For the majority of intensively reared carnivorous finfish species, such as salmonids, fishmeal (FM) is traditionally used as the protein source. While global aquaculture production has continued to grow, FM usage has remained static( 1 ). The limited supply of FM has led to increased interest in using plant proteins as alternatives to marine fishery-derived proteins. Nevertheless, inclusion of plant protein sources in aquafeeds is limited since a number of disadvantages have been recognised. Besides the negative effect on growth performance and intestinal function( Reference Van den Ingh, Krogdahl and Olli 2 – Reference Van den Ingh, Olli and Krogdahl 5 ), plant protein inclusion in fish feeds has been reported to be associated with decreased lipid digestibility, reduced bile salt levels and hypocholesterolaemia, suggesting an altered regulation of lipid metabolic pathways( Reference Olli, Krogdahl and Ingh 6 – Reference Geay, Ferraresso and Zambonino-Infante 13 ). The increased economical and quantitative importance of dietary plant protein sources in commercial fish feeds demands a better understanding of lipid metabolic processes such as absorption, transport and synthesis to improve feed utilisation and production cost optimisation.
The major lipid constituents of both natural and commercial salmonid diets are generally TAG with smaller amounts of phospholipids and cholesterol esters( Reference Henderson and Tocher 14 ). In monogastrics, TAG are hydrolysed in the intestinal lumen into NEFA (FA) and monoacylglycerols. Intracellularly, these hydrolysis products are carried by specific binding proteins to the endoplasmic reticulum, where they are resynthesised into TAG and incorporated into lipoproteins (LP). The formation of LP is mediated by the microsomal TAG transfer protein (MTP), which shuttles resynthesised TAG onto a newly formed apoB molecule. This primordial LP particle undergoes further lipidation with the addition of TAG and cholesterol esters by MTP, and phospholipids, apoAIV and, at a later stage, apoAI are added to the surface of the LP particle. This LP particle is then secreted by exocytosis via the basolateral membrane into the lymph( Reference Mansbach 15 , Reference Black 16 ). It has been suggested that the mechanism of lipid absorption and LP formation in fish intestine does not differ fundamentally from that in monogastric mammals( Reference Sire, Lutton and Vernier 17 , Reference Tocher 18 ). However, information is still lacking regarding the routes of lipid transport from the intestine. A lymphatic system has not been identified in fish yet.
Saponins are heat-stable glycosides present in legumes, such as soyabean, pea and lupin( Reference Shi, Arunasalam and Yeung 19 , Reference Krogdahl, Penn and Thorsen 20 ). Saponins, with their membrane-permeabilising activity( Reference Francis, Kerem and Makkar 21 ), are considered to be the factors responsible for the development of the distal intestine enteritis induced by soyabean meal in Atlantic salmon( Reference Knudsen, Uran and Arnous 22 , Reference Knudsen, Jutfelt and Sundh 23 ). Based on their detergent and surfactant properties( Reference Francis, Kerem and Makkar 21 ), dietary saponins probably disturb fat emulsification and micelle formation, but information necessary to understand their significance in lipid metabolism in fish is scarce. In general, saponins exhibit hypocholesterolaemic effects in a variety of animals( Reference Southon, Johnson and Gee 24 – Reference Al-Habori and Raman 28 ), but these effects may be dependent on interactions with other plant components( Reference Calvert and Blight 29 – Reference Sugano, Goto and Kimoto 31 ). It has also been noted that a saponin-induced reduction of serum cholesterol levels occurs only when an otherwise hypocholesterolaemic diet has been fed( Reference Jenkins and Atwal 32 ). It appears as if interactions between saponins and other components in plant meal are necessary for the effects on lipid metabolism, but information on these interactions is scarce.
The present study is a part of a larger experiment investigating the dose response of soya-saponin (SA) inclusion in plant meal- and FM-based diets at levels of 0, 2, 4, 6 and 10 g/kg. This part of the study aimed to understand how lipid absorption and metabolism are modulated by variation in protein source, saponin inclusion (10 g/kg), and the possible interaction between them in salmon feed. Intestinal and hepatic transcript levels of specific apo (apoAI, apoAIV and apoB), key enzymes (mtp, acyl-CoA cholesterol acyltransferase (acat), monoacylglycerol acyltransferase (mgat), choline kinase (chk) and choline-phosphate cytidylyltransferase A (pcyt1a)) and FA transporters (cluster of differentiation 36 (cd36), fatty acid transport protein (fatp) and fatty acid-binding protein 2 (fabp2)) were quantified using quantitative real-time PCR. We also quantified the hepatic expression of key genes involved in cholesterol and bile salt metabolism as described previously( Reference Kortner, Gu and Krogdahl 33 ). Total bile salt levels in the plasma and intestinal contents as well as plasma cholesterol levels were measured. Histomorphological alterations in the pyloric caeca and liver after dietary treatments were evaluated. Our hypothesis was that plant meal and SA inclusion in salmon feed would cause alterations in the expression levels of genes involved in lipid and sterol metabolism and that the differential expression would be reflected in alterations in blood plasma sterol levels and tissue lipid accumulation.
Materials and methods
Feed ingredients and diet formulation
SA (95 % concentrate) was obtained from Organic Technologies. FM, lupin meal and wheat gluten meal were used as primary protein sources. Fish oil and wheat meal were used as lipid and carbohydrate sources, respectively. For the present experiment, two basal diets were formulated: a FM-based diet (FM diet) with 587 g/kg FM and a plant meal-based diet (PM diet) with 60 % substitution of FM (235 g/kg FM, 200 g/kg lupin meal and 190 g/kg wheat gluten meal). The diets were formulated to be isoenergetic (24 MJ/kg gross energy). The fish investigated in the present study were fed one of the basal diets or diets produced from the basal diets supplemented with 10 g SA/kg. This saponin level is supposed to correspond to the level present in a diet in which a saponin-rich soyabean meal provides all the dietary protein. All diets were supplemented with standard vitamins and minerals to meet the requirements( 34 ). The diets were produced by Nofima AS, with a pellet size of 5 mm. Detailed diet formulations are given in Table 1.
Table 1 Formulation of the experimental diets
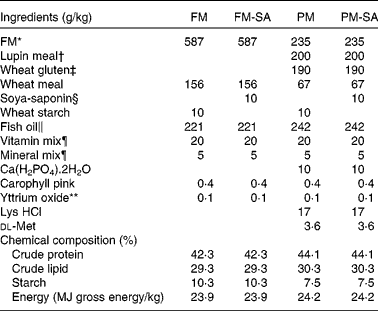
FM, fish meal; FM-SA, fish meal with soya-saponin; PM, plant meal; PM-SA, plant meal with soya-saponin.
* Fishmeal 122/11, LTQ fishmeal, produced by Welcon AS.
† Supplied by Holtermann AS.
‡ Wheat gluten 159/10, Amytex 100, produced by Syral.
§ 95 % Concentrate, supplied by Organic Technologies.
∥ Fishoil O5/10, NorSalmOil, produced by Norsildmel.
¶ Supplemented to meet the requirements.
** Marker for the evaluation of nutrient digestibility.
Experimental animals and design
The feeding trial was carried out at the Nofima Marine research station at Sunndalsøra (Norway) and in compliance with laws regulating experimentation with live animals in Norway, as overseen by the Norwegian Animal Research Authority (Forsøksdyrutvalget). The experiment was carried out using a 2 × 2 factorial design with four dietary treatments. Furthermore, two factors, PM inclusion and SA inclusion, were tested separately and in combination. Post-smolt Atlantic salmon (Salmo salar L.) of the Sunndalsøra strain with an initial weight of 442 (sem 4) g were allocated to eight 1 m3 fibreglass tanks, twenty-two fish per tank, with 250 litres of seawater flowing at a rate of 20 litres/min. Fish present in duplicate tanks were fed one of the four experimental diets. During the feeding trial, water temperature was decreased from 12 to 9°C. The oxygen content and salinity of the outlet water were monitored. Salinity ranged between 32 and 33 g/l. Fish were continuously fed by automatic disc feeders. A 24 h lighting regimen was employed during the experimental period.
Sampling
Sampling was conducted following 10 weeks of feeding the experimental diets. Randomly selected fish were anaesthetised with tricaine methanesulphonate (MS222; Argent Chemical Laboratories) and subsequently killed by a sharp blow to the head. Only the fish that had digesta throughout the intestinal tract were sampled to ensure intestinal exposure to the diets. The intestines were cleared of all fatty tissue and intestinal content before the collection of tissue samples. Pyloric caecal and liver tissues were sampled from four fish from each tank for mRNA extraction, placed in RNAlater (Ambion) at 4°C for 24 h and then stored at − 20°C. Blood (four fish per tank) was collected in heparinised vacutainers for plasma preparation. Intestinal contents (four fish per tank) from the pyloric intestine, mid-intestine and distal intestine were frozen in liquid N2 and then stored at − 80°C. Pyloric caecal and liver tissues (six fish per tank) were sampled for histological evaluation, placed in 4 % phosphate-buffered formaldehyde solution for 24 h and subsequently stored in 70 % ethanol until further processing.
Quantitative real-time PCR
RNA purification and quality control, DNase treatment, complementary DNA synthesis, primer optimisation and quantitative PCR assays were carried out as described in detail elsewhere( Reference Kortner, Valen and Kortner 35 ). Quantitative PCR primers were obtained from the literature or designed using Primer3 (http://frodo.wi.mit.edu/primer3). The details of the primers are given in Table 2. Elongation factor 1α (ef1a), β-actin (actb), RNA polymerase II (rnapolII), hypoxanthine phosphoribosyltransferase 1 (hprt1), ribosomal protein S20 (rps20) and glyceraldehyde-3-phosphate dehydrogenase (gapdh) were evaluated for use as reference genes by ranking the expression levels according to their stability, as described previously( Reference Kortner, Valen and Kortner 35 ). For pyloric caecal and liver samples, rps20 was used as the normalisation factor. The mean normalised expression of the target genes was calculated from raw quantification cycle (Cq) values( 36 ).
Table 2 Primer pair sequences, efficiency, amplicon size and annealing temperature for the genes* used for real-time PCR
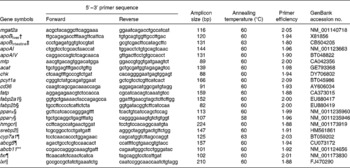
mgat2a, monoacylglycerol acyltransferase 2-A; mtp, microsomal TAG transfer protein; acat, acyl-CoA cholesterol acyltransferase; chk, choline kinase; pcyt1a, choline-phosphate cytidylyltransferase 1A; cd36, cluster of differentiation 36; fatp, fatty acid transport protein; fabp2a1/fabp2b, fatty acid-binding protein 2 isoforms; pparα/pparγ, PPAR isoforms; hmgcr, 3-hydroxy-3-methylglutaryl-CoA reductase; srebp2, sterol regulatory element-binding protein 2; cyp7a1, cytochrome P450 7A1; abcg5, ATP-binding cassette G5; abcb11, ATP-binding cassette B11; fxr, farnesoid X receptor; lxr, liver X receptor.
* Readers can refer to a previous study( Reference Kortner, Valen and Kortner 35 ) for reference gene primer details.
† Primers obtained from Torstensen et al. ( Reference Torstensen, Espe and Stubhaug 77 ). High expression was found in the liver and very low expression in the pyloric caeca.
‡ Primers designed in 5′ end of apoB transcript. High expression was found in the pyloric caeca.
§ Primers obtained from Venold et al. ( Reference Venold, Penn and Thorsen 78 ).
∥ Primers obtained from Minghetti et al. ( Reference Minghetti, Leaver and Tocher 79 ).
¶ Primers obtained from Kortner et al. ( Reference Kortner, Gu and Krogdahl 33 ).
** Primers obtained from Zaja et al. ( Reference Zaja, Munić and Klobucar 80 ).
Histology
From each treatment group, twelve fish were used for histological evaluation. Pyloric caecal and liver samples were dehydrated in ethanol, equilibrated in xylene, embedded in paraffin and sectioned (5 μm) according to standard histological techniques. Tissues were stained with haematoxylin and eosin. Blinded histological examination was carried out using a light microscope by paying particular attention to (presumed) lipid accumulation (steatosis) within enterocytes and hepatocytes. Steatosis was assessed using a categorical scoring system with grades of absent, slight, moderate and marked (Fig. 1).
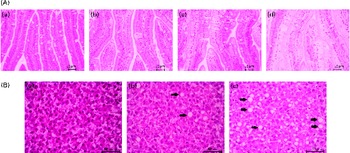
Fig. 1 Severity of steatosis of the pyloric (A) caeca and (B) liver of fish fed the experimental diets, representative for (a) absent (normal), (b) slight, (c) moderate and (d) marked. , Large vacuoles of TAG fat. (A colour version of this figure can be found online at http://www.journals.cambridge.org/bjn).
Cholesterol and bile salt analyses
Plasma cholesterol and bile salt concentrations, as well as bile salt concentrations in the intestinal contents, were determined as described previously( Reference Sørensen, Penn and El-Mowafi 12 ). All analyses were carried out on Advia® 1800 (Siemens Healthcare Diagnostics) at the Central Laboratory of the Norwegian School of Veterinary Science, Oslo, Norway.
Statistical analyses
Statistical analyses were carried out using JMP 10 (SAS Institute, Inc.). PM inclusion and SA inclusion were evaluated as class variables in a two-way ANOVA with interaction. For the histological evaluation of intestinal and liver samples, results were compared using a χ2 test. The level of significance was set at P< 0·05.
Results
Growth performance
Growth performance was significantly affected by PM inclusion, but not by SA inclusion or the interaction between them (Fig. 2(A); Table 3). Feeding the PM-based diets resulted in significantly decreased final weight and specific growth rate (SGR) compared with feeding the FM diets by 24·8 and 20·7 %, respectively.
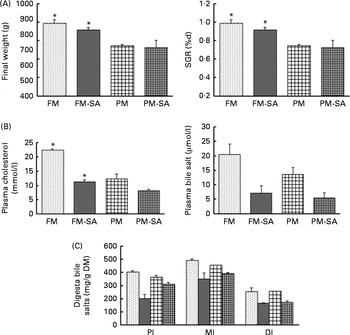
Fig. 2 Growth performance (A), concentrations of blood plasma cholesterol and bile salts (B) and concentrations of bile salts in digesta (C) of fish fed the experimental diets: fish meal (FM, ); fish meal with soya-saponin (FM-SA;
); plant meal (PM;
); plant meal with soya-saponin (PM-SA;
). Values are means, with standard errors represented by vertical bars. * Mean values were significantly different from those for plant meal inclusion (P< 0·05; two-way ANOVA). For plasma cholesterol, plasma bile salts and digesta bile salts, there was a significant effect for soya-saponin inclusion (P< 0·05; two-way ANOVA). For plasma cholesterol, there was a significant interaction between plant meal and soya-saponin inclusion (P< 0·05; two-way ANOVA). SGR, specific growth rate; PI, pyloric intestine; MI, mid-intestine; DI, distal intestine.
Table 3 Two-way ANOVA of the data for growth, cholesterol and bile salt levels, and gene expression of fish fed the experimental diets
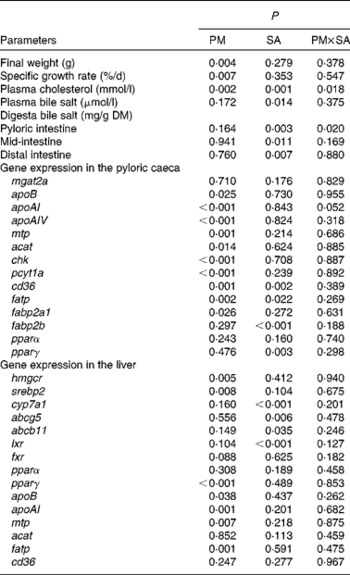
PM, plant meal; SA, soya-saponin; PM × SA, interaction between plant meal and soya-saponins; mgat2a, monoacylglycerol acyltransferase 2-A; mtp, microsomal TAG transfer protein; acat, acyl-CoA cholesterol acyltransferase; chk, choline kinase; pcyt1a, choline-phosphate cytidylyltransferase 1A; cd36, cluster of differentiation 36; fatp, fatty acid transport protein; fabp2a1/fabp2b, fatty acid-binding protein 2 isoforms; pparα/pparγ, PPAR isoforms; hmgcr, 3-hydroxy-3-methylglutaryl-CoA reductase; srebp2, sterol regulatory element-binding protein 2; cyp7a1, cytochrome P450 7A1; abcg5, ATP-binding cassette G5; abcb11, ATP-binding cassette B11; lxr, liver X receptor; fxr, farnesoid X receptor.
Histology
Lipid droplet accumulation in pyloric caecal enterocytes was significantly affected by PM inclusion (Table 4). Feeding the PM diets resulted in increased lipid droplet accumulation in enterocytes compared with feeding the FM diets. Similarly, the frequency of hepatic steatosis was significantly affected by PM inclusion (Table 4). Whereas none of the sampled fish fed the FM diets exhibited steatosis in the liver, several fish fed the PM diets exhibited slight-to-moderate hepatic steatosis. No significant interaction between PM inclusion and SA inclusion was observed.
Table 4 Severity of steatosis of the pyloric caeca and liver of fish fed the experimental diets*
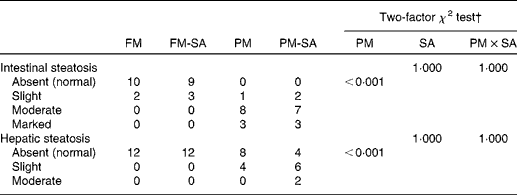
FM, fish meal; FM-SA, fish meal with soya-saponin; PM, plant meal; PM-SA, plant meal with soya-saponin; PM × SA, interaction between plant meal inclusion and soya-saponin inclusion.
* The numbers represent the number of fish. In each treatment group, twelve fish were used for histological evaluation.
† The statistical analyses pertain to all the data for each tissue.
Cholesterol and bile salt levels
Blood plasma cholesterol levels were significantly affected by both PM inclusion and SA inclusion (Fig. 2(B); Table 3). PM and/or SA supplementation significantly decreased blood plasma cholesterol levels. Moreover, a significant interaction between PM inclusion and SA inclusion was observed for this variable. The magnitude of the hypocholesterolaemic effects of SA on fish fed the PM-based diets was not as large as that of the effects on fish fed the FM diets. Plasma bile salt levels of fish were significantly decreased by SA inclusion. No effect of PM inclusion and no interaction between the saponin and dietary treatments were observed (Fig. 2(B); Table 3).
Digesta bile salt levels in the intestinal sections were significantly reduced by SA inclusion but not by PM inclusion (Fig. 2(C); Table 3).
Gene expression profiling
As shown in Fig. 3 and Table 3, PM inclusion had significant effects on pyloric caecal expression of key genes involved in lipid metabolism. Generally, PM inclusion induced the up-regulation of the expression of genes involved in LP assembly (apoB, apoAI, apoAIV, mtp, acat, chk and pcyt1a) and FA transport (cd36, fatp and fabp2a1). SA inclusion up-regulated the expression of genes encoding FA transporters (cd36, fatp and fabp2b) as well as the associated transcription factor pparγ. Neither PM inclusion nor SA inclusion changed the expression of mgat2a.
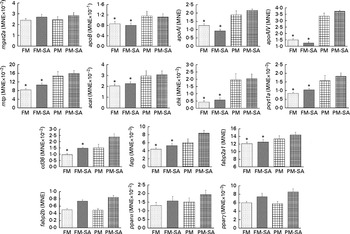
Fig. 3 Pyloric caecal gene expression of monoacylglycerol acyltransferase 2-A (mgat2a), apoB, apoAI, apoAIV, microsomal TAG transfer protein (mtp), acyl-CoA cholesterol acyltransferase (acat), choline kinase (chk), choline-phosphate cytidylyltransferase 1A (pcyt1a), cluster of differentiation 36 (cd36), fatty acid transport protein (fatp), fatty acid-binding protein 2 isoforms (fabp2a1/fabp2b) and PPAR isoforms (pparα/pparγ). Values are means, with standard errors represented by vertical bars. * Mean values were significantly different from those for plant meal inclusion (P< 0·05; two-way ANOVA). For cd36, fatp, fabp2b and pparγ, there was a significant effect for soya-saponin inclusion (P< 0·05; two-way ANOVA). FM, Fish meal (); FM-SA, fish meal with soya-saponin (
); PM, plant meal (
); PM-SA, plant meal with soya-saponin (
); MNE, mean normalised expression.
In the liver (Fig. 4; Table 3), similar to the results observed in the pyloric caeca, PM inclusion resulted in the up-regulation of the expression of genes involved in LP synthesis (apoB, apoAI and mtp) and fatty acid transport (fatp). PM inclusion had significant effects on the expression of genes involved in cholesterol metabolism. PM inclusion resulted in the up-regulation of the expression of 3-hydroxy-3-methylglutaryl-CoA reductase (hmgcr) as well as the associated transcription factors (sterol regulatory element-binding protein 2 (srebp2) and pparγ). SA inclusion had significant effects on the expression of genes involved in bile acid metabolism. Feeding SA-supplemented diets resulted in 11·5- and 8·3-fold down-regulation of the expression of cytochrome P450 7A1 (cyp7a1) compared with feeding the FM and PM diets, respectively. In parallel, SA supplementation resulted in reduced expression levels of liver X receptor (lxr), bile acid transporter (ATP-binding cassette B11 (abcb11)) and cholesterol transporter (ATP-binding cassette G5 (abcg5)).
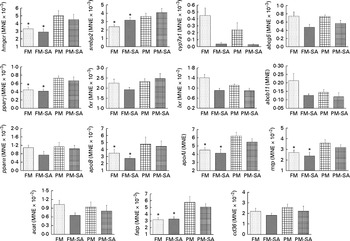
Fig. 4 Hepatic gene expression of 3-hydroxy-3-methylglutaryl-CoA reductase (hmgcr), sterol regulatory element-binding protein 2 (srebp2), cytochrome P450 7A1 (cyp7a1), ATP-binding cassette G5 and B11 (abcg5 and abcb11), liver X receptor (lxr), farnesoid X receptor (fxr), PPAR isoforms (pparα/pparγ), apoB, apoAI, microsomal TAG transfer protein (mtp), acyl-CoA cholesterol acyltransferase (acat), fatty acid transport protein (fatp) and cluster of differentiation 36 (cd36). Values are means, with standard errors represented by vertical bars. * Mean values were significantly different from those for plant meal inclusion (P< 0·05; two-way ANOVA). For cyp7a1, abcg5, lxr and abcb11, there was a significant effect for soya-saponin inclusion (P< 0·05; two-way ANOVA). FM, fish meal (); FM-SA, fish meal with soya-saponin (
); PM, plant meal (
); PM-SA, plant meal with soya-saponin (
); MNE, mean normalised expression.
Discussion
Effects of plant meal inclusion
In the present study, dietary inclusion of PM reduced growth performance, induced lipid droplet accumulation in the pyloric caeca and liver, and decreased plasma cholesterol levels in Atlantic salmon. The reduction of growth performance by PM inclusion is in accordance with several previous studies in Atlantic salmon( Reference Van den Ingh, Krogdahl and Olli 2 , Reference Olli, Krogdahl and Ingh 6 , Reference Olli and Krogdahl 37 ).
The present study indicates that the capacity for intestinal LP assembly was up-regulated in the PM diet-fed fish, probably as a result of excessive lipid droplet accumulation. Interestingly, the expression of mgat2a, which is responsible for TAG re-esterification, remained unaffected by PM inclusion. In accordance with this observation, a number of studies have demonstrated that the accumulation of large lipid droplets (mainly consisting of TAG) in the intestine of fish is not due to the increased TAG re-esterification but rather due to lipid export rate reduction( Reference Fontagné, Geurden and Escaffre 38 – Reference Daprà, Geurden and Corraze 42 ). Therefore, the excessive lipid droplet accumulation observed in the present study, most probably, was a result of reduced lipid export from the intestinal mucosa to the circulatory system. The reason for this is not clear; however, deficient amounts of one or more of the major constituents of the polar LP surface, such as phosphatidylcholine (PC), apo and cholesterol, are probably responsible for this. In support of this, it has been shown that dietary supplementation with PC prevents intestinal steatosis in common carp larvae( Reference Fontagné, Geurden and Escaffre 38 ).
In mammalian systems, molecular regulation of lipid absorption and transport from the intestine has been studied extensively( Reference Mansbach 15 , Reference Black 16 , Reference Iqbal and Hussain 43 , Reference Niot, Poirier and Tran 44 ). The present study reveals novel information on the regulation of lipid absorption and transport in a piscine model, which is assumed to be generally similar to that in mammals( Reference Tocher 18 , Reference Tocher, Bendiksen and Campbell 45 , 46 ). A summary of the proposed regulation is shown in Fig. 5. Dietary lipids are exported from the intestine in the form of large, neutral lipid-rich LP. LP production in the enterocytes involves a series of biosynthetic processes that results in the formation of a large hydrophobic core surrounded by a thin coat of phospholipids (mainly PC), cholesterol and apo( Reference Iqbal and Hussain 43 ). Clearly, phospholipids, cholesterol and apo are required for LP assembly. The positive effects of intestinal PC on LP formation have been demonstrated in mammals( Reference O'Doherty, Kakis and Kuksis 47 – Reference Wang, Du and Lu 49 ). In fish, the ability of the enterocytes to export lipids may be limited by the absence of dietary PC( Reference Olsen, Dragnes and Myklebust 41 , Reference Daprà, Geurden and Corraze 42 ). As in mammals, de novo PC synthesis in fish occurs primarily via the cytidine diphosphate (CDP)-choline pathway with choline kinase producing phosphocholine recognised as the committing enzyme, followed by phosphocholine cytidylyltransferase producing CDP-choline, recognised as the rate-limiting and rate-regulatory enzyme( Reference Tocher, Bendiksen and Campbell 45 ). In the present study, the expression of both chk and pycta1 was up-regulated in the PM diet-fed fish, indicating that the capacity for PC synthesis from choline was increased. PM inclusion also induced the up-regulation of the expression of genes encoding the apoB, apoAI and apoAIV, and an increased capacity for cholesterol esterification was indicated by the increased acat levels. In mammals, the increased mRNA expression of mtp in the intestine has been considered to be a response to excessive lipid droplet accumulation( Reference Black 16 ). The present study is in line with these studies, showing an up-regulation of the expression of mtp in the pyloric caeca when steatosis was observed. In parallel to the overall transcriptional induction of LP synthesis, the expression of genes involved in FA transport was also induced, showing an up-regulation of the FA transporters cd36 and fatp, as well as fabp2a1, which is responsible for FA transport from the cytoplasm to the endoplasmic reticulum( Reference Field and Mathur 48 ). In FA transporter knockout animals, lipids tend to accumulate in the proximal small intestine primarily because of the decreased transport of FA targeted for transport to the circulatory system through their use in the assembly of LP( Reference Drover, Ajmal and Nassir 50 ). However, it is likely that an efficient FA uptake from the intestinal lumen requires both spontaneous diffusion and protein-mediated transfer, and the relative importance of these two mechanisms is probably highly dependent on the microenvironment and tissue phenotype( Reference Niot, Poirier and Tran 44 ). Altogether the present results suggest that PM inclusion up-regulated the capacity for LP synthesis and FA transport. The correlation between induced gene expression and increased lipid droplet accumulation suggests an induction of regulatory mechanisms to compensate for the reduced lipid export rate.
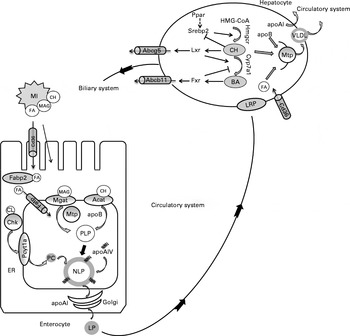
Fig. 5 Proposed molecular regulation of lipoprotein (LP) assembly, and cholesterol (CH) and bile acid (BA) metabolism based on data from the present study and previous studies in fish and mammalian species. In the intestinal lumen, products of lipid hydrolysis are solubilised in micelles (MI) and presented to the apical membrane of enterocytes. Fatty acids (FA) are transported from the intestinal lumen into the enterocytes by protein transporters including Cd36 (cluster of differentiation 36) and fatty acid transport protein (Fatp) or by protein-independent diffusion. Within the cytoplasm, FA are shuttled by fatty acid-binding protein 2 (Fabp2). Acyl-CoA cholesterol acyltransferase (Acat) and monoacylglycerolacyltransferase (Mgat) are located in the endoplasmic reticulum (ER) membrane, where they facilitate the esterification of CH and monoacylglycerols (MAG), respectively. These esterified products are assembled into proximal lipoproteins (PLP) with apoB in a microsomal TAG transfer protein (Mtp)-dependent manner. The PLP is merged with apoAIV to form a nascent lipoprotein (NLP) by core expansion. Choline kinase (Chk) and choline-phosphate cytidylyltransferase (Pcyt1a) are the committed and rate-limiting enzymes in the synthesis of phosphatidylcholine (PC) from choline (CL). PC is used in the formation of the polar lipid coat of NLP. Within the Golgi lumen, apoAI is attached to NLP to form a mature LP. The LP is released from the enterocyte to enter the circulatory system. LP are transported to the liver, in fish supposedly via the portal veins, and recognised by LP receptor-related proteins (LRP). In hepatocytes, the intestinal LP are disassembled and new LP, i.e. VLDL, are formed. apoB and Mtp participate in the formation of VLDL. apoAI is also secreted by the liver. CH and primary BA are the major constituents of bile that are synthesised in the liver and released into the biliary duct via the specific membrane transporters ATP-binding cassette G5 and B11 (Abcg5 and Abcb11), respectively. Both CH and BA are stored in the gall bladder and released into the intestine upon ingestion of feed. In the hepatocytes, sterol regulatory element-binding protein 2 (Srebp2) up-regulates the expression of genes involved in CH synthesis (3-hydroxy-3-methyl-glutaryl-CoA reductase (hmgcr)). Liver X receptor (Lxr) is activated by oxysterols, and it up-regulates the expression of genes involved in CH catabolism (cytochrome P4507A1 (cyp7a1)) and transport (abcg5). Farnesoid X receptor (Fxr) is activated by BA and controls intracellular BA levels by the regulation of Cyp7a1. PPAR isoforms (Ppar) are associated with the regulation of CH and BA metabolism, possibly by interaction with Srebp2. , Activation;
, inhibition;
, possible interaction.
In line with our observations in the pyloric caeca, PM treatment resulted in hepatic fatty change and increased levels of genes (apoB, apoAI and mtp) encoding key proteins involved in LP synthesis (mainly VLDL). These results suggest that similar regulatory mechanisms may exist in both the intestine and liver of fish fed the PM diets to compensate for the reduced lipid export rate. However, the increased lipid droplet accumulation in the liver of fish fed the PM may also result from an increase in lipolysis from adipose tissue( Reference Albalat, Gutierrez and Navarro 51 – Reference Bouraoui, Sánchez-Gurmaches and Cruz-Garcia 53 ).
Gene expression profiling also indicated that the capacity for cholesterol synthesis was up-regulated in the PM diet-fed fish. This is in accordance with several previous studies( Reference Geay, Ferraresso and Zambonino-Infante 13 , Reference Kortner, Gu and Krogdahl 33 , Reference Leaver, Villeneuve and Obach 54 – Reference Panserat, Hortopan and Plagnes-Juan 56 ) and could reflect a general need for de novo cholesterol synthesis in PM-fed fish, probably as a direct result of reduced cholesterol body pools. The proposed regulation of hepatic cholesterol and bile acid synthesis is shown in Fig. 5. In mammals, the committed step in hepatic cholesterol biosynthesis is the synthesis of mevalonate by Hmgcr, and hmgcr mRNA transcription is controlled by Srebp( Reference Brown and Goldstein 57 , Reference Goldstein, DeBose-Boyd and Brown 58 ). At low sterol levels, Srebp are cleaved and translocated to the nucleus and these up-regulate the expression of genes involved in sterol metabolism( Reference Goldstein, DeBose-Boyd and Brown 58 ). The involvement of Srebp2 in the up-regulation of cholesterol biosynthetic pathways has been shown in salmon fed plant products( Reference Kortner, Gu and Krogdahl 33 , Reference Leaver, Villeneuve and Obach 54 , Reference Brown and Goldstein 57 , Reference Morais, Taggart and Guy 59 ). The present study is in line with these previous studies, showing increased mRNA levels of srebp2, as well as hmgcr, in fish fed the PM diets.
In addition to Srebp, Ppar are also important regulators of lipid and sterol metabolism and may interact and crosstalk with Srebp, Lxr and Fxr( Reference Li and Chiang 60 ). In an earlier study also conducted in salmon, pparγ showed a stronger response to the diet supplemented with PM when compared with pparα ( Reference Kortner, Gu and Krogdahl 33 ). The present study is in line with this report, as the hepatic expression of pparα remained unaffected by PM inclusion, whereas that of pparγ was up-regulated. The observed induction of the expression of pparγ could be a result of alterations in hepatic fat homeostasis, as some studies have demonstrated an enhanced expression of pparγ in animal models of steatotic liver( Reference Gavrilova, Haluzik and Matsusue 61 – Reference Yu, Matsusue and Kashireddy 63 ). Recent mammalian studies have also shown that Pparγ regulates the expression of many genes involved in cholesterol transport and metabolism( Reference Baker, Malur and Barna 64 – Reference Chawla, Boisvert and Laffitte 67 ).
Effects of soya-saponin
In the present study, SA inclusion significantly decreased plasma cholesterol levels. A number of studies have shown that saponin from different sources lowers plasma cholesterol levels in various animals (reviewed in Francis et al. ( Reference Francis, Kerem and Makkar 21 )). These reports suggest that dietary saponins may be, at least partly, responsible for the hypocholesterolaemic effects of dietary PM in fish studies( Reference Kortner, Gu and Krogdahl 33 , Reference Yun, Mai and Zhang 68 ). Moreover, the present results showed a reduction in bile salt concentrations after SA exposure both in the intestinal lumen and in blood plasma. Many factors may possibly play a role in causing the decreased body pools of cholesterol and bile salt observed with dietary SA inclusion. For example, most saponins can form complexes with cholesterol and bile salts, thereby increasing their faecal excretion( Reference Oakenfull 69 , Reference Oakenfull and Sidhu 70 ). Additionally, SA has been shown to be an important factor responsible for the enteritis induced by dietary soyabean meal( Reference Knudsen, Uran and Arnous 22 , Reference Knudsen, Jutfelt and Sundh 23 , Reference Kortner, Skugor and Penn 71 , Reference Chikwati, Venold and Penn 72 ). The saponin-induced intestinal inflammation seems to impair enterocyte maturation and may, therefore, limit bile salt reabsorption from the gut. Furthermore, the results of the present study indicated that the capacity for hepatic biosynthesis and secretion of bile salts was down-regulated by saponin exposure, which may aggravate the depletion of bile salts.
The classical pathway of primary bile acid synthesis mediated by the rate-limiting enzyme Cyp7a1 is thought to be the major contributor to bile salt synthesis in humans( Reference Li and Chiang 60 ). The basal expression of cyp7a1 is primarily controlled by one or more specific transcription factors, wherein positive and negative regulation is mediated by Lxr and Fxr, respectively( Reference Gadaleta, van Mil and Oldenburg 73 ). In the present study, lxr mRNA levels were reduced after SA supplementation, but fxr levels remained unaffected. As an important transcription factor in sterol metabolism in mammals and, mostly probably, fish( Reference Archer, Lauter and Hauptmann 74 ), lxr transcripts have been cloned and characterised in salmonids( Reference Cruz-Garcia, Minghetti and Navarro 75 ). The hepatic expression of lxr has been shown to be higher in salmon fed fish oil than in those fed vegetable oil, which could be related to either dietary cholesterol/phytosterol contents or FA composition( Reference Cruz-Garcia, Minghetti and Navarro 75 ). The results of the present study showed that saponins could be another factor inducing the down-regulation of the expression of lxr in fish fed plant-derived alternatives. Consistent with the lxr expression profile, the expression of cyp7a1 was markedly down-regulated by SA exposure. These results suggest that the capacity for hepatic bile acid biosynthesis was down-regulated in fish fed SA.
In the liver, the ATP-binding cassette Abcg5 (which forms a heterodimer with Abcg8) is regulated by Lxr activation and mediates cholesterol transport from the liver into the bile( Reference Chawla, Repa and Evans 76 ). In the present study, the expression of both abcg5 and lxr was down-regulated by SA exposure. This result is in line with the previous observation that abcg5 and lxr displayed similar gene expression profiles in the liver of salmon fed soyabean meal( Reference Kortner, Gu and Krogdahl 33 ). The reason for the down-regulation of the expression of abcg5 is not clear; however, it is probably a result of alterations in hepatic sterol metabolism induced by SA. Similarly, the hepatic expression of the bile salt transporter abcb11 was down-regulated by SA. Since the bile salt concentration is about 100- to 1000-fold higher in the bile than in the hepatocytes, canalicular bile salt transport represents the rate-limiting step in bile formation( Reference Li and Chiang 60 ), and Abcb11 is mainly responsible for bile salt transport across the canalicular membrane( Reference Chawla, Repa and Evans 76 ). These results indicate that the capacity for bile salt transport from the liver to the biliary duct was decreased in fish fed SA.
In contrast to PM inclusion, SA inclusion did not affect lipid droplet accumulation or expression levels of genes involved in LP assembly. However, the expression of the FA transporters cd36, fatp and fabp2b was up-regulated in the pyloric caeca, indicative of an enhanced capacity for intestinal FA absorption. SA inclusion decreased plasma and digesta bile salt levels and hepatic capacity for bile salt synthesis and transport. Given the key role of bile salts in fat emulsification and micelle formation, it is likely that the decreased body pools of bile salts affected intestinal lipid absorption. Thus, the enhanced capacity for FA transport could be a response to the decreased body pool of bile salts in fish fed saponins.
Interaction
Studies on interactions between different ingredients would be useful to avoid ingredient combinations causing negative effects in fish when using PM. However, in the present study, the hypocholesterolaemic effects of SA in the PM group were not as obvious as those in the FM group. Hepatic gene expression profiles indicated that the capacity for cholesterol biosynthesis was up-regulated in fish by PM exposure. Hardly any information exists regarding the interactions between SA and ingredients in lupin meal, and the mechanism behind the weakened hypocholesterolaemic effects of SA supplementation in the PM diets is not clear. However, the present results indicated that SA supplementation alone could have hypocholesterolaemic effects on Atlantic salmon.
Conclusion
PM inclusion in salmon feed resulted in decreased growth performance, excessive lipid droplet accumulation in the pyloric caeca and liver and reduced plasma cholesterol levels. Gene expression profiling suggested that the capacity for LP assembly and cholesterol synthesis was up-regulated by PM inclusion, probably occurring as a result of impaired lipid transport and cholesterol metabolism. SA inclusion had hypocholesterolaemic effects on Atlantic salmon, accompanied by decreased bile salt levels. Gene expression profiles indicated that the capacity for hepatic biosynthesis and secretion of bile salts was down-regulated by saponin exposure.
Acknowledgements
The authors thank the technicians and supporting researchers at the Nofima Marine research station at Sunndalsøra, Norway, for their skilful animal care and scientific follow-up and Ellen K. Hage, Elin C. Valen and Gunn C. Østby in their laboratory in Oslo for excellent technical assistance. They also thank China Scholarship Council (CSC) for providing financial assistance to M. G. as a visiting PhD student at the Norwegian School of Veterinary Science (NVH).
The present study was financially supported by the Research Council of Norway's Centre of Excellence Aquaculture Protein Centre (APC; grant no. 145949/120). APC had no role in the design and analysis of the study or in the writing of this article.
The authors' contributions were as follows: M. G. performed the gene expression and histopathology work and wrote the manuscript; T. M. K. guided the gene expression work and revised the manuscript; M. P. and Å. K. designed and conducted the feeding trial and reviewed the manuscript. A. K. H. participated in the planning and conductance of the molecular work.
The authors declare that there are no conflicts of interest.