Cognition refers to the mental process of acquiring knowledge and processing information. It includes functions such as attention, memory, problem solving, decision making, planning, inhibition, judgement and evaluation, reasoning, comprehension and production of language and orientation/visuospatial skills. Dementia is a general term for a loss of one or more of these functions that is severe enough to interfere with daily life. There are now over 100 recognised forms of dementia, with Alzheimer's disease (AD) being the most prevalent, and responsible for about two-thirds of dementia cases.
Globally, there are 50 million living with dementia(Reference Patterson1), occurring in 5–8 % of those aged over 60 years, with the prevalence increasing exponentially with age(2, Reference Prince, Knapp and Guerchet3) (Fig. 1).
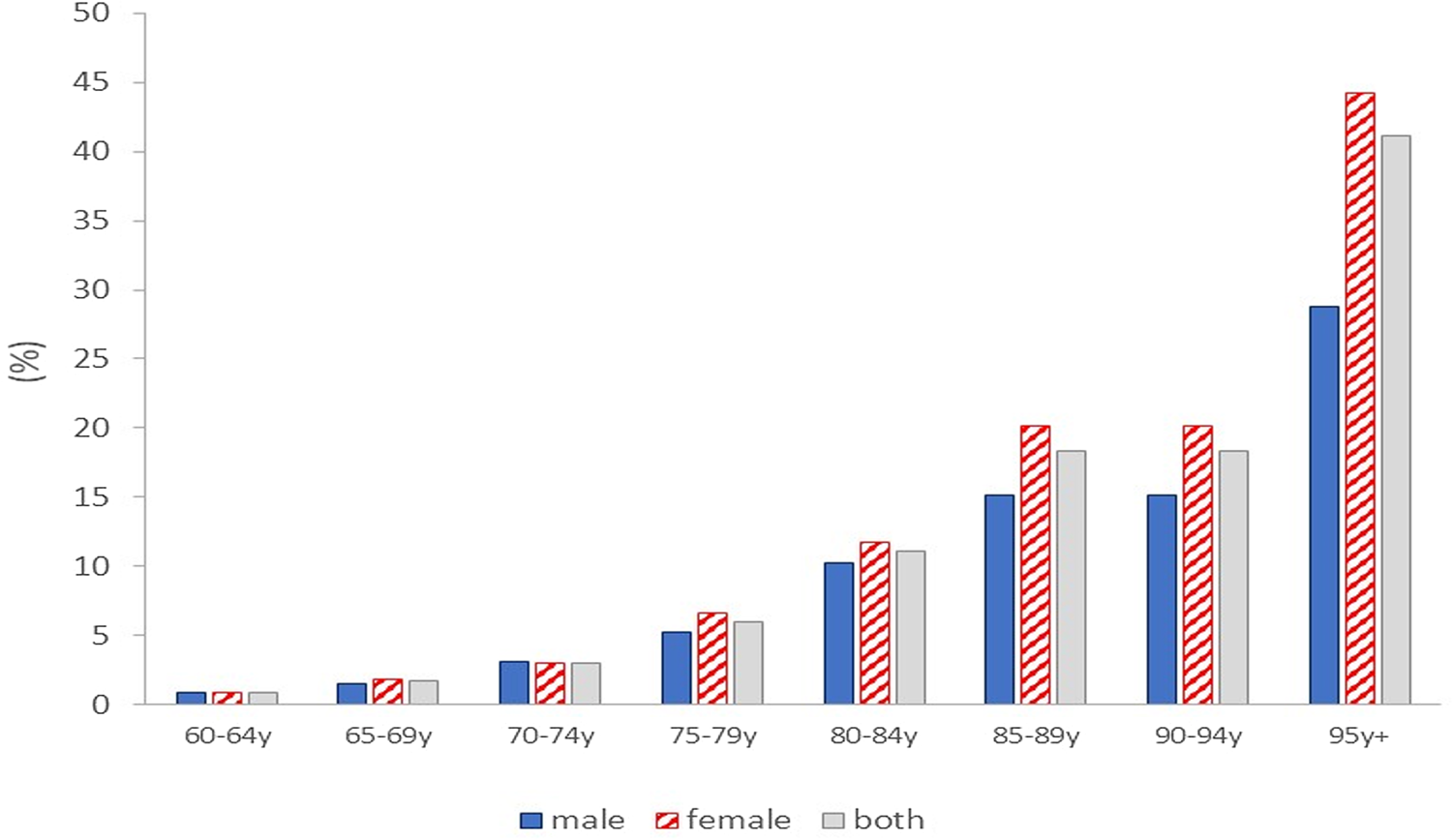
Fig. 1. Dementia prevalence (% of the population) by age group in the UK(Reference Prince, Knapp and Guerchet3).
With growing and ageing populations, and more widespread diagnostic services, diagnosed dementia rates are predicted to triple by 2050(Reference Patterson1). Dementia is the second leading cause of death globally after IHD(2) and in England and Wales dementia is now the single greatest cause of death in women, responsible for 16⋅5 % of total mortality (v. 8⋅7 % in men)(4). This sex difference in dementia-associated death rates is reflective of the fact that two-thirds of dementia patients are females(2), the physiological and molecular basis of which is only partially elucidated(Reference Ferretti, Iulita and Cavedo5–Reference Oveisgharan, Arvanitakis and Yu7). Accelerated neuropathology and cognitive decline, evident during the menopausal transition in females, and the higher penetrance of the at-risk APOE4 allele in female carriers are likely to be major contributing factors(Reference Neu, Pa and Kukull8–Reference Rahman, Schelbaum and Hoffman11).
However, encouragingly age-standardised rates are decreasing in many high-income countries. Between 1990 and 2016, a 6⋅8, 10⋅3 and 8⋅4 % reduction in dementia-associated death, prevalence and disability-adjusted life years rates, respectively, was observed in the UK(2). These reductions have been attributed to greater education attainment (creating cognitive reserve), better cardiovascular health and improved nutrition.
Overview of interventions for dementia treatment and prevention
There are currently few effective drugs to prevent or treat dementias. In the UK, there are four licensed drugs available (donepezil, rivastigmine, galantamine and memantine) which temporarily treat symptoms by targeting synaptic function and neurotransmission. In 2021, the Food and Drug Administration granted accelerated approval to aducanumab, the first drug in 18 years for AD(Reference Walsh, Merrick and Milne12). It is a monoclonal antibody which targets amyloid clearance and is currently undergoing regulatory review in Europe. Its purported efficacy is controversial with the benefits thought to be marginal in most patients(Reference Walsh, Merrick and Milne12).
In the absence of effective pharmaceutical options to prevent, reverse or treat dementia, there is a widespread interest in lifestyle behaviour approaches including nutrition to prevent or delay neurophysiological and cognitive decline. In the 2020 Dementia prevention, intervention, and care: 2020 report of the Lancet Commission, it was estimated ‘that 12 modifiable risk factors account for around 40% of worldwide dementias, which consequently could theoretically be prevented or delayed’(Reference Livingston, Huntley and Sommerlad13). Many of these are nutrition-dependent (hypertension, obesity, diabetes, depression and recovery from traumatic brain injury) and likely mediate the emerging role of nutrition in brain health.
Research into the role of nutrition in age-related cognitive decline is in its relative infancy compared with other chronic conditions such as CVD and osteoporosis, with research evidence largely derived from prospective cohort studies or experimental models.
Although not fully consistent, a growing body of prospective cohort evidence shows that plant-based dietary patterns and individual dietary bioactives such as selenium, vitamin D, B-vitamins, polyphenols and long chain n-3 fatty acids (LC n-3 PUFA) improve cognition and reduce dementia risk, conversion of mild cognitive impairment to AD and brain atrophy(Reference Solfrizzi, Custodero and Lozupone14–Reference Jennings, Cunnane and Minihane20). A Mediterranean dietary pattern and the Mediterranean-Dietary Approaches to Stop Hypertension Diet Intervention for Neurodegenerative Delay diet have emerged as particularly effective with high v. low adherence associated with up to a 40 % reduced dementia rate(17,Reference Melo van Lent, O'Donnell and Beiser21–Reference Wu and Sun23) . The potential of the protective role of a Mediterranean dietary pattern was highlighted in the 2017 Lancet Commission Dementia prevention, intervention, and care report(Reference Livingston, Sommerlad and Orgeta24), with a Mediterranean dietary pattern being the only specific dietary approach for which the WHO 2019 Risk Reduction of Cognitive Decline and Dementia guidelines(25) recommended to adults with normal cognition to reduce the risk of cognitive decline and dementia. A defining component of a Mediterranean dietary pattern is a high fish and n-3 fatty acid intake. Here, we focus on the role of the LC n-3 PUFA DHA in brain function and on evidence for a protective role of higher fish and DHA and EPA intake and status in cognitive health. We critique the apparent inconsistencies between the protective associations observed in prospective cohort and biological effects in experimental models v. the inconsistent and often null or marginal effects seen in randomised controlled trials (RCTs).
Fatty acid uptake into the brain
DHA is a 22-carbon n-3 fatty acid, with multifaceted structural and functional roles in the central nervous system. Although DHA can be synthesised in the liver from the plant precursor n-3 fatty acid α-linolenic acid, bioconversion is less than 0⋅2 %(Reference Burdge, Finnegan and Minihane26). Within the brain, the synthesis of DHA from α-linolenic acid is negligible. Therefore, DHA uptake via the highly selective blood–brain barrier (BBB) is required to replace the DHA consumed in metabolic reactions. A dietary supply of DHA as either oily fish or supplements is recommended to meaningfully enrich brain levels. DHA is the predominant PUFA in the brain, accounting for 15 % of total fatty acids which is several fold higher than most other tissues such as the heart and the liver where it constitutes about 2 %(Reference Arterburn, Hall and Oken27). Grey matter, including synaptic membranes, synaptic vesicles and mitochondria, are particularly enriched(Reference Crawford, Casperd and Sinclair28).
The BBB is formed of tightly connected endothelial cells, embedded within a network of pericytes and astrocytes foot processes that support its function(Reference Zhao, Nelson and Betsholtz29). Fatty acids cross the BBB by two known mechanisms, either facilitated transport by several transmembrane proteins or by passive diffusion(Reference Bazinet and Layé30). BBB uptake of plasma fatty acids was historically thought to be only from NEFAs, which originate from lipoproteins or are bound to plasma proteins, mainly albumin(Reference Lacombe, Chouinard-Watkins and Bazinet31). More than 99 % of non-esterified-DHA is protein bound. NEFAs are transported through the endothelial cell membranes and cytoplasm via a group of fatty acid transport proteins (FATP) and fatty acid binding proteins. FATP1 and FATP4 are highly expressed in both the vascular and the parenchymal regions of the brain(Reference Mitchell, On and Del Bigio32). Recent studies show that FATP1 participates in 60 % of DHA uptake(Reference Ochiai, Uchida and Ohtsuki33). Interestingly, in a cell culture model, amyloid-β (Aβ), the hallmark of AD pathology, induced a 96 % reduction in FATP1 protein expression and an associated 45 % reduction in DHA efflux(Reference Ochiai, Uchida and Tachikawa34). More recently, acyl-CoA synthetase 6 (Acsl6) has been identified as an essential transporter for enriching the brain with DHA(Reference Chouinard-Watkins and Bazinet35,Reference Fernandez, Kim and Zhao36) .
Besides the NEFA form, DHA is also taken up into the brain in the form of lysophosphatidylcholine (LPC-DHA)(Reference Chan, Wong and Chin37). The major facilitator superfamily domain-containing protein 2a (MFSD2A) is considered the major route of LPC-DHA uptake(Reference Nguyen, Ma and Shui38–Reference Wong, Silver and Jiang40). Indeed, Mfsd2a knockout mice showed 50 % lower brain DHA levels compared to wild-type animals, with consequent cognitive deficits, anxiety and microcephaly(Reference Nguyen, Ma and Shui38). Along with its emerging role in LPC fatty acid transport and the regulation of BBB permeability(Reference Ben-Zvi, Lacoste and Kur41), MFSD2A is emerging as having more far-reaching functions in neuroinflammation and other physiological and pathophysiological brain processes(Reference Eser Ocak, Ocak and Sherchan42). Overall, although the uptake and partitioning coefficient is higher for LPC-DHA relative to non-esterified-DHA following intravenous injections, non-esterified-DHA is thought to be the main source of DHA for the brain due to its higher circulating concentrations(Reference Lacombe, Chouinard-Watkins and Bazinet31,Reference Chouinard-Watkins, Lacombe and Bazinet43) .
Although currently largely unknown, it is emerging that variables such as age, menopause, neuropathology and APOE genotype status(Reference Yassine, Braskie and Mack44), may impact the brain DHA uptake processes, and has implications for the recommended DHA dose in population subgroups, and the optimal intervention ‘window of opportunity’ when DHA supplementation is most likely to bring about cognitive benefits. Furthermore, defective brain DHA uptake could underpin the lack of benefit of DHA observed in several RCTs, particularly in APOE4 carriers (see section ‘Fish and DHA intake and status and cognition: randomised controlled trial evidence’).
APOE genotype: impact on dementia/Alzheimer's disease risk and age of onset
ApoE, produced mainly in the brain by glial cells, is the principal lipid transporter within the brain and cerebrospinal fluid, but also has numerous other roles in neuroinflammation and neuronal function. Two missense mutations in APOE gene (rs429358 and rs7412) produce three allele variants: ε2, ε3 and ε4. These alleles have different amino acids (cysteine or arginine) in positions 112 and 158, resulting in ApoE2 (Cys112 and Cys158), ApoE3 (Cys112 and Arg158) and ApoE4 (Arg112 and Arg158)(Reference Zhong and Weisgraber45). These amino acid differences lead to conformational changes in ApoE structure which affects binding to lipoprotein receptors and also the stability and tissue concentrations of the protein(Reference Frieden and Garai46). The global frequencies of the ε2, ε3 and ε4 alleles are approximately 8⋅4, 77⋅9 and 13⋅7 %, respectively(Reference Abondio, Sazzini and Garagnani47). APOE genotype is the most important common genetic determinant of cognitive decline and AD risk, with a 3-fold increased prevalence of the ε4 allele in AD v. the general population and the APOE3/E4 and APOE4/E4 genotypes having a 2–3- and 12–15-fold increased risk of AD compared to the wild-type APOE3/E3 genotype(Reference Liu, Liu and Kanekiyo48,Reference Farrer, Cupples and Haines49) . In addition, APOE4 is associated with an average lower age of AD onset(Reference Farrer, Cupples and Haines49). It falls from 84 years in APOE4 non-carriers to 76 years in APOE3/E4 to 68 years in APOE4/E4 (Reference Farrer, Cupples and Haines49,Reference Corder, Saunders and Strittmatter50) . The aetiology of the increased risk in APOE4 carriers is multi-faceted and can be attributed to defective Aβ clearance, a loss of neuronal synaptic plasticity and dendrite outgrowth, neuroinflammation, cerebrovascular and BBB dysfunction and lower brain DHA status(Reference Yassine and Finch51).
In a transgenic rodent model, the uptake of [14C]-DHA using in situ cerebral perfusion was significantly lower in APOE4 v. APOE2 animals, which was exacerbated by age(Reference Vandal, Alata and Tremblay52). This observation of a greater effect of age on brain DHA is consistent with our more recent rodent studies, where the effect of age was more evident in females(Reference Martinsen, Tejera and Vauzour53) and following induction of menopause(Reference Pontifex, Martinsen and Saleh10). In human subjects, DHA supplementation resulted in lower circulating DHA levels(Reference Chouinard-Watkins, Conway and Minihane54,Reference Chouinard-Watkins, Rioux-Perreault and Fortier55) , higher systemic β-oxidation(Reference Chouinard-Watkins, Rioux-Perreault and Fortier55) and lower cerebrospinal fluid DHA following 18 months of supplementation(Reference Yassine, Rawat and Mack56). Defective BBB transfer, brain lipid transport and increased oxidation of DHA following upregulated release by PhospholipaseA2, are all likely contributors to a lower DHA brain status in APOE4 (Reference Yassine, Braskie and Mack44). As will be discussed later, APOE genotype has also emerged as an important mediator of the effect of DHA status and intervention on incident dementia and cognitive outcomes, but the effect is inconsistent and likely to dependent on age, sex and brain health stage.
The role of DHA in the brain
Since first being identified in the brain by Klenk and Bongard in 1952(Reference Klenk and Bongard57), many neurophysiological roles have been identified for DHA in experimental models, including membrane structural roles (fluidity and modulation of membrane protein function) and the modulation of neurogenesis and neuronal cell growth and cell survival, Aβ clearance, vascular function and brain perfusion, BBB permeability, oxidative status, neuroinflammation, synaptic function and neurotransmission(Reference Pontifex, Vauzour and Minihane9,Reference Lacombe, Chouinard-Watkins and Bazinet31,Reference Belayev, Hong and Menghani58–Reference Weiser, Butt and Mohajeri66) . Synaptosomal membranes are particularly enriched in DHA, where it constitutes up to 40 % of PUFA in select lipid species and modulates neurotransmitter levels and membrane dynamics(Reference Sinclair67). Loss of synaptic plasticity is a major contributor to the pathogenesis of cognitive decline, mediated in part through reduced levels of brain-derived neurotrophic factor and its related signalling pathways(Reference Kowiański, Lietzau and Czuba68). DHA is known to increase the level of brain-derived neurotrophic factor and consequently activates protein kinase B (AkT) and extracellular signal-regulated kinase signalling pathways leading to improved synaptic plasticity(Reference Wu, Ying and Gomez-Pinilla69). Reduced recognition memory was evident in menopausal APOE4 mice models fed with a high-fat diet(Reference Pontifex, Martinsen and Saleh10). This memory deficit was associated with a 13 % reduction in cortical DHA, reduced brain-derived neurotrophic factor expression and compromised Akt, mammalian target of rapamycin and extracellular signal-regulated kinase signalling pathways, highlighting the mechanistic role of DHA, interacting with menopause and APOE4, in cognitive decline via modulation of synaptic plasticity-related pathways(Reference Pontifex, Martinsen and Saleh10).
A systematic review on the effects of relatively long-term n-3 intervention in animal AD models included data from 15 studies and reported significant reductions in amyloid levels, plaque burden and neuronal loss and improved cognition following DHA only or EPA + DHA supplementation(Reference Hooijmans, Pasker-de Jong and de Vries70).
Once released from membrane phospholipids via phospholipase A2, DHA regulates inflammation through the modulation of cytokine production and as a precursor for a host of bioactive oxylipins(Reference Bazinet and Layé30,Reference Chataigner, Martin and Lucas71–Reference Song, Manku and Horrobin75) (see the next section).
Neuroinflammation, oxylipins and brain health
Amyloid plaque deposition is one of the hall mark of AD pathology. Risk factors such as APOE4 carrier status, vascular pathologies and neuroinflammation play interactive roles in the cascade of synthesis of Aβ and the progression of cognitive decline(Reference Devassy, Leng and Gabbs76). Indeed, proinflammatory cytokines such as IL6 and TNFα are increased in the blood and brain of patients with AD(Reference Fillit, Ding and Buee77,Reference Strauss, Bauer and Ganter78) . Brain microglia, the brain-resident immune cells, are the major regulator of brain inflammatory status via the release of inflammatory cytokines such as IL1β, TNFα and inducible nitric oxide synthase(Reference Liu and Hong79). Activated microglia surround amyloid plaques in the cerebral cortex of AD patients, which suggests that Aβ deposition can trigger microglial activation and subsequent release of inflammatory cytokines(Reference Sastre, Klockgether and Heneka80,Reference Wyss-Coray81) .
However, recent studies suggest that neuroinflammation also plays an Aβ-independent role in the pathogenesis of cognitive decline(Reference Calsolaro and Edison82,Reference Cai, Hussain and Yan83) . Imaging studies have observed microglial activation in patients with mild cognitive impairment even before the appearance of amyloid deposits(Reference Dani, Wood and Mizoguchi84,Reference Chandra, Valkimadi and Pagano85) which increases with disease progression(Reference Fan, Okello and Brooks86,Reference Jack, Knopman and Jagust87) .
PUFAs have been extensively studied as a modulator of systemic inflammation in chronic diseases such as atherosclerosis, diabetes and rheumatoid arthritis. These conditions are consistently associated with higher C-reactive proteins (CRPs), TNFs, IL6, thromboxane A2 (TXA2) and leucotrienes B4 (LTB4)(Reference Dinarello88,Reference Calder89) , which are affected by tissue PUFA status(Reference Simopoulos90) (Fig. 2). Similar to its systemic anti-inflammatory role, n-3 PUFAs are considered effective modulators of the brain inflammatory status(Reference Orr, Trépanier and Bazinet91,Reference Layé, Nadjar and Joffre92) . Higher DHA intake was associated with lower IL6 in the mouse hippocampus(Reference Fourrier, Remus-Borel and Greenhalgh93) and inhibition of the NF-κβ inflammatory pathway(Reference Chen, Chen and Fan94). DHA reduced Aβ deposition in an AD mouse model(Reference Hur, Mateo and Amalric62) through the reduction of the IL12/IL23 signalling pathway(Reference Vom Berg, Prokop and Miller95).
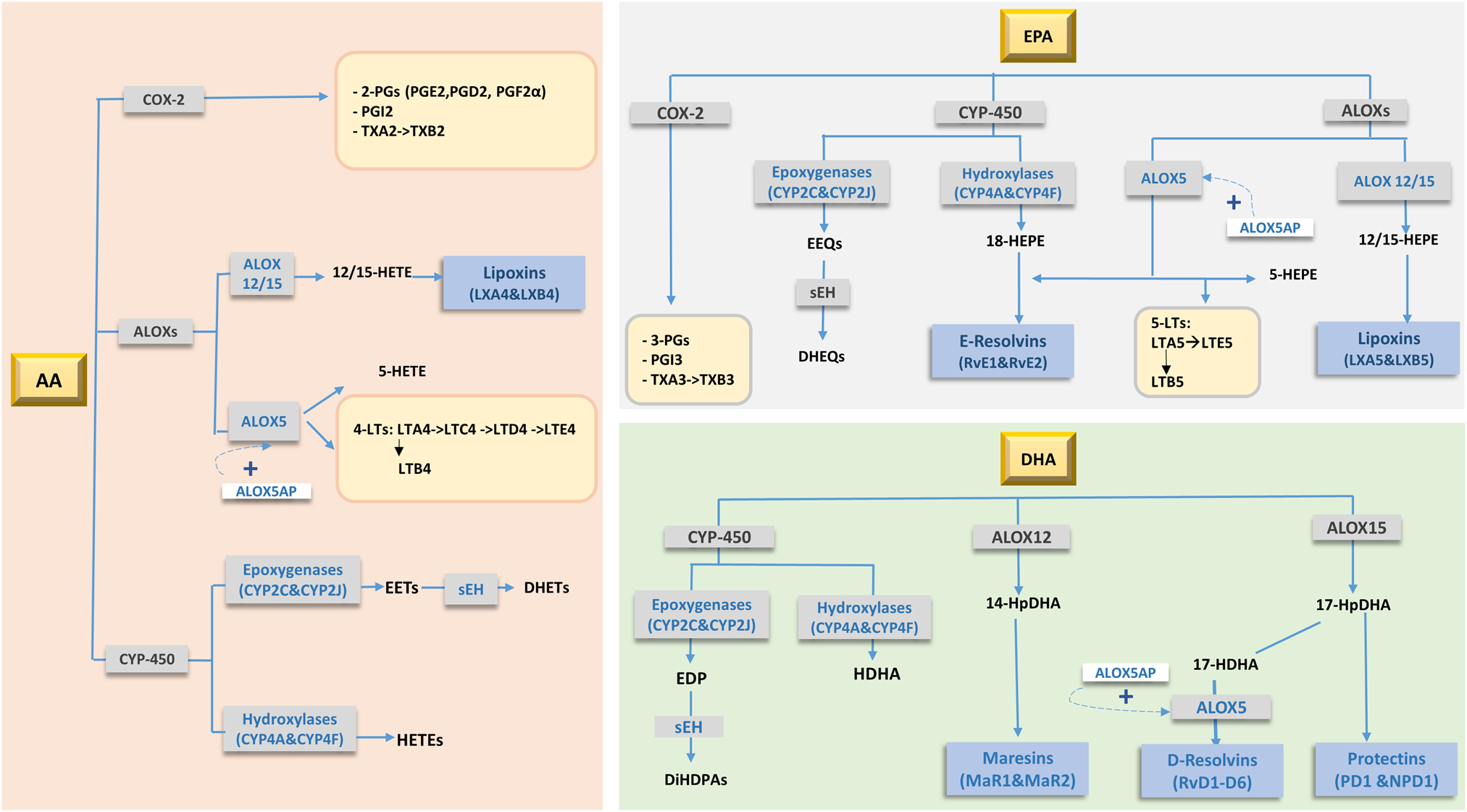
Fig. 2. Main oxidative products (oxylipins) of arachidonic acid (AA), EPA and DHA metabolism. Adapted from Schulze et al.(Reference Schulze, Minihane and Saleh97). 2-PGs, 2 series prostaglandins; 3-PGs, 3 series prostaglandins; 4-LTs, 4 series leucotrienes; 5-LTs, 5 series leucotrienes; ALOXs, arachidonate Lipoxygenases; ALOX5AP, 5-lipoxygenase activating protein; COX-2, cyclooxygenase-2; CYP-450, cytochrome-P450; DHEQs, dihydroxyeicosatetraenoic acids; DHET, dihydroxyeicosatrienoic acid; DiHDPA, dihydroxydocosapentaenoic acid; EDP, epoxydocosapentaenoic acid; EEQ, epoxyeicosatetraenoic acid; EETs, epoxyeicosatrienoic acid; HDHA, hydroxydocosahexaenoic acid; HEPE, hydroxyeicosapentaenoic acid; HETE, hydroxy-eicosatetraenoic acid; HpDHA, hydroperoxide intermediate of DHA; sEH, serum epoxide hydrolase enzyme; TX, thromboxanes.
The biological actions of PUFAs in controlling neuroinflammation are in part mediated through their enzymatically and non-enzymatically oxidised metabolites, called oxylipins(Reference Gabbs, Leng and Devassy96) (Fig. 2). These lipid-derived oxygenated metabolites of PUFA are synthesised by three groups of enzymes: cyclooxygenases, lipoxygenases and cytochrome P450 enzymes(Reference Schulze, Minihane and Saleh97), which produce hydroxy-, dihydroxy- or epoxy-fatty acids. Due to their highly unsaturated nature, PUFAs are also non-enzymatically oxidised (i.e. autooxidation) by reactive oxygen and nitrogen species(Reference Anderson and Taylor98). Oxylipins are precursors for specialised pro-resolving mediators (SPMs; resolvins, protectins, maresins and lipoxins) which have anti-inflammatory and pro-resolving roles(Reference Buckley, Gilroy and Serhan99). n-6 PUFA-derived oxylipins are generally pro-inflammatory relative to EPA/DHA-derived species. The enzymatic action of cyclooxygenases on AA produces the pro-inflammatory PGH2, TXA2 and 5-, 12- and 15-hydroxy-eicosatetraenoic acid (HETEs)(Reference Christie and Harwood100). It is worth mentioning that AA is also a precursor of the anti-inflammatory and pro-resolving lipoxins A4 and B4(Reference Gabbs, Leng and Devassy96).
n-3 PUFA-derived oxylipins generally have anti-inflammatory and pro-resolving properties. EPA produces less inflammatory PGs, TXAs (3-series) and LTs (5-series)(Reference Wada, DeLong and Hong101) relative to AA-derived oxylipins. Epoxy-EPA oxylipins produce anti-inflammatory responses(Reference Kodani and Hammock102) partly through the inhibition of NF-κβ pathway and through antagonising inflammation induced by PGE2(Reference Node, Huo and Ruan103,Reference Inceoglu, Wagner and Schebb104) . RvE1 reduces proinflammatory cytokine production(Reference Seki, Fukunaga and Arita105), neutrophil infiltration and reduces proinflammatory gene expression in peripheral blood mononuclear cells and microglia through binding to ChemR23 receptor(Reference Herová, Schmid and Gemperle106,Reference Arita, Bianchini and Aliberti107) .
The role of oxylipins and SPMs in the protection against neuroinflammation and the development of AD is gaining research attention(Reference Joffre, Dinel and Chataigner73,Reference Devassy, Leng and Gabbs76,Reference Miyazawa, Fukunaga and Tatewaki108) . The resolution of the inflammatory process is disrupted with ageing and cognitive decline(Reference Whittington, Planel and Terrando109). In murine models, increased n-6 PUFA-derived oxylipins and decreased n-3-derived oxylipins and SPMs are generally observed in neuroinflammatory brain disorders(Reference Devassy, Leng and Gabbs76). A reduction in cortical 14- and 17-hydroxydocosahexaenoic acid and hippocampal neuroprotectin D1 with age was observed(Reference Martinsen, Tejera and Vauzour53). Brain LXA4 was also found to decline with age, with the reduction more pronounced in an AD-mouse model(Reference Dunn, Ager and Baglietto-Vargas110). Interestingly, administration of LXA4 in this AD-mouse model(Reference Dunn, Ager and Baglietto-Vargas110) and RvD1 in a post-operative cognitive impairment model(Reference Terrando, Gómez-Galán and Yang111) reduced cognitive decline under both conditions. Protectin D1 was first detected in murine blood and neuroprotectin D1 is present in the brain(Reference Hong, Gronert and Devchand112). Neuroprotectin D1 levels greatly increased in the hippocampus after lipopolysaccharide stimulation(Reference Orr, Palumbo and Bosetti74). It binds to the GPR37 receptor(Reference Qu and Caterina113) to inhibit NF-κβ and pro-inflammatory gene expression(Reference Marcheselli, Hong and Lukiw114). Neuroprotectin D1 showed protective function in neurodegeneration through modulating synaptic plasticity and microglial activity(Reference Asatryan and Bazan115). In human studies, LXA4 was lower in patients with AD compared to mild cognitive impairment or subjective cognitive impairment patients. Similarly, LXA4 and MaR1 were reduced in postmortem hippocampi of AD patients compared to controls, whereas the n-6 PUFA oxylipins 5-HETE, 15-HETE, TXB2 and PGs increased(Reference Wang, Zhu and Hjorth116).
Being precursors to oxylipins and SPMs, several studies have explored the potential benefits of n-3 PUFA in preventing cognitive decline via modulating the levels of brain oxylipins and SPMs. In aged rats, EPA and DHA supplementation increased cortical 5-HEPE, 7-, 10-, and 17-hydroxydocosahexaenoic acid, PD1, RvD1 and RvD2(Reference Hashimoto, Katakura and Tanabe117). AA-derived PGE2, PGD2 and PGF2α significantly decreased with an associated improvement in reference memory. In response to lipopolysaccharide stimulation, n-3 PUFA supplemented mice showed an increase in hippocampal n-3 oxylipins compared to non-supplemented mice who showed an increase in the n-6 pro-inflammatory oxylipins(Reference Rey, Delpech and Madore118). In AD patients, EPA and DHA supplementation increased peripheral blood mononuclear cell RvD1 levels compared to controls(Reference Wang, Zhu and Hjorth116).
Significant inter-individual variability in the response of oxylipins to n-3 PUFA supplementation has been reported(Reference Ostermann and Schebb119). In mice, select EPA- and DHA-derived brain oxylipins and SPMs were lower in APOE4 compared to APOE3 (Reference Martinsen, Tejera and Vauzour53). In addition, the plasma oxylipins response to EPA + DHA supplementation was influenced by APOE genotype in healthy individuals with a greater production of a number of EPA- and DHA-derived species in APOE4 carriers(Reference Saleh RN, Ostermann and Schebb120). Genetic variation in enzymes involved in PUFA metabolism has been implicated as a possible modulator of oxylipin production from PUFAs. Genetic variation in LTA4H, an enzyme in the pathway of leucotriene synthesis, significantly interacted with dietary n-3 and n-6 fatty acid intake to determine intima-media thickness(Reference Zhao, Roman and Devereux121). Variants in ALOX5 gene were associated with a differential oxylipin response to fish oil supplementation in healthy African American adults(Reference Stephensen, Armstrong and Newman122).
Given the central role of neuroinflammation in cognitive decline, the modulation of cytokine, oxylipin and SPM production is a tractable target to prevent and delay neuropathology by increasing EPA and DHA status and intake.
Fish and DHA intake and status and cognition: prospective cohort evidence
There is a substantial and a relatively consistent body of research from prospective cohorts, of an inverse association between fish and EPA and DHA intake and status (measured in a number of blood lipid fractions), and dementia and AD risk, brain atrophy and cognitive decline. In the earliest report from the Rotterdam Cohort study (n 5386), with an incident case rate of 1⋅1 % (n 58) over 2⋅1 years, total fat, saturated fat and fish intake were inversely related to incident dementia(Reference Kalmijn, Launer and Ott123). However, in the 9⋅6 years follow-up of this cohort, with 465 dementia cases, total fish, EPA or DHA intake was not associated with either total dementia or AD risk(Reference Devore, Grodstein and van Rooij124). This lack of association is in contrast to the findings of the largest prospective analysis conducted to date on fish, n-3 fatty acids and dementia, namely the NIH-AARP study in 421 309 adults followed up for 16 years, with 85 112 deaths(Reference Zhang, Zhuang and He125). Quintile 5 (Q5) v. Q1 of total fish intake was associated with a hazard ratio (HR) of AD death of 0⋅76 (95 % CI: 0⋅61, 0⋅95) with an even stronger association evident when fried fish was removed. Considering LC n-3 PUFA intake, an HR of AD death of 0⋅70 (95 % CI: 0⋅54, 0⋅89) was observed in Q5 v. Q1 in males, with an even greater benefit in females (HR: 0⋅59 (95 % CI: 0⋅43, 0⋅80))(Reference Zhang, Zhuang and He125). Q5 represented a mean intake of >180 mg and 160 mg daily in males and females of LC n-3 PUFAs, mainly EPA + DHA. This intake is modest compared to the typical UK and global recommended intakes of 450–500 mg daily EPA + DHA minimum recommended intake(126,127) , which is mainly targeted towards cardiovascular health.
In an analysis of post-mortem brains, seafood consumption (≥1 meal(s)/week), measured on average 4⋅5 years before death was correlated with less AD pathology including lower neuritic plaques, less severe and widespread neurofibrillary tangles, and lower neuropathologically defined AD but only among APOE ε4 carriers(Reference Morris, Brockman and Schneider128).
A number of analyses have reported positive associations between DHA or EPA + DHA status in blood lipids fractions and cognitive outcomes(Reference Schaefer, Bongard and Beiser129–Reference von Schacky131). In the Framingham Cohort, high v. low (Q4 v. Q1) phosphatidylcholine-DHA was associated with a 47 % reduction in all cause dementia(Reference Schaefer, Bongard and Beiser129). In the Women's Health Initiative Memory Study, the HR of probable dementia in the 9⋅8 years follow-up was 0⋅92 (95 %: 0⋅84, 1⋅00) per sd of erythrocyte EPA + DHA (n-3 index) with a similar HR when EPA and DHA were considered separately(Reference Ammann, Pottala and Robinson130). The 15-year cumulative incidence of probable dementia was estimated to be 12⋅1 % with high EPA + DHA exposure compared to 14⋅2 % with low EPA + DHA exposure (absolute risk difference = 2⋅05 %).
Ageing and dementia progression are underpinned by total brain atrophy (loss of volume) and in AD the hippocampus is particularly affected. In the Women's Health Initiative Memory study, a 1 sd greater erythrocyte EPA + DHA level was correlated with 2⋅1 cm3 larger brain volume and greater hippocampal volume (50 mm3), with the effect size purported to be equivalent to 1–2 years of ageing(Reference Pottala, Yaffe and Robinson16). An association between erythrocyte EPA + DHA and medial temporal lobe volume trajectories assessed over a maximum of 10⋅8 years (median follow-up 4⋅0 years) was observed in the Three-City study, along with improved global cognition and memory and a 60 % increased risk of dementia in Q1 v. Q5 of EPA + DHA status(Reference Thomas, Baillet and Proust-Lima132).
The findings from prospective cohort studies have been synthesised into four meta-analyses which focus on fish intake(Reference Bakre, Chen and Khutan133,Reference Samieri, Morris and Bennett134) , or both fish and LC n-3 PUFA intake(Reference Wu, Ding and Wu135,Reference Zhang, Chen and Qiu136) on a variety of cognitive outcomes, which are further summarised in an umbrella review of meta-analyses(Reference Barbaresko, Lellmann and Schmidt15). Samieri et al. pooled the French Three-City study and four US cohorts and included data from n 23 688 (88 % female) with median follow-ups of 3⋅9–9⋅1 years(Reference Samieri, Morris and Bennett134). Higher fish intake was associated with slower decline in both global cognition and episodic memory. The effect of consuming ≥4 servings/week v. <1 serving/week of fish on episodic memory decline was estimated to be equivalent to 4 years of ageing. Although the Bakre et al.'s (n 9 studies) analysis does not provide information on actual fish portion consumption per category, a dose-dependent effect was observed with a relative risk (RR) (95 % CI) of dementia of 0⋅84 (0⋅72, 0⋅98), 0⋅78 (0⋅68, 0⋅90) and 0⋅77 (0⋅61, 0⋅98) in those with low, middle and high consumption of fish v. those with no or lowest consumption of fish, with the corresponding RRs of 0⋅88 (0⋅74, 1⋅04), 0⋅79 (0⋅65, 0⋅96) and 0⋅67 (0⋅58, 0⋅78), respectively for AD(Reference Bakre, Chen and Khutan133). In the most comprehensive and granular meta-analysis, Zhang et al. combine data from twenty-one individual studies (181 580 participants) with 4438 cases, during follow-up periods ranging from 2⋅1 to 21 years to examine associations between fish, total PUFA and individual PUFA intakes and total dementia and dementia sub-types(Reference Zhang, Chen and Qiu136). The main findings is that an increase in fish of one serving per week is associated with a lower RR (95 % CI) of dementia and AD of 0⋅95 (0⋅90, 0⋅99) and 0⋅93 (0⋅90, 0⋅95), with an equivalent RR for a 0⋅1 g/d increment of dietary DHA intake (but not EPA) of 0⋅86 (0⋅76, 0⋅96) and 0⋅63 (0⋅51, 0⋅76), respectively. This effect size for one portion of fish and AD is relatively consistent with Wu et al.'s analysis who reported that an increment of 100 g/week of fish intake (UK portion is 140 g) was associated with an 11 % lower risk of AD (RR = 0⋅89, 95 % CI 0⋅79, 0⋅99)(Reference Wu, Ding and Wu135).
There is conflicting evidence that associations may be influenced by APOE genotype status, with some prospective cohorts reporting no influence(Reference Ammann, Pottala and Robinson130,Reference Thomas, Baillet and Proust-Lima132,Reference Samieri, Morris and Bennett134) , some no benefits of fish or EPA/DHA intake in APOE4 carriers(Reference Barberger-Gateau, Samieri and Féart137–Reference Whalley, Deary and Starr139) and some reporting a beneficial association only in APOE4 (Reference Morris, Brockman and Schneider128). These apparent inconsistencies are likely attributable to a lack of a granular understanding of influencers of brain DHA metabolism in APOE4. It is possible that due to a defective brain DHA uptake and metabolism there is a greater DHA need throughout life in APOE4. However, with variables such as age, menopause and significant pathology potentially having a greater impact on brain DHA uptake in APOE4, beyond a certain physiological stage an increased DHA intake or blood status may have a lower or negligible cognitive benefit in APOE4 as it will not translate into higher brain DHA levels. More research is needed to identify the optimal DHA intake and supplementation ‘window’ in APOE4.
Therefore overall, in prospective cohort studies high v. low/no fish and LC n-3 PUFA consumption is associated with up to a 40 % reduced risk of total dementia, and in particular AD, with effect sizes equivalent to several years of ageing. It is likely the benefits of fish consumption extend beyond the provision of LC n-3 PUFAs, with fish also being a rich sources of selenium, vitamin B12 and vitamin D, all of which may enhance cognition(Reference Scarmeas, Anastasiou and Yannakoulia18). For a dietary component such as DHA/fish which is considered a signature of affluence and an overall healthy diet and lifestyle(Reference Thomas, Baillet and Proust-Lima132) the possibility of residual confounding should be considered, with some of the cognitive benefits seen in prospective cohorts, potentially due to as yet unknown factors which are not fully corrected for in the statistical models.
Fish and DHA intake and status and cognition: randomised controlled trial evidence
To the best of our knowledge, there is currently no RCT which has investigated the impact of fish intake in isolation (i.e. not as part of a multi-food or whole diet intervention) on cognitive outcomes. Prospective cohort evidence where EPA and DHA are predominantly derived from fish, have examined the impact on dementia risk and cognition over follow-up periods up to 20 years. In contrast, RCTs have intervened with a mixed LC n-3 PUFA or DHA-rich supplement for up to 3 years, but typically 6 months, which have produced mixed and often null findings (Table 1).
Table 1. Select RCTs of nutrition interventions to improve cognition
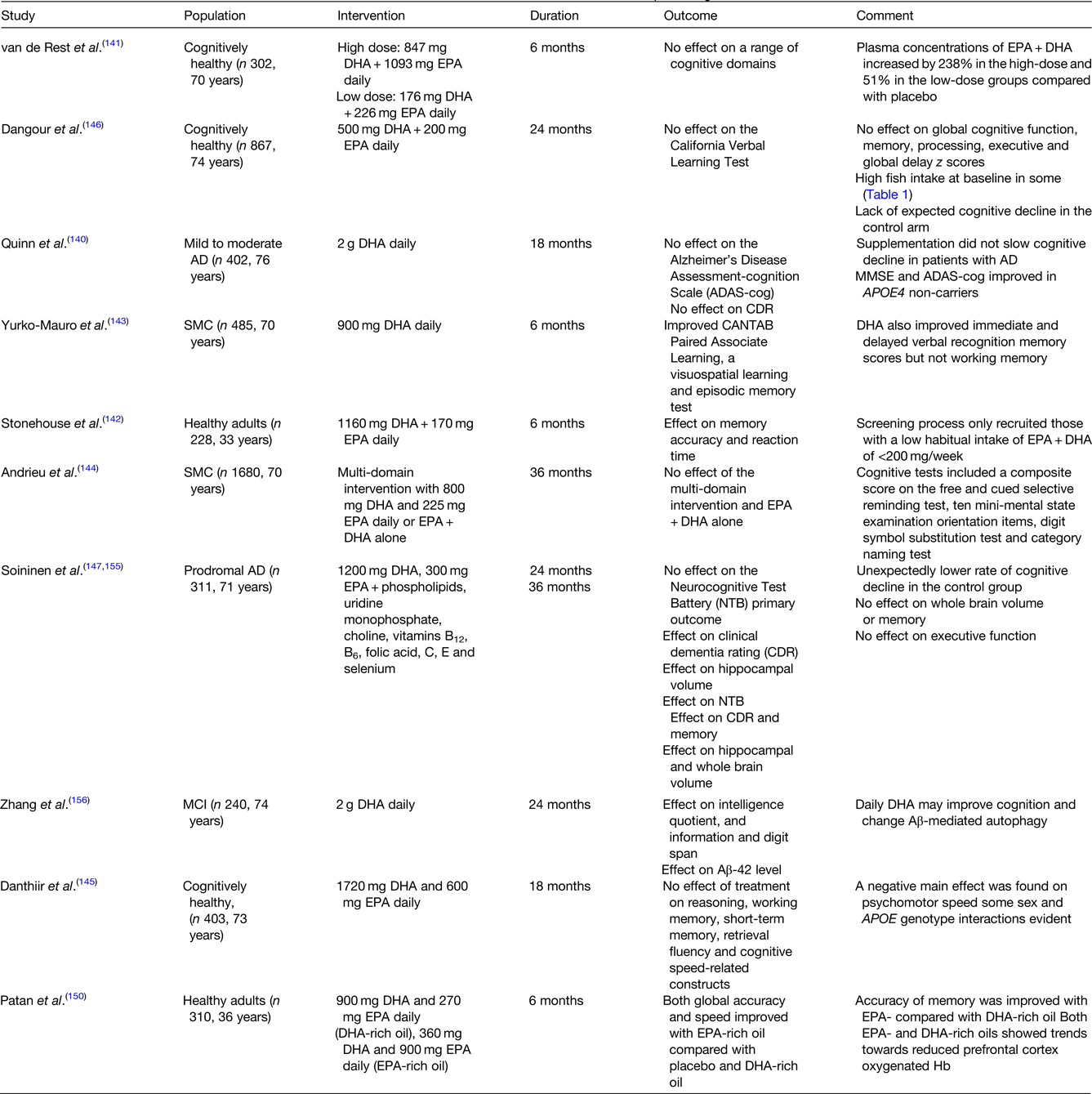
AD, Alzheimer's disease; Aβ, amyloid-β; CANTAB, cambridge neuropsychological test automated battery; MCI, mild cognitive impairment; MMSE, mini-mental state examination; RCT, randomised controlled trial; SMC, subjective memory complaints.
This table is by no means exhaustive. The RCTs included were >6 months in duration and were selected to demonstrate the discordance between individual study findings.
Cognitive benefits of EPA + DHA supplementation have not been observed in AD patients(Reference Quinn, Raman and Thomas140). In the Alzheimer's Disease Cooperative study, supplementation with 2⋅0 g DHA for 18 months in those with mild to moderate AD, did not affect the co-primary outcome measures, the cognitive subscale of the Alzheimer's Disease Assessment Scale (ADAS-cog) and the clinical dementia rating sum of boxes(Reference Quinn, Raman and Thomas140). An effect of intervention on the cognitive subscale of the Alzheimer's Disease Assessment Scale and the mini-mental state examination was, however, observed in APOE4 non-carriers. In a 2008 RCT in three-hundred and two cognitively healthy individuals, no effect of doses of 400 or 1900 mg EPA + DHA daily on a range of cognitive outcomes was evident(Reference van de Rest, Geleijnse and Kok141). This is in contrast to the findings of Stonehouse and colleagues who observed a significant impact of 1160 mg DHA + 170 mg EPA daily over 6 months, on the speed of episodic and working memory, and episodic memory performance in women only, with low habitual EPA and DHA intake at baseline (<200 mg/week)(Reference Stonehouse, Conlon and Podd142) with Yurko-Mauro et al., also observing improvements in a number of cognitive outcomes supplementing with 900 mg daily in those with subjective memory complaints for 6 months(Reference Yurko-Mauro, McCarthy and Rom143). This is in contrast to the Multidomain Alzheimer Preventive Trial (MAPT) (3 years, n 1680)(Reference Andrieu, Guyonnet and Coley144) and a more recent RCT (18 m, n 403)(Reference Danthiir, Hosking and Nettelbeck145) who observed no impact of 800 mg DHA plus 225 mg EPA daily or 1720 mg DHA plus 600 mg EPA, respectively, on cognitive performance. The MAPT intervention highlights the importance of participant selection, with a much higher mean education attainment in the study group relative to a general French population, which may provide cognitive reserve and have contributed to the lower than expected decline in cognitive function in the MAPT control group(Reference Andrieu, Guyonnet and Coley144) and also a number of other RCTs(Reference Dangour, Allen and Elbourne146,Reference Soininen, Solomon and Visser147) , which impacts study power. A secondary analysis in MAPT, in the control group showed the greatest cognitive decline in participants with the lowest quartile (Q1) v. Q2–Q4 of baseline erythrocyte EPA + DHA n-3 index (EPA + DHA), with the optimal n-3 index cut-off for predicting notable cognitive decline calculated as 5⋅3 %(Reference Coley, Raman and Donohue148). Using this cut-off, there was a consistent but non-significant difference in 3-year cognitive decline between EPA + DHA treated and placebo subjects with ‘low’ baseline n-3 index. The authors concluded that those with an n-3 index below approximately 5 % are at an increased risk of cognitive decline and could be a good target for recruiting a responsive population subgroup.
Intervention studies which target cognition have predominately fed DHA-rich or DHA-only supplements as the bioactive LC n-3 PUFA, based on the observation that brain DHA levels are >250 higher than EPA(Reference Bazinet, Metherel and Chen149). EPA does enter the brain with uptake efficiencies equivalent to DHA, but is thought to be rapidly metabolised following entry, although concentrations of EPA are higher than DHA in microglial cells(Reference Bazinet, Metherel and Chen149). The impact of EPA on cognition and depression is being increasingly recognised(Reference Bazinet, Metherel and Chen149–Reference Dyall151). In a recent intervention, Patan and colleagues observed a significant effect of an EPA-rich oil on cognitive global accuracy and speed relative to a DHA-rich or placebo oil fed for 6 months (Table 1). Future interventions should not only consider what dose, but also what DHA:EPA ratio of the supplement and its chemical form (ethyl ester, TAG and phospholipid LPC)(Reference Patrick152).
The prodrome of AD is thought to be 20–30 years or potentially longer(Reference Sperling, Aisen and Beckett153). Therefore, cognitive assessment or brain volume and atrophy (assessed by MRI) rather than incident disease have to date been exclusively used as primary RCT outcomes. Cognitive questionnaires and other assessment tools historically may not have been fully fit for purpose, lacking the specificity and sensitivity to detect subtle effect of intervention on specific cognitive domains. The variability and lack of a standard battery of cognitive tests employed in cognitive RCTs is likely to be a large contributor to the heterogeneity in finding, between trials.
Furthermore, due attention is not given to the length of the intervention period. As brain DHA half-life is estimated to be 2⋅5 years(Reference Umhau, Zhou and Carson154), supplementation periods of at least 1 year are likely to be needed to detect the cognitive benefits associated with DHA enrichment of neuronal cells, such as effects on dendrite outgrowth and spine density, synaptic function and Aβ processing. The impact of intervention period on the study conclusions is evidenced by comparing the 24 month and 36 month findings from the LipiDiDiet study which fed the Souvenaid (Fortasyn Connect) medicinal food, which combines 1200 mg DHA and 300 mg EPA with phospholipids, uridine monophosphate, choline, vitamins B12, B6, folic acid, C, E and selenium. At 24 months, although an effect of Souvenaid on secondary outcomes was observed (hippocampal volume and clinical dementia rating score) no effect of the intervention on the primary outcome, the neurocognitive test battery performance, was evident. By 36 months the intervention had significantly increased the neurocognitive test battery test score by 60 % relative to the control group, with the greatest cognitive benefits (based on the clinical dementia rating score) evident in those with the highest cognitive status at baseline (mini-mental state examination ≥29).
There is a strong justification and need to conduct a future RCT in ‘at-risk’ cognitively healthy participants with incident dementia or AD as the primary outcome. Such a trial is likely to require at least a 5-year intervention period and several thousand per intervention arm, given the long prodrome and AD incident rates. Careful enrichment of the trial with an at-risk responsive population based on such factors at APOE genotype status, cardiovascular risk profile, baseline EPA + DHA status, education attainment, brain imaging and blood biomarker profiles is key to success. Consideration should also be given not only to the LC n-3 PUFA dose, but also the DHA:EPA ratio and chemical form.
Final thoughts
The prospective and experimental evidence for the role of DHA in brain function and the cognitive benefits of increased fish, and LC n-3 PUFA intakes are convincing with large effect sizes. It is likely that EPA and DHA have complementary neurophysiological benefits, which includes an effect on oxylipin production, and should be co-supplemented or ideally consumed as oily rich fish. Confirmation of the cognitive benefits is needed from the well-designed RCTs which include large population subgroups who are likely to be most responsive and gain most benefit. Accumulating evidence suggests that APOE4 carriers have a lower brain uptake and status and would particularly benefits from DHA intervention prior to any significant neuropathology, which affects brain DHA uptake.
Financial Support
Financial support for our work included in the review has been provided by the BBSRC (BB/M004449/1), EU-JPI/BBSRC, (BB/P028233/1) Alzheimer's Society (AS-PhD-2015-023), Alzheimer's Research UK (PRRF2017-006), the UK Foods Standards Agency (N05065 and N05066) and the UK Medical Research Council (U105960389 and U1052.00.014).
Conflict of Interest
None.
Authorship
The authors had sole responsibility for all aspects of preparation of this paper.