Fish oil (FO) is a major source of n-3 PUFA, which include EPA and DHA( Reference Calder 1 ). Several studies have demonstrated the cardiovascular-protective potential of n-3 PUFA( Reference Lavie, Milani and Mehra 2 , Reference Jump, Depner and Tripathy 3 ). Many studies have suggested that FO not only prevents detrimental cardiovascular events but also lowers the mortality rate for individuals with CVD( Reference Rizos, Ntzani and Bika 4 , Reference Balakumar and Taneja 5 ). In addition to lowering the amount of lipids in the blood, n-3 PUFA in FO can regulate blood pressure and enhance vascular integrity and compliance( Reference Schwellenbach, Olson and McConnell 6 ). Additionally, n-3 PUFA have the ability to protect vascular endothelial cells by decreasing oxidative stress, halting atherosclerotic events and preventing vascular inflammatory and adhesion cascades( Reference Massaro, Habib and Lubrano 7 ). Interestingly, recent studies have demonstrated that n-3 PUFA improve vascular endothelial function by enhancing the generation and bioavailability of the endothelium-derived relaxing factor through the up-regulation and activation of endothelial NO synthase( Reference Taneja, Mahadevan and Balakumar 8 , Reference Turgeon, Dussault and Maingrette 9 ). This knowledge has uncovered a new area of research to identify potential mechanisms that influence FO-mediated functional regulation of the vascular endothelium.
Atherosclerotic CVD remains the leading cause of cardiovascular morbidity and mortality worldwide. A key mechanism in atherogenesis is endothelial dysfunction, which is characterised by decreased NO bioavailability and the development of an inflammatory phenotype( Reference Hansson 10 ). Increasing evidence has shown the importance of mitochondrial function in the regulation of endothelial homeostasis( Reference Shenouda, Widlansky and Chen 11 , Reference Ong and Hausenloy 12 ). Mitochondrial function is maintained by the fusion and fission of these dynamic organelles. Mitochondrial fission and fusion are known to play essential roles in maintaining normal mitochondrial morphology and dynamics( Reference Youle and van der Bliek 13 ). The proteins that control fusion include mitofusin (Mfn)-1, Mfn2 and optic atrophy-1 (Opa1). Fission is regulated by dynamin-related protein-1 (Drp1) and fission-1 (Fis1). Fusion may be beneficial because it allows the distribution of metabolites, proteins and DNA throughout the mitochondrial network( Reference Youle and van der Bliek 13 ). Dysfunctional mitochondria and damaged mitochondrial components are eliminated by fission and are subsequently subjected to lysosome-dependent autophagy( Reference Twig, Elorza and Molina 14 , Reference Zhang, Qi and Taylor 15 ). Under pathological conditions, such as atherosclerotic disease, fission is increased and autophagy is impaired, which leads to a loss of mitochondrial networks, an accumulation of small dysfunctional mitochondria and an increase in mitochondrial reactive oxygen species (ROS)( Reference Davidson and Duchen 16 ).
However, it is uncertain whether FO supplementation can alter mitochondrial dynamics and improve endothelial function. The present study was designed to investigate the protective effects of FO on altered mitochondrial dynamics and impaired endothelial cell function under proatherogenic conditions in an apoE− / − mouse model.
Materials and methods
Diets
Semi-purified high-fat diets (HFD) containing 45 % fat (approximately 45 % of total energy from fat)( Reference Wang, Zhang and Wang 17 ) and HFD with partial replacement of lard by 10 % (w/w) FO (containing 10 % EPA (20 : 5n-3) and 50 % DHA (22 : 6n-3) and providing 45 % of total energy from fat) were provided by Medicience Limited. FO was chemically refined, and then degummed, neutralised, bleached, deodorised and stabilised with delta-enriched mixed tocopherols. The oil was shipped at 4°C and stored at − 25°C to minimise autoxidation.
Animal experiments
ApoE− / − mice with the C57BL/6 genetic background were provided by Jackson Laboratory. A total of eighteen male apoE− / − mice (8 weeks of age) were randomly divided into two groups (n 12 per group) and were fed either the HFD or FO diet (total EPA and DHA content 64·1 g/kg) for 8 weeks. The animals were housed in a specific pathogen-free facility (n 3 animals per cage) in a temperature-controlled room (22–24°C) with a 12 h light–dark cycle and given free access to food and water. Mice received a fresh diet every 3rd day, and food consumption and body weight were monitored weekly. Institutional and national guidelines for the care and use of animals were followed, and all experimental procedures involving animals were approved by the Institutional Animal Care and Use Committee of Sun Yat-sen University.
Serum lipid concentrations and blood pressure
After the intervention period, blood samples were collected from the lateral saphenous vein using EDTA as an anticoagulant after mice were fasted for 12 h. Serum lipid concentrations were measured using enzymatic colorimetric methods as described previously( Reference Davidson and Duchen 16 ). Systolic blood pressure and heart rate were measured by a computerised tail-cuff system (BP-2000; Visitech Systems) in conscious animals.
Endothelium-dependent vasorelaxation
For endothelial function experiments, thoracic aortas were cut into rings (2–3 mm long). The rings were attached to a force transducer, suspended in an organ chamber filled with 6 ml of Krebs–Ringer solution (125 mm-NaCl, 2·5 mm-KCl, 2·0 mm-CaCl2, 1·0 mm-MgCl2, 1·25 mm-NaH2PO4, 25 mm-NaHCO3 and 25 mm-glucose, 37°C, pH 7·4), and then bubbled with 95 % O2 and 5 % CO2. The rings were slowly subjected stepwise to a predetermined optimal tension of 30 mN and allowed to equilibrate for at least 30 min. Following equilibration, each ring was pre-contracted with phenylephrine (3 μm). Following 60–90 min of contraction, a relaxation concentration response curve was generated by adding four cumulative additions of the endothelium-dependent vasodilator acetylcholine (Ach, 10− 9 to 10− 6 m) every 3 min. In addition, sodium nitroprusside (10− 6 m, endothelium-independent NO-releasing agent) was added to the organ bath, and endothelium-independent vasorelaxation was recorded( Reference Wang, Zhang and Wang 17 ).
Evaluation of atherosclerotic lesions
The presence of atherosclerotic lesions in the aortic sinus was measured using oil red O staining as described previously( Reference Wang, Zhang and Wang 17 ). The atherosclerotic plaque area of the aortic sinus was reported as the net area and the ratio of the total intimal plaque lesion area to the total cross-sectional vessel wall area, which was defined by the external elastic lamina.
Isolation of mitochondrial fraction
Thoracic aortas were suspended in a mitochondrial buffer (10 mm-3-(N-morpholino) propane sulfonic acid (MOPS), pH 7·2, 10 mm-KCl, 1·5 mm-MgCl2, 1 mm-EDTA, 10 μg leupeptin/ml, 10 μg aprotinin/ml and 0·25 m-sucrose) and then homogenised with a Dounce homogeniser. The homogenate was centrifuged at 750 g for 10 min at 4°C to remove nuclei and debris, and the resulting supernatant was combined and centrifuged at 13 000 g for 10 min. The mitochondrial extract was finally resuspended in a lysis buffer. Protein concentration was determined by the bicinchoninic acid protein assay (Pierce)( Reference Pung, Rocic and Murphy 18 ).
Mitochondrial DNA integrity
Mitochondrial DNA integrity was assessed in the mitochondria isolated from the cells obtained from the thoracic aorta of mice in both HFD and FO groups using quantitative PCR-based amplification of a large fragment of mitochondrial DNA as described previously( Reference Paneni, Osto and Costantino 19 ). Briefly, total DNA (30 ng) was used in two PCR: one amplifying a short 117 bp product of mtDNA and the other amplifying a long fragment of a 10 kb product of mtDNA. The primer sequences used were 10 kb forward 5′-GCCAGCCTGACCCATAGCCATAATAT-3′ and reverse 5′-GAGAGATTTTATGGGTGTAATGCGG-3′ and 117 bp forward 5′-CCCAGCTACTACCATCATTCAAGT-3′ and reverse 5′-GATGGTTTGGGAGATTGGTTGATG-3′. mtDNA integrity was determined by calculating the ratio of the amount of 10 kb products to that of 117 bp products.
Assays for mitochondrial enzyme complexes
The activities of NADH–ubiquinone oxidoreductase (complex I), succinate–CoQ oxidoreductase (complex II), ubiquinol–cytochrome c reductase (complex III), cytochrome c oxidase (complex IV) and ATP synthase (complex V) in the thoracic aortas of mice from the HFD and FO groups were measured spectrometrically using conventional assays as reported previously( Reference Feng, Zou and Jia 20 ).
Measurements of mitochondrial superoxide anion, nitric oxide and peroxynitrite from mouse aortas
Concurrent measurements of NO, superoxide anion (
$$O _{2}^{ - } $$
) and peroxynitrite (ONOO−) were performed with three electrochemical nanosensors combined into one working unit with a total diameter of 2·0 to 2·5 μm. The nanosensor design was based on previously developed and well-characterised chemically modified carbon-fibre technology(
Reference Paneni, Osto and Costantino
19
). Differential pulse voltammetry was used to measure basal NO,
$$O _{2}^{ - } $$
and ONOO− concentrations, and amperometry was used to measure the changes in NO,
$$O _{2}^{ - } $$
and ONOO− concentrations from the basal level over time. The assays were operated in amperometric mdoe with a computer-based Gamry VFP600 multichannel potentiostat. The maximum current of the peak should be observed at a potential of 0·63 to 0·67 V (at 40 mV pulse amplitude) and for ONOO− at − 0·42 to − 0·38 V. Linear calibration curves (current v. concentration) were constructed for each sensor from 10 nm to 2 μm before and after measurements with aliquots from standard solutions of NO and ONOO−, respectively. The consumption of redox species by nanosensors depends on the area of the electrode ( < 0·12 μm2), and the duration of electrolysis was from 5 to 10 s. In amperometric measurements, peak concentrations of NO and ONOO− varied between 0·04 and 0·1 %. To maintain a constant distance from the cells, the module of sensors was lowered until it reached the surface of the cell membrane. Thereafter, the sensors were slowly raised 4+1 μm (z-coordinates) from the surface of the cells. The sensors were then moved horizontally (x- and y-coordinates) and positioned above a surface of randomly chosen single endothelial cells in an aortic ring.
Mitochondrial swelling assay
Isolated mitochondria (40 μg) from mouse aortas in a swelling buffer (250 mm-sucrose, 10 mm-3-(N-morpholino)propanesulfonic acid, 5 μm-ethylene glycol tetraacetic acid, 2 mm-MgCl2, 5 mm-KH2PO4, 5 mm-pyruvate and 5 mm-malate) were incubated with 150 μm-CaCl2 in a final volume of 200 μl in a ninety-six-well plate for 20 min. Absorbance was read every 30 s at 520 nm( Reference Borillo, Mason and Quijada 21 ).
Caspase-3 activity assay
Caspase-3 activity was measured in the homogenates of abdominal aortas from the HFD or FO group according to the manufacturer's instructions (Caspase-Glo 3/7 assay kit; Promega). The aortas were dissected and immediately homogenised in cell culture lysis reagent (Promega), centrifuged at 10 000 g for 5 min at 4°C to remove insoluble proteins, and then stored at − 80°C. Aortic homogenates of 20 μg protein were assayed for caspase-3 activity by incubating with the same volume of caspase-3 luminogenic substrate at room temperature. Luminescence was measured within 30–60 min. Activity was measured in three separate experiments, and each sample was measured in duplicate.
Terminal deoxynucleotidyl transferase dUTP nick-end labelling assay
Apoptosis was evaluated using the DeadEnd Colorimetric TUNEL System (Promega) with in situ detection according to the manufacturer's instructions. The thoracic aorta was paraffin-embedded, sectioned, deparaffinised with fresh xylene and washed with graded ethanol. Subsequently, the recombinant terminal deoxynucleotidyl transferase reaction mix was added inside a humidified chamber at 37°C for 60 min. Endogenous peroxidases were blocked by immersing the slides in 0·3 % H2O2 for 3–5 min at room temperature. Then, streptavidin–horseradish peroxidase solution was added, the slides were incubated for 30 min and 3,3′-diaminobenzidine tetrahydrochloride was added to develop the slides( Reference Yang, Sato and Aprahamian 22 ).
Western blot
Abdominal aortas were homogenised in ice-cold RIPA lysis buffer (1 μg leupeptin/ml, 5 μg aprotinin/ml, 100 μg phenylmethanesulfonyl fluoride/ml, 1 mm-sodium orthovanadate, 1 mm-ethylene glycol tetraacetic acid, 1 mm-EDTA, 1 mm-NaF and 2 mg β-glycerolphosphate/ml). Then, the homogenates were incubated on ice for 20 min and centrifuged at 20 000 g for another 20 min at 4°C. The supernatant was collected, and protein concentration was quantified by the bicinchoninic acid protein assay (Pierce). Aliquots from 40 μg of protein extracts were separated by SDS–PAGE and then transferred onto an Immobilon-P membrane (Millipore). After blocking, the blots were incubated with various primary antibodies followed by horseradish peroxidase-labelled secondary antibodies. Protein bands were detected with a SuperSignal West Pico Chemiluminescent kit (Pierce). Primary antibodies used were as follows: anti-Mfn2 antibody (sc-50 331; Santa Cruz); anti-Opa1 antibody (sc-30 573; Santa Cruz); anti-Fis1 antibody (sc-98 900; Santa Cruz); anti-Drp1 antibody (sc-134315; Santa Cruz); anti-cytochrome c antibody (no. 4280; Cell Signaling Technology); anti-β-actin antibody (no. 4967; Cell Signaling Technology).
Cyclic GMP production
Isolated aortas were harvested and rinsed with PBS, and the adventitia was dissected away. Cyclic GMP concentrations were determined in aortic homogenates using the Cyclic GMP XP Assay kit (Cell Signaling Technology) and normalised to total protein concentrations.
Statistical analysis
Data were analysed using Quantity One (version 4.6.2), GraphPad Prism (version 5.0) and SPSS (version 17.0). All variables were tested by the Shapiro–Wilk test for normal distribution. Independent t tests and Wilcoxon tests were used when appropriate. P< 0·05 was considered as statistically significant. Results are presented as means and standard deviations.
Results
Body weight and food intake
Food intake levels were not different between the two groups at either the beginning (2·22 (sd 0·29) g) or the end (2·23 (sd 0·28) g) of the study (Fig. 1(a)). Body weights differed between the two groups during the last 3 weeks of the study; the body weight of mice fed the FO diet was significantly lower than that of the HFD-fed mice after 8 weeks of intervention (P< 0·01; Fig. 1(b)).
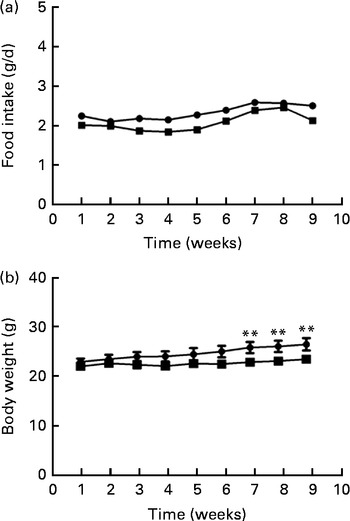
Fig. 1 Food intake (a) and body weight (b) of apoE− / − mice fed a high-fat diet or a high-fat diet enriched with 10 % fish oil
for 8 weeks. Values are means (n 12), with standard deviations represented by vertical bars. ** Mean value was significantly different from that of the high-fat diet group (P< 0·01).
Serum lipid concentrations and blood pressure
Total serum cholesterol and TAG concentrations were higher in FO-fed mice than in HFD-fed mice (P< 0·05). HDL- and LDL-cholesterol concentrations did not differ between the two groups. There were no significant differences in systolic blood pressure or heart rate between the two groups (Table 1).
Table 1 Blood lipid concentrations and blood pressure of apoE−/− mice from the groups fed the high-fat diet (HFD group) and the high-fat diet enriched with 10 % fish oil (FO group) (Mean values and standard deviations; n 12)
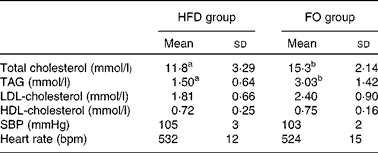
SBP, systolic blood pressure; bpm, beats per min.
a,bMean values within a row with unlike superscript letters were significantly different (P< 0·05).
Relaxation response
We tested vascular function in mice using isometric tension studies. The endothelium-dependent arterial relaxation responses of the isolated thoracic aortic rings to endothelium-dependent acetylcholine (Ach) were measured. Compared with HFD-fed mice, FO supplementation resulted in an increased endothelium-dependent vasorelaxation response to Ach, indicating a significant improvement in endothelial function (P< 0·01; Fig. 2(a)). Endothelium-independent arterial relaxation in response to sodium nitroprusside did not differ between the two groups (Fig. 2(b)).
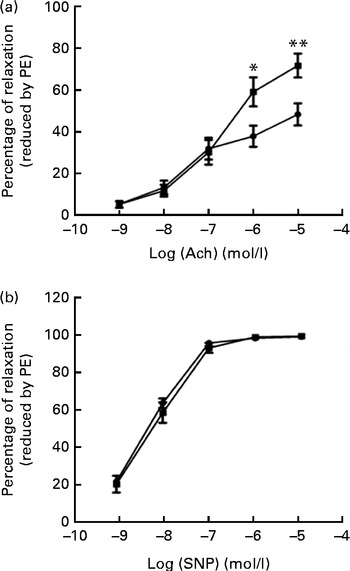
Fig. 2 Vascular reactivity. (a) Endothelium-dependent relaxation response to the increasing concentrations of acetylcholine (Ach). (b) Endothelium-independent relaxation response to sodium nitroprusside (SNP) in the thoracic aortic rings pre-contracted with 3 μm-phenylephrine (PE). Values are means (n 12), with standard deviations represented by vertical bars. Mean value was significantly different from that of the high-fat diet group: * P< 0·05, ** P< 0·01.
, High-fat diet enriched with 10 % fish oil.
Atherosclerotic plaque area
We then quantified the total atherosclerotic lesion area in HFD- and FO-fed apoE− / − mice. The HFD produced aortic atherosclerotic lesion areas in the aortic sinus, as shown by oil red O staining (Fig. 3(a)). Treatment with FO significantly decreased the mean atherosclerotic lesion area ((2·25 (sd 0·91)) × 105μm2) in the aortic sinus by 62·90 (sd 7·84) % compared with HFD-fed apoE− / − mice ((6·07 (sd 3·35)) × 105μm2) (P< 0·01; Fig. 3(b)). These data clearly showed that chronic FO treatment significantly inhibited the formation of atherosclerotic lesions in HFD-fed apoE− / − mice.
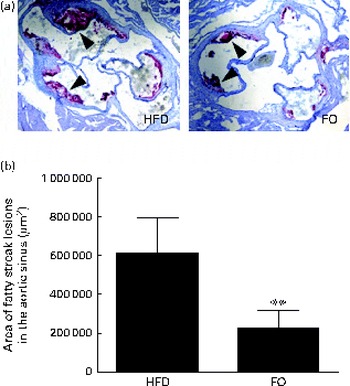
Fig. 3 Atherosclerotic lesions. (a) Representative photomicrographs of the aortic sinus stained with oil red O are shown. The arrows indicate fatty streak lesions. 100 × magnification. (b) The area of fatty streak lesions in the aortic sinus is calculated with software LAS version 3.8. Values are means, with standard deviations represented by vertical bars. ** Mean value was significantly different from that of the high-fat diet (HFD) group (P< 0·01). FO, high-fat diet enriched with 10 % fish oil.
Mitochondrial dynamic protein expression and respiratory chain complexes
ApoE− / − mice fed FO had a higher level of mitochondrial fusion proteins, including Mfn2 and Opa1 (both P< 0·05), than HFD-fed mice. Conversely, FO-fed mice had lower protein expression levels of Fis1 than HFD-fed mice (P< 0·05). There was no significant difference in the protein expression levels of Drp1 between the two groups (Fig. 4(a) and (b)). ApoE− / − mice in the FO group had higher complex I–IV activities compared with those in the HFD group (P< 0·05). Conversely, mice in the FO group had lower complex V activity than those in the HFD group (P< 0·05). Complex III activity was similar between the two groups (Table 2).
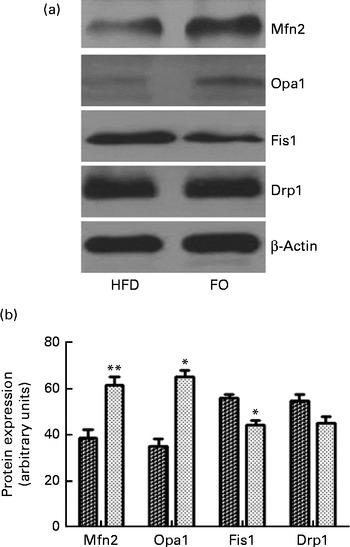
Fig. 4 Mitochondrial fusion and fission protein expression in the aortas. (a) Representative Western blotting images. β-Actin is a comparative control. (b) Densitometric quantification of the expression levels of mitofusin-2 (Mfn2), optic atrophy-1 (Opa1), fission-1 (Fis1) and dynamin-related protein-1 (Drp1). Fusion proteins include Mfn2 and Opa1; fission proteins include Fis1 and Drp1. Values are means of three experiments, and the bands are representative of twelve mice, with standard deviations represented by vertical bars. Mean value was significantly different from that of the high-fat diet (HFD; ) group: * P< 0·05, ** P< 0·01. FO
, high-fat diet enriched with 10 % fish oil.
Table 2 Activities of mitochondrial respiratory chain complexes in the aorta of apoE−/− mice from the groups fed the high-fat diet (HFD group) and the high-fat diet enriched with 10 % fish oil (FO group) (Mean values and standard deviations; n 12)
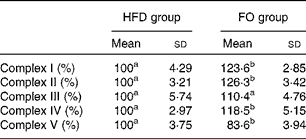
a,bMean values within a row with unlike superscript letters were significantly different (P< 0·05).
Mitochondrial derangement and oxidative stress
Mitochondrial
$$O _{2}^{ - } $$
levels were 52 % lower in FO-fed mice than in HFD-fed mice (P< 0·05). The mitochondrial swelling assay showed that the mitochondria from FO-fed mice displayed a significant increase in absorbance after a Ca overload compared with those from HFD-fed mice (Fig. 5(a)). Mitochondrial DNA integrity in the FO group was approximately twofold greater than that in the HFD group (Fig. 5(b)), whereas caspase-3 activity was 27·6 % lower in the FO group than in the HFD group (P< 0·05). In addition, mice fed FO had less cytochrome c release compared with those fed the HFD (Fig. 5(c)). Terminal deoxynucleotidyl transferase dUTP nick-end labelling (TUNEL) staining showed that the percentage of apoptotic endothelial cells in the aorta from FO-fed mice was significantly decreased compared with that from HFD-fed mice (Fig. 5(d)).
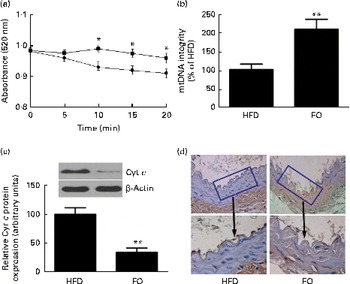
Fig. 5 Mitochondrial derangement and apoptosis. (a) Line graphs represent a decrease in light absorbance over time in the presence of calcium overload (CaCl2; 150 μm). Values are means, with their standard deviations represented by vertical bars. * Mean value was significantly different from that of the high-fat diet (HFD; ) group (P< 0·05). FO
, high-fat diet enriched with 10 % fish oil. (b) Assessment of mitochondrial DNA (mtDNA) integrity by real-time PCR in the mitochondria isolated from the aortas of HFD- and FO-fed mice. Values are means, with standard deviations represented by vertical bars. ** Mean value was significantly different from that of the HFD group (P< 0·01). (c) Representative Western blotting photographs of cytochrome c (Cyt c). Densitometric quantification of Cyt c protein expression. Values are means of three experiments, and the bands are representative of twelve mice, with standard deviations represented by vertical bars. ** Mean value was significantly different from that of the HFD group (P< 0·01). (d) Brown staining in the endothelial layer identifies apoptotic cells. Each photomicrograph is representative of a study conducted in six mice.
Endothelial nitric oxide synthase activation and nitric oxide release
In agreement with decreased mitochondrial
$$O _{2}^{ - } $$
generation, NO release from single aortic endothelial cells was higher in the FO group (238·6 (sd 28·5) nm) than in the HFD group (112·7 (sd 15·8) nm) (P< 0·01). Cyclic GMP concentration in the aorta was higher in FO-fed mice (57·1 (sd 3·02) pm/mg protein) than in HFD-fed mice (7·10 (sd 1·02) pm/mg protein) (P< 0·01). Increased NO availability was associated with greater activation of endothelial NO synthase Ser-1177 phosphorylation levels (Fig. 6(a)) and endothelial NO synthase activity (Fig. 6(b)) in FO-fed mice compared with HFD-fed mice. Furthermore, aortic ONOO− levels were reduced in FO-fed mice (71·3 (sd 11·3) nm) compared with HFD-fed mice (130·9 (sd 10·5) nm) (P< 0·01).
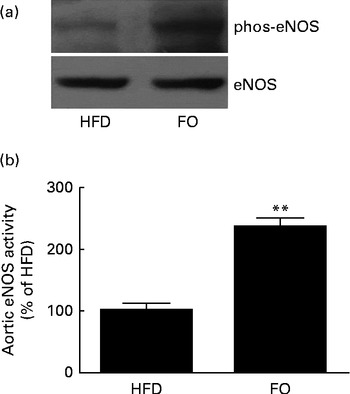
Fig. 6 Endothelial NO synthase (eNOS) expression and activity in the aortas. (a) Representative Western blots of Ser-1177 phosphorylation (phos) levels of eNOS. The blots are representative of six mice. HFD, high-fat diet; FO, high-fat diet enriched with 10 % fish oil. (b) eNOS activity in the aortas from the HFD group was defined as 100 %, and the same activity in the FO group was expressed as a percentage change of this control value. ** Mean value was significantly different from that of the HFD group (P< 0·01).
Discussion
In the present study, we demonstrated a novel mechanism by which FO preserves endothelial cell function and inhibits atherogenesis. In contrast to apoE− / − mice fed a HFD, impairment of endothelial-dependent arterial relaxation was ameliorated in FO-fed mice. As a result, the HFD supplemented with FO significantly reduced atherosclerotic plaque formation in apoE− / − mice. A marked decrease in mitochondrial
$$O _{2}^{ - } $$
concentrations, paralleled by enhanced endothelial NO bioavailability and decreased ONOO− concentrations, was found in the aorta of mice receiving FO. FO supplementation improved mitochondrial function by decreasing the rate of mitochondrial swelling, decreasing mitochondrial DNA fragmentation and attenuating caspase-3 activity. Furthermore, FO supplementation increased the expression levels of the mitochondrial fusion proteins Mfn2 and Opa1 but decreased the protein expression levels of Fis1 in the aorta.
Many studies have shown that high fish intake, n-3 PUFA intake or status is associated with reduced progression of coronary artery atherosclerosis( Reference Erkkilä, Matthan and Herrington 23 , Reference Erkkilä, Lichtenstein and Mozaffarian 24 ). Similar to these studies, we confirmed the anti-atherogenic effects of n-3 PUFA. FO supplementation in a HFD attenuated the atherosclerotic plaque volume in apoE− / − mice, a widely used atherosclerotic mouse model, compared with HFD-fed mice. The amount of EPA and DHA provided in the FO diet from the present study was 64·08 g/kg diet, i.e. 10·68 g EPA and 53·4 g DHA. This dose is similar to the amount used in clinical intervention trials and is an amount achievable through the regular consumption of oily fish or fish oil capsules( Reference von Schacky, Angerer and Kothny 25 ). This dose has been demonstrated to enhance the stability of atherosclerotic plaques in a randomised controlled trial of patients with advanced carotid atherosclerosis( Reference Thies, Garry and Yaqoob 26 ). The protective effects on the initiation and progression of atherosclerosis by FO were mainly attributed to the ready incorporation of n-3 PUFA into atherosclerotic plaques and exhibition of the anti-inflammatory effects( Reference Brown, Zhu and Rong 27 , Reference Cawood, Ding and Napper 28 ).
Endothelial dysfunction is a pivotal event in the initiation and progression of atherogenesis( Reference Bonetti, Lerman and Lerman 29 ). ApoE− / − mice fed a Western-type diet represents a well-characterised animal model of hyperlipidaemia-induced endothelial dysfunction( Reference Wang, Zhang and Wang 17 ). Concomitant with a significant reduction in atherosclerotic lesion size, dietary supplementation with FO ameliorated HFD-induced endothelial dysfunction in apoE− / − mice, indicating the direct effects of FO on the endothelium. Previous studies have documented the hypolipidaemic effects of FO in human subjects( Reference Milte, Coates and Buckley 30 ); however, the effect FO exerted on endothelial dysfunction and atherosclerosis in the present study is unlikely due to the changes in lipid concentrations because FO supplementation did not exert any metabolic influences on dyslipidaemia. There are several possible explanations for these findings. First, the FO dose used in the present study was lower compared with doses reported to effectively decrease blood lipid concentrations( Reference Milte, Coates and Buckley 30 – Reference Okuda, Ueshima and Okayama 32 ). Furthermore, apoE deficiency in mice has been shown to result in severe hypercholesterolaemia due to the accumulation of VLDL and remnant particles. However, the lipid profile of mice is very different from that of humans, who carry approximately 75 % of their plasma cholesterol on LDL( Reference Pendse, Arbones-Mainar and Johnson 33 ). More importantly, a recent cross-sectional analysis has shown that the ApoE genotype differentially modified the association between plasma n-3 fatty acids and plasma lipid levels( Reference Liang, Steffen and Steffen 34 ). Thus, FO may not exhibit the hypolipidaemic effects in apoE− / − mice without the assistance of the ApoE gene.
The primary cause of induced endothelial cell dysfunction is endothelial cell injury or death due to excessive oxidative stress in the endothelium(
Reference Pober, Min and Bradley
35
). The mitochondria are the main target of damage caused by ROS(
Reference Adam-Vizi and Chinopoulos
36
). In line with this notion, we found that
$$O _{2}^{ - } $$
generation was significantly elevated in HFD-fed mice and largely diminished in FO-fed mice. The improved oxidant-rich milieu observed in FO-fed mice was associated with decreased NO breakdown, as indicated by reduced ONOO− levels. Mice receiving FO also showed decreased DNA fragmentation and swelling of the mitochondria, indicating improved mitochondrial derangement. Our findings may be contrary to previous studies showing that n-3 PUFA up-regulate ROS and are readily susceptible to peroxidation by ROS(
Reference Hong, Chapkin and Barhoumi
37
). This inconsistency was attributed to the different cell types used. Furthermore, DHA has been shown to be preferentially incorporated into cardiolipin(
Reference McMillin and Dowhan
38
), an important diphosphatidylglycerol lipid component of the inner mitochondrial membrane. Cardiolipin appears particularly susceptible to ROS attack, which results in a decrease in cardiolipin levels in the mitochondrial membrane and the initiation of the apoptotic process(
Reference Paradies, Petrosillo and Pistolese
39
,
Reference Goldstein, Muñoz-Pinedo and Ricci
40
). Thus, DHA may directly interact with the mitochondria by incorporating into the mitochondrial membrane. This interaction may result in the modulation of mitochondrial function through the maintenance of membrane integrity, a decrease in ROS production and an increase in the membrane's resistance to oxidative stress.
Release of cytochrome c from the mitochondria in response to apoptotic signals is a central event in the apoptotic process( Reference Li, Nijhawan and Budihardjo 41 ). The present data suggested that FO-fed mice had lower levels of cytochrome c release from the mitochondria in the aorta than HFD-fed mice, indicating lower levels of apoptosis. Specifically, cytochrome c is a component of the apoptosome that facilitates the downstream activation of caspase-3 and caspase-7 and triggers the apoptotic process( Reference Sheridan and Martin 42 ). FO supplementation markedly decreased caspase-3/7 activities in response to HFD feeding. Thus, the protection of endothelial cell function in hyperlipidaemic apoE− / − mice by FO was closely associated with the induction of an antiapoptotic response in the endothelium, which overrode the proapoptotic response of caspase-3/7 activation. Furthermore, cellular apoptosis was significantly attenuated in the vascular endothelium of FO-fed mice compared with that of HFD-fed mice, as assessed by the in situ TUNEL technique, a common method for detecting DNA fragmentation. As a result, FO supplementation enhanced the production of aortic cyclic GMP, a key marker for the maintenance of NO bioavailability and vascular integrity( Reference Pober, Min and Bradley 35 ).
The mitochondria dynamically undergo fusion and fission processes, which are critical for the release of cytochrome c and are associated with increased cellular apoptosis( Reference Leboucher, Tsai and Yang 43 ). We hypothesised that FO would affect mitochondrial morphology by inducing alterations in fusion proteins, such as Mfn1 and Mfn2, and fission proteins, such as Drp1. Our findings demonstrated that the expression of the mitochondrial proteins Mfn2 and Opa1 was higher in the aorta of apoE− / − mice fed FO than that of HFD-fed mice. Conversely, FO supplementation resulted in a significant decrease in the expression of the fission protein Fis1 in the aorta. These findings suggest that FO-induced alterations in mitochondrial dynamics improved endothelial cell function. However, the underlying mechanism for this observation is unknown and warrants further study.
The main limitations of the present study are the very small sample size in each group and the absence of a control group (low-fat/normal-fat-fed diet). Second, supplementation of FO to a HFD largely contained saturated fat instead of FO per se. Third, the present study lacked a dose–response design. Another limitation is that we did not determine the DHA content in the plaques of apoE− / − mice receiving FO. Thus, the lack of significant enrichment of plaques with DHA may have reduced the likelihood of finding more marked effects on the plaque outcomes assessed here.
Taken together, the findings of the present study reveal a novel mechanism by which FO ameliorates HFD-induced endothelial cell dysfunction and atherosclerosis by improving mitochondrial oxidative stress via the regulation of mitochondrial dynamic protein expression.
Acknowledgements
The present study was supported by grants from the National Natural Science Foundation of China (no. 81072301), the National Natural Science Foundation from Guangdong Province (no. S2012020011104) and Project Supported by the Guangdong Province Universities and Colleges Funded Scheme (2011). All of the aforementioned funders had no role in the design, analysis or writing of this article.
The contributions of each author were as follows: X. W. conducted the research; X. W., R. S. and Y. L. analysed the data; M. X. designed the experiments and prepared the manuscript. All authors read and approved the final manuscript.
None of the authors has any conflict of interest to declare.