and 14C dating methods can be used together to better understand the evolution of aeolian landforms of the Nyírség blown-sand area.
ACKNOWLEDGMENTS
This research was supported by the European Union and the State of Hungary, co-financed by the European Regional Development Fund in the project of GINOP-2.3.2-15-2016-00009 “ICER,” and by the Hungarian National Research, Development and Innovation Office (grant number: K119309, K126065 and K135793).