Introduction
Current chemical strategies to control gastrointestinal nematodes in animal agriculture are heavily dependent on regular treatment with broad-spectrum anthelmintic drugs. Similarly, in poultry, anthelmintics have been the mainstay for the control of helminth infections worldwide (Ruff, Reference Ruff1999; Gauly et al., Reference Gauly, Bauer, Mertens and Erhardt2001). Relatively few anthelmintics have been registered and approved to control parasitic nematodes of economic importance in poultry (Permin & Hansen, Reference Permin and Hansen1998; McDougald, Reference McDougald, Swayne, Boulianne and Logue2020). Heavy reliance on a limited number of drugs combined with application of sub-therapeutic doses during mass application are likely to increase selection pressure for anthelmintic resistance (AR) in poultry nematodes (Knapp-Lawitzke et al., Reference Knapp-Lawitzke, Krücken, Ramünke, von Samson-Himmelstjerna and Demeler2015; Tarbiat et al., Reference Tarbiat, Jansson, Tydén and Höglund2016). At present, evidence of AR in chicken nematodes is scarce although the expansion of the free-range production system usually involving regular application of anthelmintics may further increase the risk of resistance (Tarbiat et al., Reference Tarbiat, Jansson, Tydén and Höglund2016; Collins et al., Reference Collins, Jordan, Bishop and Kaplan2021; Soudkolaei et al., Reference Soudkolaei, Kalidari and Borji2021; Feyera et al., Reference Feyera, Ruhnke, Sharpe, Elliott, Shifaw and Walkden-Brown2021a) et al. In order to delay the onset and spread of AR and ensure optimal use of the available anthelmintics, regular monitoring of the efficacy of anthelmintics is essential (Coles et al., Reference Coles, Jackson, Pomroy, Prichard, von Samson-Himmelstjerna, Silvestre, Taylor and Vercruysse2006; Demeler et al., Reference Demeler, Küttler and El-Abdellati2010).
In poultry, anthelmintic efficacy assessment is mainly based on the worm count reduction test using standardized procedures as detailed by Yazwinski et al. (Reference Yazwinski, Chapman, Davis, Letonja, Pote, Maes, Vercruysse and Jacobs2003). This in vivo method is expensive in terms of labour and cost of maintaining experimental birds in different treatment groups. If available, non-invasive and reliable in vitro assays comparable with in vivo assays offer substantial savings and welfare benefits (Dobson et al., Reference Dobson, Donald, Waller and Snowdon1986). Furthermore, it is also suggested that in vivo AR testing assays be supported by complementary in vitro tests in gastrointestinal nematodes (Coles et al., Reference Coles, Bauer, Borgsteede, Geerts, Klei, Taylor and Waller1992; Demeler et al., Reference Demeler, Kleinschmidt, Küttler, Koopmann and von Samson-Himmelstjerna2012). The egg hatch assay and larval development assay (LDA) are the most commonly used in vitro bioassays for monitoring AR in nematode species (Várady & Čorba, Reference Várady and Čorba1999; FAO, 2004; Albonico et al., Reference Albonico, Wright, Ramsan, Haji, Taylor, Savioli and Bickle2005). There are several modifications of these assays for the detection of AR to benzimidazoles (BZs), levamisole (LEV) and macrocyclic lactones largely in ruminant nematodes (Wagland et al., Reference Wagland, Jones, Hribar, Bendixsen and Emery1992; Várady & Čorba, Reference Várady and Čorba1999; Taylor et al., Reference Taylor, Hunt and Goodyear2002; Demeler et al., Reference Demeler, Küttler and El-Abdellati2010). These assays mainly use the pre-parasitic stages (egg or larvae) and measure efficacy in the form of inhibition of larval development, hatch, or motility. Thus, such assays are useful mainly in nematode parasites where hatched larvae, not embryonated eggs, are the infective stage in the lifecycle.
Unfortunately, the life cycle of ascarid nematodes of poultry renders the commonly used egg hatch assay and LDA unusable for most classes of anthelmintics (Zhao et al., Reference Zhao, Williams and Hansen2017). Embryonated eggs do not hatch spontaneously outside the chicken host and there is no free-living larval stage (Hansen et al., Reference Hansen, Terhaar and Turner1956; Rogers, Reference Rogers1961; Salih & Saleem, Reference Salih and Saleem1987), making it difficult to employ these methods. However, an in ovo LDA, a variant of LDA for ruminant nematodes, proved to be successful for detection of BZ resistance in A. galli (Tarbiat et al., Reference Tarbiat, Jansson, Tydén and Höglund2017). It measures the embryonic development of parasite eggs to third larval stage in the presence of increasing concentrations of anthelmintics. However, this assay cannot be used for anthelmintics that do not demonstrate ovicidal activity including LEV, piperazine (PIP) and macrocyclic lactones such as ivermectin.
Induced hatching of the embryonated eggs and in vitro maintenance of hatched larvae is a prerequisite for any in vitro larval assays developed for A. galli. Many factors, both chemical and physical, can induce hatching of infective ascarid eggs (Salih & Saleem, Reference Salih and Saleem1987; Han et al., Reference Han, Eriksen, Boes and Nansen2000). Several researchers have used artificial media for hatching A. galli eggs in vitro (Elliott, Reference Elliott1954; Hansen et al., Reference Hansen, Terhaar and Turner1956; Chatterjee & Singh, Reference Chatterjee and Singh1968; Dick et al., Reference Dick, Leland and Hansen1973; Feyera et al., Reference Feyera, Ruhnke, Sharpe, Elliott, Campbell and Walkden-Brown2020)et al.et al.et al.. The established methods include incubation of embryonated eggs with deshelling reagents including sodium hydroxide (NaOH) and sodium hypochlorite (NaOCl) followed by centrifugation to free the larvae. With these methods, the thick egg shell is digested leaving the contents enclosed in a thin membrane which is disrupted on centrifugation freeing the enclosed larvae (Hansen et al., Reference Hansen, Terhaar and Turner1956; Dick et al., Reference Dick, Leland and Hansen1973; Feyera et al., Reference Feyera, Ruhnke, Sharpe, Elliott, Campbell and Walkden-Brown2020). The existing larval hatching assays for A. galli need improvement in terms of hatching time, percentage yield and reduction of procedure-induced larval mortality. Reliable and time saving methods have been described for other ascarid nematodes that could be of value to overcome these drawbacks. The glass-bead hatching assay is one such method that employs mechanical shell disruption using glass beads coupled with magnetic stirring with or without stimulating agents such as bile, carbon dioxide (CO2), sodium chloride (NaCl), sulphur dioxide, and sodium bicarbonate (Han et al., Reference Han, Eriksen, Boes and Nansen2000).
Even though artificial larval hatching assays have been described for A. galli, the possibility of using artificially hatched A. galli larvae for anthelmintic sensitivity studies has not been investigated. Zhao et al. (Reference Zhao, Williams and Hansen2017) reported a larval migration assay using artificially hatched larvae of A. suum which allowed accurate estimation of effective concentration (EC50) values of BZ, tetrahydropyrimidin and imidazothiazole anthelmintics. This approach appears promising for the use of artificially hatched larvae of A. galli for in vitro efficacy evaluation of multiple classes of anthelmintics with different modes of action. The three commonly used anthelmintics classes in poultry, BZs, LEV and PIP exhibit different pharmacological effects on A. galli. The BZs disrupt energy metabolism whereas LEV and PIP cause worm paralysis (Lacey, Reference Lacey1988).
In vitro parasite assays require sufficient numbers of viable parasite stages especially eggs. For assays involving A. galli, eggs are most commonly obtained from mature worms either by disruption of the worm's uterus (Permin et al., Reference Permin, Bojesen, Nansen, Bisgaard, Frandsen and Pearman1997; Rahimian et al., Reference Rahimian, Gauly and Daş2016) or by in vitro culturing of female worms in artificial media and recovering eggs shed into the media (Sharma et al., Reference Sharma, Hunt, Hine, Sharma, Swick and Ruhnke2017; Reference Sharma, Hunt, Hine, Sharma, Swick and Ruhnke2018; Feyera et al., Reference Feyera, Ruhnke, Sharpe, Elliott, Campbell and Walkden-Brown2020). These approaches are not always feasible given the requirement of chicken necropsy to recover mature worms (Feyera et al., Reference Feyera, Ruhnke, Sharpe, Elliott, Campbell and Walkden-Brown2020). Excreta collected from chickens harbouring A. galli-specific infection can also serve as source of eggs (Rahimian et al., Reference Rahimian, Gauly and Daş2016; Tarbiat et al., Reference Tarbiat, Rahimian, Jansson, Halvarsson and Höglund2018) but methods for recovery of clean eggs from excreta have not been optimized. While a number of experimental studies have used A. galli eggs recovered from host excreta by sequential sieving and washing through a series of sieves (with gradually reducing mesh sizes, ranging from 1 mm to 36 μm) followed by flotation–centrifugation procedures, to the best of our knowledge, the effect of different flotation solutions on recovery efficiency and subsequent egg viability has not been thoroughly investigated to date. The most commonly used egg flotation fluids for coprological samples are magnesium sulphate (MgSO4), sucrose, NaCl, sodium nitrate, and zinc sulphate (ZnSO4) (Ballweber et al., Reference Ballweber, Beugnet, Marchiondo and Payne2014; Gibbons et al., Reference Gibbons, Dennis, Mark and Hansen2014). Most solutions are used at saturation or near-saturation and a specific gravity (SG) from 1.2 to 1.3 is best for separating most nematode eggs from faecal debris (Foreyt, Reference Foreyt2013). Saturated salt solutions have a tendency to crystalize rapidly, and cause significant distortion of eggs particularly at high SGs (David & Lindquist, Reference David and Lindquist1982; Ballweber et al., Reference Ballweber, Beugnet, Marchiondo and Payne2014). Additionally, the integrity of some parasite elements will vary in different solutions (Zajac & Conboy, Reference Zajac and Conboy2012). Sugar solution is recommended if the eggs are required for culturing as it is considered to have little effect on the egg viability (Gibbons et al., Reference Gibbons, Dennis, Mark and Hansen2014). A recent study (Daş et al., Reference Daş, Klauser, Stehr, Tuchscherer and Metges2020) reported that sugar solution increased A. galli egg recovery from excreta by approximately 10%, relative to the lower SG NaCl solution.
The purpose of the present study was, therefore, to adapt and evaluate in vitro drug exposure assays based on eggs or larvae of A. galli for testing the efficacy of different classes of anthelmintics. It also aimed to describe optimized non-invasive methods that would yield high numbers of minimally damaged parasite stages (eggs or larvae) for in vitro anthelmintic sensitivity assays or other uses. We hypothesized that in vitro drug sensitivity assays based on fresh excreta eggs or artificially hatched larvae would enable estimation of ECs (EC50/EC99) of different anthelmintics that could then be correlated with effective plasma drug concentrations or in vivo efficacy values. The specific propositions tested were: (i) use of saturated sugar solution as flotation fluid will allow recovery of higher numbers of morphologically normal and viable A. galli eggs from chicken excreta compared to saturated salt solutions; (ii) the in-ovo LDA will be a suitable test to estimate the EC50/EC99 of ovicidal BZ anthelmintics against A. galli eggs; (iii) the glass-bead larval hatching method will be superior to the chemical deshelling–centrifugation method in terms of hatching percentage and post-hatch survival rate of A. galli larvae; and (iv) LEV, PIP and BZ anthelmintics will exhibit inhibitory effects on the migration behaviour of artificially hatched A. galli larvae in a concentration-dependent fashion but have different EC50/EC99 values.
Materials and methods
Study design
This study consisted of two main parts. Part 1 involved optimization of methods that would yield high numbers of minimally damaged eggs or larvae for subsequent in vitro anthelmintic sensitivity assays. In Part 1a we evaluated different flotation solutions for recovery of A. galli eggs from excreta to determine the solution that would yield the highest numbers of morphologically normal and viable A. galli eggs for an in ovo LDA. In Part 1b we then compared two assays for artificially inducing egg hatching, a deshelling–centrifugation method (Dick et al., Reference Dick, Leland and Hansen1973; Feyera et al., Reference Feyera, Ruhnke, Sharpe, Elliott, Campbell and Walkden-Brown2020) and a glass-bead hatching method with or without bile (Han et al., Reference Han, Eriksen, Boes and Nansen2000) to select the best method for subsequent larval migration inhibition assay (LMIA). In Part 2, drug exposure assays were evaluated, using eggs or artificially hatched larvae of A. galli, to create concentration–response curves and determine the in vitro drug efficacy estimation values (EC50/EC99). In Part 2a an in ovo LDA was applied to fresh A. galli eggs recovered by an optimized technique in Part 1a following an assay procedure described earlier (Tarbiat et al., Reference Tarbiat, Jansson, Tydén and Höglund2017). In Part 2b a modification of a LMIA described for Ascaridia suum (Zhao et al., Reference Zhao, Williams and Hansen2017) was applied to A. galli larvae hatched by an optimized method as developed in Part 1b.
Parasite material and source
An A. galli isolate (UNE 2020-QLD-2), which was originally recovered from naturally infected laying hens on a commercial poultry farm in Queensland, Australia, with ‘no history of application of anthelmintics’, was employed. This isolate had undergone a single experimental passage in young cockerels (infected at day-old) in a previously reported experiment (Feyera et al., Reference Feyera, Sharpe, Elliott, Shifaw, Ruhnke and Walkden-Brown2021b). Eggs were obtained from fresh excreta samples collected from chickens in individual cages harbouring A. galli infection late in this experiment by placing paper sheets beneath the individual cages between approximately 5 pm in the afternoon and 10 am the next morning. The isolate had been shown in a separate study to have no evidence of resistance to LEV, PIP or flubendazole (Feyera et al., Reference Feyera, Sharpe, Elliott, Shifaw, Ruhnke and Walkden-Brown2021b).
Anthelmintics
The anthelmintics employed in this study were powder formulations of LEV, PIP, thiabendazole (TBZ), and fenbendazole (FBZ) (Sigma, St. Louis, USA). For both the LDA and LMIA assays, stock solutions of the drugs were prepared in 100% dimethyl sulfoxide (DMSO) while serial dilutions were carried out using 0.5 and 2% DMSO, respectively, for LDA and LMIA to achieve the final drug concentrations tested. The concentration ranges of the tested drugs were chosen based on plasma concentration values (including Cmax) reported for poultry or concentration ranges used in determining EC50 values of these compounds against other ascarid species (Hu et al., Reference Hu, Ellis, Yiu, Miller, Urban, Shi and Aroian2013; Tarbiat et al., Reference Tarbiat, Jansson, Tydén and Höglund2017; Zhao et al., Reference Zhao, Williams and Hansen2017; Scare et al., Reference Scare, Dini, Norris, Steuer, Scoggin, Gravatte, Howe, Slusarewicz and Nielsen2020). Accordingly, for the LDA only the ovicidal drugs TBZ and FBZ were used in two-fold serial dilution with final concentration ranges of 9.94 × 104–3.18 × 106 nM (0.02–0.64 μg/ml) for TBZ and 6.68 × 104–2.14 × 106 nM (0.02–0.64 μg/ml) for FBZ. For the LMIA all compounds were tested in a four-fold serial dilution providing final concentration ranges of 1.25–1280 nM (2.5 × 10−4–0.258 ng/ml) for TBZ, 0.125–128 nM (3.74 × 10−5–0.128 ng/ml) for FBZ, 2.5–2560 nM (5.1 × 10−4–0.523 ng/ml) for LEV, and 7.82 × 104–8.0 × 107 nM (6.73–6891 ng/ml) for PIP. Distilled water containing 0.5% and 2% DMSO, respectively, were used as negative control samples for the LDA and LMIA.
Optimization of methods for efficient isolation of eggs or larvae
Recovery of A. galli eggs from excreta (Part 1a)
Fresh excreta samples were collected from chickens harbouring A. galli artificial infection and kept in individual cages, for a separate study approved by the animal ethics committee of the University of New England, Australia (approval number AEC19−070). Fresh droppings were collected by placing paper sheets beneath the individual cages overnight. The excreta materials were pooled and thoroughly mixed using a glass rod in a plastic bucket for 5 min to obtain a homogenized mixture. Three sub-samples of 2.5 g were examined by a modified McMaster method using Whitlock egg counting chamber (Whitlock, Reference Whitlock1948) with a diagnostic sensitivity of 40 egg per gram of excreta (EPG) to obtain an estimate of the initial excreta egg counts (EECs).
Eggs were extracted from fresh excreta using a consistent procedure involving sequential washing with water through a series of sieves followed by flotation and centrifugation to obtain a clean concentrated egg sample (Daş et al., Reference Daş, Klauser, Stehr, Tuchscherer and Metges2020). From the homogenized excreta sample of known initial EPG, 5 replicates of 22.5 g of excreta for each flotation solution were subjected to the recovery procedure detailed below. The saturated flotation solutions used in this experiment and their corresponding SG were sucrose solution (Sheather's solution, SG 1.27), sucrose–NaCl solution (SG 1.28), NaCl (SG 1.2), MgSO4 (Epsom salts, SG 1.2) and ZnSO4 (SG 1.35).
Excreta samples were flushed with tap water and passed through a series of sieves with mesh aperture sizes of 1000, 500, 250 and 90 μm, with the eggs then collected on the final 36 μm sieve. Eggs were then washed off 36 μm sieve and cleaned and concentrated by flotation coupled with centrifugation. Briefly, the material retained on the 36 μm sieve was washed off and transferred to 50 ml conical centrifuge tubes. The tubes were then centrifuged at 1620 × g for 1 min and the supernatant was discarded leaving approximately 5 ml of the sieve content in the tubes. The tubes were then topped up with flotation solution, the contents mixed and then no more than 5 min later centrifuged at 1619 × g for 1 min. After centrifugation, the supernatant containing eggs was poured off through 36-μm sieve followed by several rinses with a large volume of deionized water. The washed eggs on the sieve were then recovered by rinsing and stored in water (boiled and cooled) at 4 °C for not more than two days before being used for subsequent steps. Recovery efficiency, morphological quality of eggs at the time of recovery and subsequent developmental ability of eggs were assessed as described below.
Recovery efficiency
For each flotation solution, egg recovery efficiency was calculated relative to the initial mean egg count in the excreta material. Total number of eggs recovered and concentrated from each sample was estimated by a modified McMaster method applying a diagnostic sensitivity of 40 EPG.

Morphological quality of eggs at the time of recovery
For each sample, 200–300 eggs were examined microscopically and classified as damaged or normal (intact) based on morphological keys described earlier (Feyera et al., Reference Feyera, Ruhnke, Sharpe, Elliott, Campbell and Walkden-Brown2020) and shown in fig. 1 using an Olympus BX40 microscope equipped with a digital Olympus DP50 camera (Nikon Corporation, Tokyo, Japan).

Fig. 1. Representative examples of the morphological quality of fresh Ascaridia galli eggs at the time of recovery from chicken excreta: (a) normal (intact); (b) damaged (undulating and irregular egg shell).
Developmental ability of eggs
For each sample, an estimated 500 eggs were placed in 1.5 ml Eppendorf tubes, the tubes topped up with 0.1 N sulphuric acid (H2SO4) and incubated aerobically at 26 °C in a digital incubator for 14 days. To ensure aerobic conditions during embryonation, tube lids were opened and samples were aerated manually for 5 min three times per week. After the incubation period, the percentage of embryonated eggs was assessed by microscopically examining the morphological characteristics of 100–200 eggs. The proportion of eggs at different stages of development (undeveloped, early development, vermiform and embryonated) or dead were recorded for each category as described previously (Feyera et al., Reference Feyera, Ruhnke, Sharpe, Elliott, Campbell and Walkden-Brown2020) and shown in fig. 2.

Fig. 2. Morphological characteristics of Ascaridia galli eggs at different developmental stages following 14 days of incubation at 26 °C (original magnification 200×): (a) undeveloped; (b–d) early development; (e and f) vermiform; (g) embryonated; and (h) dead.
Evaluation of artificial egg hatching methods (Part 1b)
In this part, a deshelling–centrifugation method and a glass-bead hatching method, were compared and where possible optimized to maximize hatching yield, hatched larval viability and temporal change in larval survivability over time. The eggs used were recovered from excreta as described above using the sugar flotation solution, then resuspended in 0.1 N H2SO4 and incubated aerobically at 26 °C for 6–8 weeks. To ensure aerobic conditions, samples were aerated manually by opening the lids and gentle agitation of tubes three occasions per week. Embryonation status was confirmed by light microscopy at 100× magnification prior to inclusion in the hatching assays.
For each hatching assay, five replicates of 2000 embryonated A. galli eggs suspended in hatching solutions in 5 ml Falcon tubes (2000 eggs per tube) were subjected to each of the egg hatching methods described below. Following hatching, live hatched larvae were separated from dead larvae and egg shell debris by active migration into sterile Hanks’ buffered salt solution (HBSS) at 38 °C using a Baermann apparatus equipped with 20 μm mesh (Han et al., Reference Han, Eriksen, Boes and Nansen2000). The live larvae in each replicate tube were then washed, counted and suspended in larval culture media (Roswell Park Memorial Institute (RPMI) 1640 supplemented with 2 mm L-glutamine, 100 U/ml penicillin and 100 lg/ml streptomycin) and incubated at 37 °C. To assess temporal change in post-hatch larval survivability, at least 50 larvae per replicate were counted at different time points (0, 12, 24, 36, 48, 60, 72, 84 and 96 h) and their viability determined using the methylene blue (Fronine Pty Ltd, Melbourne, Australia) exclusion method. Hatched larvae in solution were mixed 1:1 with a 1:10,000 solution of methylene blue and examined under light microscopy at 100× magnification as described previously (Feyera et al., Reference Feyera, Ruhnke, Sharpe, Elliott, Campbell and Walkden-Brown2020) and shown in fig. 3. Live larvae remained motile and unstained (fig. 3a), whereas dead larvae absorbed the methylene blue stain (fig. 3b) with uptake of dye indicating larval death and inactivation.
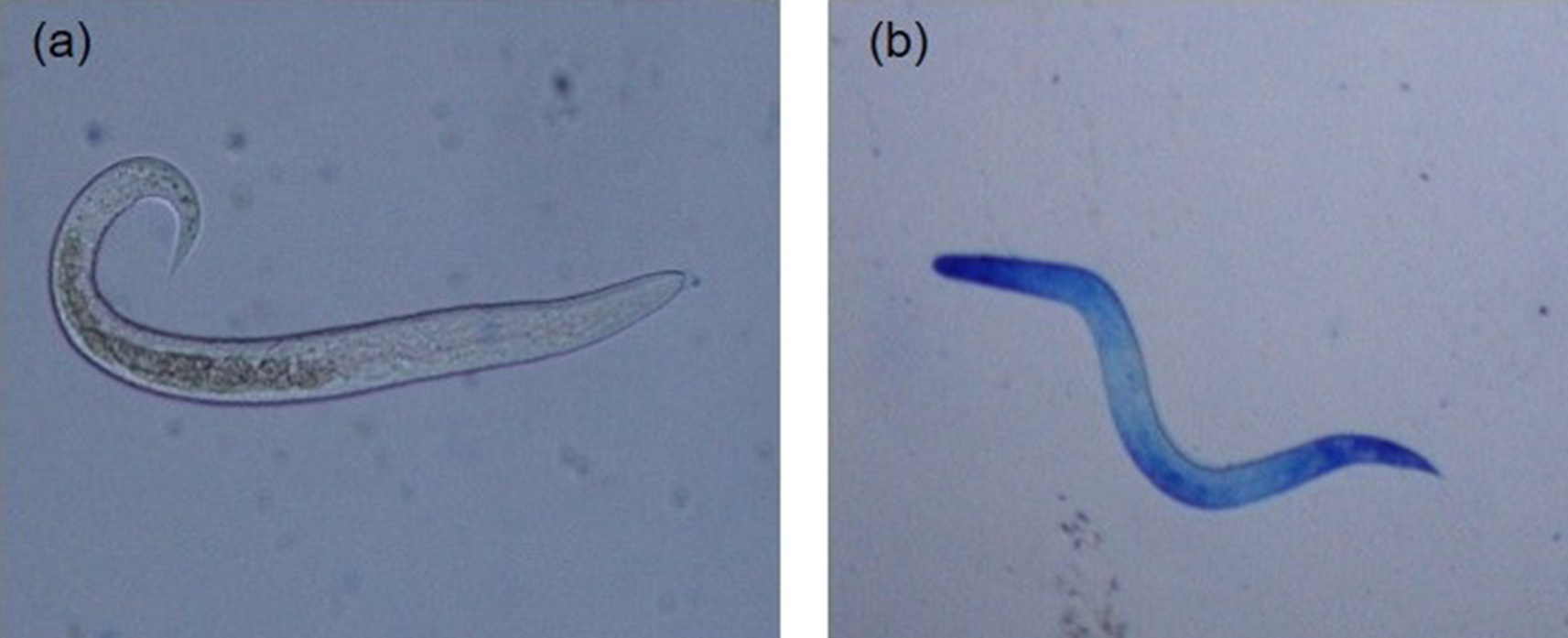
Fig. 3. Artificially hatched Ascaridia galli larvae stained with methylene blue (original magnification 200×): (a) live and active larvae demonstrating intact membrane and impermeability to methylene blue; and (b) dead larvae demonstrating uptake of methylene blue.
Method 1: deshelling–centrifugation method
A chemical–mechanical hatching assay originally developed for A. galli (Dick et al., Reference Dick, Leland and Hansen1973) and recently described by Feyera et al. (Reference Feyera, Ruhnke, Sharpe, Elliott, Campbell and Walkden-Brown2020) was used with slight modification. Briefly, eggs in 0.1 N H2SO4 were first washed thoroughly with 0.9% NaCl to remove the embryonation medium. Then, eggs were placed for 24 h at 26 °C in a solution containing equal parts of 4% NaOH and 4% NaOCl. After this treatment, the samples were incubated in 0.2% Tween-80 for 1 h and washed three times in distilled water by centrifugation. Liberation of the larvae was then achieved by centrifugation at 930 × g for 10 min. Live larvae were then separated from unhatched eggs and debris by migration into sterile HBSS using a Baermann apparatus equipped with 20 μm mesh. The larvae were then washed in sterile phosphate buffered saline (PBS), counted and suspended in larval culture media (RPMI 1640 supplemented with 2 mm L-glutamine, 100 U/ml penicillin and 100 lg/mL streptomycin) and stored aerobically at 37 °C until evaluated for larval viability at various times as described above.
Method 2: glass-bead hatching method
The glass-bead hatching method described for A. suum eggs (Han et al., Reference Han, Eriksen, Boes and Nansen2000; Zhao et al., Reference Zhao, Williams and Hansen2017) was adopted with slight modification. Briefly, eggs were washed thoroughly with 0.9% NaCl to remove the embryonation medium (0.1 N H2SO4). The eggs were then suspended in hatching buffer (HBSS with or without 5% bovine bile (Sigma, St. Louis, USA)) and hatched by stirring with 2 mm glass beads using a magnetic stirrer for 30 min at 37 °C. Live larvae were then separated from unhatched eggs and debris by migration into sterile HBSS using a Baermann apparatus equipped with 20 μm mesh. The larvae were then washed in sterile PBS, counted and suspended in larval culture media (RPMI 1640 supplemented with 2 mm L-glutamine, 100 U/ml penicillin and 100 lg/mL streptomycin) and stored aerobically at 37 °C until evaluated for larval viability at various times as described above.
Evaluation of in vitro drug exposure assays (Part 2)
LDA (Part 2a)
The LDA was conducted essentially as described previously (Tarbiat et al., Reference Tarbiat, Jansson, Tydén and Höglund2017). Fresh A. galli eggs recovered from excreta using the sugar solution were used. Briefly, the A. galli eggs were exposed to a series of gradually increasing drug concentrations in micro-titre plates with 5 replicates per interval for each anthelmintic. To prevent dehydration, each plate was covered with a plate sealer which allows passage of air molecules and placed in a water bath. The sealed plates were then incubated at 26 °C for two weeks to achieve optimum egg development rate as described previously (Feyera et al., Reference Feyera, Ruhnke, Sharpe, Elliott, Campbell and Walkden-Brown2020). At the end of the incubation period 10 μl of Lugol's iodine solution was added to each well and plates were examined under an inverted microscope. A minimum of 100 eggs per well were counted and the number of embryonated eggs at each drug concentration was determined as described above. Percentage inhibition of egg embryonation was calculated relative to the negative control using the formula (Wagland et al., Reference Wagland, Jones, Hribar, Bendixsen and Emery1992):

LMIA (Part 2b)
The LMIA was conducted essentially as described previously for A. suum (Williams et al., Reference Williams, Soelberg and Jäger2016, Zhao et al., Reference Zhao, Williams and Hansen2017), using A. galli larvae hatched by the deshelling–centrifugation method. Briefly, 100 larvae (in duplicate) were incubated with graded concentrations of anthelminthic drugs in 96 well plates with larval culture medium (RPMI 1640 supplemented with 2 mm L-glutamine, 100 U/ml penicillin and 100 lg/mL streptomycin) in each well. The plates were incubated overnight for 15–18 h at 38 °C in an atmosphere containing 5% CO2. Then, an equal amount of 1.5% agar solution (45 °C) was added to each well and mixed thoroughly. The agar was allowed to set prior to the addition of fresh culture media on top to cover the agar and the plates returned to the incubator overnight. The next day, the media containing larvae that had migrated from the setting agar was collected from each well and the number of larvae in the sample enumerated as described earlier (Williams et al., Reference Williams, Soelberg and Jäger2016). Percentage inhibition of migration was calculated relative to the negative control using the formula (Wagland et al., Reference Wagland, Jones, Hribar, Bendixsen and Emery1992):

Statistical analysis and determination of EC50 and EC99
The JMP® software version 16.0 (SAS Institute Inc., Cary, USA) was used for data analysis and creation of concentration–response curves. Distributions of the data and model residuals were assessed for compliance with the assumptions of analysis of variance (ANOVA). No data transformations were required except log transformation of drug concentrations for creation of concentration–response curves and determining EC50 and EC99. Data from egg recovery from excreta (including differences in egg recovery efficiency between different flotation solutions, morphological quality of eggs and subsequent developmental ability) and larval hatch assays (hatching efficiency) were analysed by one-way ANOVA using the fit least squares platform of JMP. Egg embryonation inhibition and larval migration inhibition were calculated relative to eggs or larvae recovered in control wells and expressed as percentage inhibition. Temporal changes in larval viability over time were analysed with repeated measures ANOVA using a mixed restricted maximum likelihood model with individual sample fitted as random factor and treatment and time and their interactions fitted as fixed effects. Nonlinear logistic regression (logistic 3P) was used to define the dose–response curve and calculate EC50, EC99 with 95% confidence limits. Statistical significance was set at P < 0.05 throughout.
Results
Recovery of A. galli eggs from excreta (Part 1a)
The type of flotation solution significantly (P = 0.003) affected the number of eggs recovered relative to the expected number of eggs contained in the excreta material. ZnSO4 (68.3%) and sucrose (67.8%) solutions provided the highest egg recovery efficiency followed by sucrose–NaCl solution (65.6%), while NaCl (58.3%) and MgSO4 (57.9%) extracted the least (fig. 4). There was a strong positive association between recovery efficiency and SG of the flotation solution (R 2 = 0.79; P = 0.03).

Fig. 4. Box plots of Ascaridia galli eggs recovery efficiency by different flotation fluids with lines from bottom to top representing the minimum, first quartile, median, third quartile and maximum values. Mean values of plots not sharing a common letter (a, b) differ significantly (P < 0.05).
The type of flotation solution also had a significant effect (P = 0.004) on the morphological quality of recovered eggs. The sugar solution yielded the highest proportion (98.1%) of morphologically normal eggs as assessed by morphological appearance whereas ZnSO4 resulted in the highest percentage of damaged eggs (9.7%) at the time of recovery compared to others (table 1). There was no major difference between the remaining flotation solutions in terms of the quality of eggs recovered.
Table 1. Morphological quality at the time of recovery and subsequent developmental ability of Ascaridia galli eggs recovered by different flotation solutions.

Values are mean ± SE; SE = standard error; SG = specific gravity. Means within columns not sharing a common letter (a, b) in the superscript differ significantly (P < 0.05) for each measurement and factor.
Furthermore, the type of flotation solution used to recover eggs from excreta had significant (P = 0.0074) effect on the subsequent developmental ability of A. galli eggs (table 1). Eggs isolated by sucrose solution had the highest embryonation capacity (93.3%) followed by those isolated by MgSO4 (87.1%). A positive linear association between morphological quality at the time of recovery and developmental ability of eggs was observed (n = 25; R 2 = 0.16; P = 0.047).
Combining the effects of flotation solution on efficiency of recovery and embryonation capacity an estimated yield of 63, 50, 49.7, 57 and 56% embryonated eggs from excreta could be expected following use of sucrose, MgSO4, NaCl, sucrose–NaCl and ZnSO4 as flotation solutions, respectively.
Evaluation of in vitro A. galli larvae hatching assays (Part 1b)
The deshelling–centrifugation and the glass-bead with 5% bile or without bile, respectively, resulted in 97.4, 95.2 and 94.9% hatching of the embryonated eggs with no statistically significant difference. Repeated measured analysis of post-hatch larvae survival data following incubation in RPMI media revealed significant effects of hatching method (P = 0.0053) and incubation period (P < 0.0001) without significant interaction between these effects (fig. 5). Overall, larvae hatched by the deshelling–centrifugation method demonstrated higher percentage survivability (P = 0.004) than those hatched by the glass-bead hatching assays. Larval survival decreased with incubation time with percentage survival diminishing to as low as <10–15% after 96 h of incubation almost in all cases with poor motility. This observation, however, employed a viability dye exclusion method and did not formally assess temporal change in the migratory behaviour (change in motility) of larvae over time.

Fig. 5. Analysis of temporal change in post-hatch survivability of artificially hatched Ascaridia galli larvae showing overall effects of method of hatching and incubation period in Roswell Park Memorial Institute media. Data are presented as least squares means with standard errors following statistical analysis. IP = incubation period.
LDA (Part 2a)
The mean percentage development to embryonation of eggs in the control wells was 92.5%. There was a concentration dependent inhibition of in ovo larval development for the two BZ drugs tested (fig. 6). EC50 and EC99 estimates are shown in table 2. FBZ had a slightly lower EC50 value (0.071 μg/ml) than TBZ (0.084 μg/ml).

Fig. 6. Concentration–response curves for TBZ (a) and FBZ (b) anthelmintics in a larval development assay for assessing anthelmintic efficacy in Ascaridia galli. TBZ = thiabendazole; FBZ = fenbendazole. Individual points represent replicates and the curve is a logistic 3P fit.
Table 2. EC50 and EC99 estimates (± standard error for log estimates) for inhibition of egg development to the embryonated stage based on a larval development assay of an Ascaridia galli isolate with no recent history of exposure to anthelmintics and known susceptibility to flubendazole.

TBZ = thiabendazole; FBZ = fenbendazole; EC = effective concentration.
LMIA (Part 2b)
As expected, the anthelmintics caused a concentration-dependent inhibition of larval migration (fig. 7). EC50 and EC99 values are shown in table 3. The concentration–response curve revealed that FBZ exhibited the lowest EC50 value (6.32 nM) whereas PIP the highest EC50 value (6.78 × 107 nM) with the EC99 estimates also following a similar trend.

Fig. 7. Concentration–response curves for different classes of anthelmintics in a larval migration inhibition assay for assessing anthelmintic efficacy in Ascaridia galli. (a) TBZ; (b) FBZ; (c) LEV; (d) PIP. TBZ = thiabendazle; FBZ = fenbendazole; LEV = levamisole; PIP = piperazine; individual points represent replicates and the curve is a logistic 3P fit.
Table 3. EC50 and EC99 estimates (± standard error for log estimates) for inhibition of larval migration in a larval migration inhibition assay using an Ascaridia galli isolate with no recent history of exposure to anthelmintics and known susceptibility to LEV and PIP.

TBZ = thiabendazle; FBZ = fenbendazole; LEV = levamisole; PIP = piperazine; EC = effective concentration. For PIP, the EC99 values are considerably outside the range of concentrations evaluated.
Discussion
The present study evaluated two in vitro drug exposure bioassays for anthelmintic efficacy testing against A. galli as well as the associated pre-assay sample preparation methods to maximize the yield of high-quality eggs and larvae for this purpose. It was found that the in-ovo LDA measuring inhibition of larval development in the egg shows considerable promise for the evaluation of efficacy of BZ anthelmintics against A. galli. This was evidenced by the clear concentration–response curves obtained enabling estimation of EC50/99 values of FBZ and TBZ. While the LDA method is restricted to anthelmintics with ovicidal activity, the more complex LMIA method evaluated also showed promise as a method for the creation of concentration–response curves enabling quantification of anthelmintic activity for anthelmintics acting on development stages beyond the egg. Among the flotation solutions tested, the saturated sucrose solution provided excellent recovery (67.8%) of fresh A. galli eggs from excreta with highest morphological quality (98.1%) and embryonation capacity (93.1%). The larval hatching assays evaluated did not differ in hatching efficiency but the deshelling–centrifugation method yielded larvae that had a better survival rate. These findings are discussed in more detail and in light of the original propositions below.
The first proposition of this study that sugar solution would allow better recovery of morphologically normal and viable A. galli eggs from excreta compared to saturated salt solutions was supported by the findings. Sucrose solution yielded a significantly higher number of minimally damaged eggs with superior developmental ability compared to the other flotation solutions tested. Taking into account all of the effects of flotation solution, the yield of fully embryonated eggs following extraction from excreta using sucrose, MgSO4, NaCl, sucrose–NaCl, and ZnSO4 and incubation in 0.1 N H2SO4 aerobically at 26 °C for 14 days would be 63, 50, 49.7, 57 and 56%, respectively. The sucrose solution thus resulted in an embryonated egg yield that is 7–13 percentage units higher than the other flotation fluids. As observed in other studies, the recovery efficiency of a flotation solution may in part be attributed to the SG of the flotation solution used (Cringoli et al., Reference Cringoli, Rinaldi, Veneziano, Capelli and Scala2004; Vadlejch et al., Reference Vadlejch, Petrtýl, Zaichenko, Čadková, Jankovská, Langrová and Moravec2011; et al. Reference Cringoli, Maurelli, Levecke, Bosco, Vercruysse, Utzinger and Rinaldi2017; Daş et al., Reference Daş, Klauser, Stehr, Tuchscherer and Metges2020) consistent with the positive association observed between SG and recovery efficiency in the present study. As noted by Ballweber et al. (Reference Ballweber, Beugnet, Marchiondo and Payne2014) the use of flotation solutions with high SG while favouring flotation of eggs, may simultaneously result in distortion of eggs. This was evident in the present study in the case of ZnSO4 which yielded the highest recovery rate due to its high SG, but lowest morphological quality and developmental ability as has also been reported previously (Amoah et al., Reference Amoah, Reddy and Stenström2017). Unlike the high SG salt solutions, the saturated sugar solution showed excellent recovery efficiency while also yielding eggs of highest morphological quality and subsequent developmental ability. This is consistent with literature that sugar solution should be used if the eggs are required for culturing as it is considered to have little effect on the egg viability (Gibbons et al., Reference Gibbons, Dennis, Mark and Hansen2014). While saturated NaCl is the most commonly used flotation solution in the laboratory, it yielded eggs of significantly inferior morphological quality as well as embryonation capacity compared to the sugar solution. In summary, the sugar solution can be recommended for recovering eggs from excreta for assays (such as LDA) when high numbers of fresh A. galli eggs with a high embryonation potential are required.
Our second proposition that the glass-bead method would result in a higher hatching percentage and post-hatch survival rate of A. galli larvae compared to the deshelling–centrifugation method was not supported by the findings. The three methods evaluated did not differ in hatching efficiency consistent with previous work reporting 96–98% for A. galli employing the deshelling–centrifugation method (Dick et al., Reference Dick, Leland and Hansen1973; Feyera et al., Reference Feyera, Ruhnke, Sharpe, Elliott, Campbell and Walkden-Brown2020) and 94–96% for A. suum using the glass-bead method (Han et al., Reference Han, Eriksen, Boes and Nansen2000). However, the deshelling–centrifugation method using Tween-80 in the deshelling solution yielded larvae with better post-hatch survival rates in RPMI media especially during the first 48 h post-hatch. The use of Tween-80 is considered advantageous not only to enable a high hatching rate, but also consistently high viability of larvae recovered (Dick et al., Reference Dick, Leland and Hansen1973) although the mechanism of these effects is unknown. The disadvantage of this method is that it involves egg hatching in two steps, that is, deshelling followed by liberation of larvae which is time consuming in comparison to the glass-bead method. The glass-bead method is a shorter procedure in terms of time required for hatching and is potentially useful if optimized but it did not provide any advantage over the former despite addition of bovine bile which was reported to enhance hatching, migration and survival of A. suum eggs depending on the method of hatching used (Han et al., Reference Han, Eriksen, Boes and Nansen2000). In the glass-bead method, the physical stimulus of magnetic stirring with glass beads is thought to be responsible for inducing egg hatching of A. suum, but in the present study also appeared to damage a proportion of the larvae thereby reducing their viability and subsequent ability to migrate as also reported previously (Han et al., Reference Han, Eriksen, Boes and Nansen2000; Vejzagić et al., Reference Vejzagić, Thamsborg, Kringel, Roepstorff, Bruun and Kapel2015). The post-hatch survival rate of newly hatched larvae observed in the current study is very low compared to earlier reports indicating larval survival up to 8–10 days in an ordinary culture media and as long as 112 days with in vitro development in enriched larval culture media at 38.5 °C (Dick et al., Reference Dick, Leland and Hansen1973). Overall larval viability decreased by 0.87% every hour of incubation in artificial media at 37 °C indicating a complete loss of viability by around 120 h. This suggests that incubation in RPMI at 37 °C may not be ideal for maintaining longevity of newly hatched larvae and requires that the larvae be used within 2–3 days of hatching for tests such as LMIA. It has been suggested that culture medium essential for the growth of several animal cell lines and containing most of the amino acids, vitamins and other metabolites are suited for in vitro maintenance of artificially hatched Ascaris larvae (Cleeland & Laurence, Reference Cleeland and Laurence1962). Regarding temperature, earlier studies involving in vitro cultivation of A. galli and Ascaris lumbricoides larvae used a culture temperature of 37–38 °C (Cleeland & Laurence, Reference Cleeland and Laurence1962; Dick et al., Reference Dick, Leland and Hansen1973) with larvae undergoing active exsheathing and development. The influence of incubating A. galli larvae at 37 °C (4.5 °C below the 41.5 °C body temperature of chickens) is unknown but cultivation of A. lumbricoides larvae at temperatures as low as 20–25 °C maximized survival time up to 100 days presumably due to lower metabolic activity (Cleeland & Laurence, Reference Cleeland and Laurence1962).
The third proposition that the in-ovo LDA would be a suitable method to estimate the EC50 of BZ anthelmintics for efficacy testing in A. galli was supported by the data. The evaluated LDA appears to be suitable for the creation of reliable concentration–response curves and calculation of EC50 allowing for assessment of AR for anthelmintics with ovicidal activity. The current LDA is in part a validation of a previous report by Tarbiat et al. (Reference Tarbiat, Jansson, Tydén and Höglund2017) who also found that this assay could be successfully used to assess the sensitivity of A. galli eggs to inhibition of development by TBZ. This technique is known to have high sensitivity, reproducibility and ease of interpretation (Várady et al., Reference Várady, Čudeková and Čorba2007). Given that the broad spectrum BZs will continue to be the most widely applied anthelmintics in the poultry industry worldwide and the LDA is a rather simple assay to perform, it should be considered as a method of choice for rapid in vitro AR testing in this class of anthelmintics compared to the more labour intensive LMIA. However, to estimate the resistance level in a worm population using this method, calibration of EC50 or EC99 results with gold standard measures of anthelmintic efficacy such as the worm count reduction test is required. As AR does not appear to be currently widespread in the poultry industry (Tarbiat et al., Reference Tarbiat, Jansson, Tydén and Höglund2017; Feyera et al., Reference Feyera, Ruhnke, Sharpe, Elliott, Shifaw and Walkden-Brown2021a, et al. Reference Feyera, Sharpe, Elliott, Shifaw, Ruhnke and Walkden-Brownb, et al. Reference Feyera, Shifaw, Sharpe, Elliott, Ruhnke and Walkden-Brown2022), finding A. galli isolates of known resistance status for such calibration is a substantial challenge. The EC50 value for TBZ obtained in the current study was slightly lower (0.084 μg/ml) compared to the previously reported EC50 value of 0.101–0.106 μg/ml (Tarbiat et al., Reference Tarbiat, Jansson, Tydén and Höglund2017) using drug sensitive A. galli isolates. A possible reason might be that the isolates used by Tarbiat et al. (Reference Tarbiat, Jansson, Tydén and Höglund2017) had a history of repeated exposure to BZ anthelmintics. It should be noted that the A. galli isolate used in our study originated from a commercial poultry farm with no history of anthelmintic use and shown to be fully susceptible to LEV, PIP and flubendazole (Feyera et al., Reference Feyera, Sharpe, Elliott, Shifaw, Ruhnke and Walkden-Brown2021b). The EC99 estimates, however, were slightly higher compared to the EC99 values of 0.33–0.35 μg/ml for TBZ reported by Tarbiat et al.et al. (Reference Tarbiat, Jansson, Tydén and Höglund2017). Identifying and testing of various resistant and susceptible isolates of A. galli will be necessary in order to calibrate EC50 and EC99 values against gold standard tests of AR to the BZ anthelmintics such as the worm count reduction test (Yazwinski et al., Reference Yazwinski, Chapman, Davis, Letonja, Pote, Maes, Vercruysse and Jacobs2003). The applicability of the LDA for mixed nematode species samples, which commonly occur in the field, also requires further evaluation, but it is likely that the ovicidal effects of BZ anthelmintics will extend to the other important nematode infections of chickens.
The fourth proposition of this study that LEV, PIP and BZ anthelmintics would exhibit inhibitory effects on the migration behaviour of artificially hatched A. galli larvae in a concentration-dependent fashion but would have different EC50/EC99 values was supported by the findings. Following exposure to anthelmintic drugs, the migration of A. galli larvae from agar gel was shown to be inhibited by anthelmintics in a dose-dependent manner. This is the first effort to develop such an assay using artificially hatched A. galli larvae and the concentration–response curves created for the tested anthelmintics showed that artificially hatched larvae of A. galli could be used for estimation of EC50 and thus in vitro AR testing using the LMIA provided calibration using strains of known AR status is done. This method is based on the nematode migration behaviour in the solidified agar gel, thereby allowing for counting of larvae that moved out of agar gel and quantifying the inhibitory effect of the nematode migration (Zhao et al., Reference Zhao, Williams and Hansen2017). Dead larvae or larvae whose migration is inhibited by anthelmintic drugs stay inside of the agar gel, only active larvae are able to migrate out of the agar gel (Williams et al., Reference Williams, Fryganas, Ramsay, Mueller-Harvey and Thamsborg2014; Zhao et al., Reference Zhao, Williams and Hansen2017). This assay allowed for assessment of anthelmintic activity simply by the counting of all migrated larvae rather than relying on assessment of individual worms as either motile or non-motile. The values of EC50 of TBZ, FBZ and LEV estimated in the current study are within the concentration ranges reported for the same compounds using LMIA against artificially hatched larvae of drug sensitive A. suum isolates which ranged 74–150, 4.9–13.9 and 358–1150 nM, respectively (Zhao et al., Reference Zhao, Williams and Hansen2017). PIP exhibited the highest EC50 values 190–10,000 fold higher than the other anthelmintics in the current study. Against adult ascarids the therapeutic dose of PIP (100 mg/kg) is 4-fold higher than that of LEV (28 mg/kg) and up to 20-fold higher than that of BZ anthelmintics such as FBZ (5–10 mg/kg). However, PIP has been shown to have poor efficacy against immature A. galli (Leiper, Reference Leiper1954; Horton-Smith & Long, Reference Horton-Smith and Long1956; Alicata, Reference Alicata1958; Feyera et al., Reference Feyera, Sharpe, Elliott, Shifaw, Ruhnke and Walkden-Brown2021b, et al., Reference Feyera, Shifaw, Sharpe, Elliott, Ruhnke and Walkden-Brown2022) and this appears to extend to freshly hatched larvae in the LMIA as the likely reason for the very much higher EC50 values observed for this anthelmintic. In summary, the current study showed that the LMIA, using artificially hatched A. galli larvae, could potentially be used for anthelmintic sensitivity studies against A. galli. However, this assay is methodologically complex to implement compared to the LDA and may not offer sufficient advantages over the gold standard worm count reduction test to warrant optimization for routine diagnosis of AR in A. galli. Its applicability for evaluating AR to PIP also requires further investigation given the selective efficacy of PIP for adult A. galli and low sensitivity of larval stages to its effects.
Conclusions
The current study provided strong confirmatory evidence that a LDA based on inhibition of A. galli egg embryonation will generate reliable concentration–response curves for the ovicidal BZ anthelmintics TBZ and FBZ. The LDA thus shows considerable potential for in vitro anthelmintic efficacy testing for BZ drugs, provided EC50 values can be calibrated against gold standard measures of efficacy such as the worm count reduction test. The LDA is an easy test to implement, but will not work for the anthelmintics which do not exhibit ovicidal activity. The advantages of this test are that it could be deployed on excreta samples sent in from a farm, and would not require animal testing and sacrifice. However, the applicability of the LDA for mixed species samples, which commonly occur in the field, requires further evaluation. The present study found that there are reasonable prospects for developing in vitro anthelmintic efficacy tests based on a LMIA that would be effective for evaluating the full range of anthelmintics, but the method is far more complex to implement than LDA and may not offer sufficient advantages over the worm count reduction test to warrant optimization. Its applicability for predicting the efficacy of PIP against adult worms is also uncertain, given the high level of resistance to its effects shown by larvae in this study. For in vitro studies, a saturated sugar solution is most appropriate for recovering eggs from excreta prior to use in assays. Further work is needed to optimize larval hatching/freeing methods, together with optimal temperature and media for maintenance of newly hatched larvae. Whatever in vitro tests are developed, standardization of assays and development of EC50 or EC99 reference values linked to known levels of anthelmintic resistance would also need to be achieved.
Acknowledgements
The authors thank Danielle Smith, Brian Cross and Craig Johnson, staff at the University of New England, Australia, for their technical support during the study.
Financial support
This research was funded by Australian Eggs Ltd. Project 1BS003. Teka Feyera was supported by a University of New England, Australia, international postgraduate scholarship.
Conflicts of interest
None.
Ethical standards
None.