1. Introduction
Structural inheritance is a key factor in influencing and controlling the tectonic evolution and structural deformation pattern of orogenic belts during each stage of the Wilson cycle (Wilson, Reference Wilson1966; Dewey & Spall, Reference Dewey and Spall1975), where continents recurrently disintegrate and reassemble, and oceanic crust and lithosphere form, subduct and recycle. Although different contrasting models exist, several studies document the significant role of structural inheritance on the location of extension and continental break-up, rift and passive margin evolution and segmentation (e.g. Dunbar & Sawyer, Reference Dunbar and Sawyer1989; Piqué & Laville, Reference Piqué and Laville1996; Theunissen et al. Reference Theunissen, Klerkx, Melnikov and Mruma1996; Tommasi & Vauchez, Reference Tommasi and Vauchez2001; Petersen & Schiffer, Reference Petersen and Schiffer2016). Observation that rift propagation is not random but tends to follow the trend of pre-existing orogenic fabrics and plates, systematically reactivating ancient lithospheric structures, provides evidence for the active role played by inherited structures in rifting processes (e.g. Tommasi & Vauchez, Reference Tommasi and Vauchez2001). In convergent tectonic settings, oceanic extensional detachment faults (e.g. Maffione et al. Reference Maffione, Thieulot, van Hinsbergen, Morris, Plumper and Spakman2015), as well as fracture zones (e.g. Toth & Gurnis, Reference Toth and Gurnis1998; Hall et al. Reference Hall, Gurnis, Sdrolias, Lavier and Muller2003; Leng & Gurnis, Reference Leng and Gurnis2011), represent among others, important inherited structures which may provide ideal conditions to nucleate new subduction zones. Oceanic detachment faults, which are commonly associated with oceanic core complexes documented along the slow- (Atlantis Massif, Mid-Atlantic Ridge – MAR) and ultraslow-spreading (Atlantis Bank, Southern Indian Ridge – SWIR) ridges (e.g. Cannat, Reference Cannat1993; Tucholke, Lin & Kleinrock, Reference Tucholke, Lin and Kleinrock1998; Boschi, Früh-Green & Delacour, Reference Boschi, Früh-Green and Delacour2006; Karson et al. Reference Karson, Früh-Green, Kelley, Williams, Yoerger and Jakuba2006; Dick, Tivey & Tucholke, Reference Dick, Tivey and Tucholke2008; Miranda & Dilek, Reference Miranda and Dilek2010), pervasively cut the lithosphere to a depth of 7–8 km (e.g. Dick, Tivey & Tucholke, Reference Dick, Tivey and Tucholke2008; Escartin et al. Reference Escartin, Smith, Cann, Schouten, Langmuir and Escrig2008; Reston & Ranero, Reference Reston and Ranero2011). They represent weakness zones in the lithosphere, characterized by mylonitic to cataclastic shear zones up to hundreds of metres thick and dominated by serpentine minerals and other phyllosilicates (e.g. talc and chlorite; see Boschi, Früh-Green & Delacour, Reference Boschi, Früh-Green and Delacour2006; Boschi et al. Reference Boschi, Bonatti, Ligi, Brunelli, Cipriani, Dallai, D'Orazio, Früh-Green, Tonarini, Barnes and Bedini2013). These detachment faults are, in fact, ideal pathways for fluid infiltration in the lower crust and upper mantle, triggering hydrothermal alteration and serpentinization (see e.g. Schroeder, John & Frost, Reference Schroeder, John and Frost2002; Mével, Reference Mével2003; Boschi, Früh-Green & Delacour, Reference Boschi, Früh-Green and Delacour2006; Boschi et al. Reference Boschi, Bonatti, Ligi, Brunelli, Cipriani, Dallai, D'Orazio, Früh-Green, Tonarini, Barnes and Bedini2013).
In the Western Alps, the understanding of the role played by tectonostratigraphic inheritances, in conditioning and controlling their present-day architecture, is often difficult because of the multistage, intense deformation and metamorphic recrystallization they experienced during subduction and subsequent continental collision. As a result, the interpretation of the tectonic evolution of the Western Alps may be partially incomplete, misleading the role played by subduction- and collisional-related processes if that of inherited structures is not adequately taken into consideration and understood.
In this paper, we propose a new interpretation for the tectonostratigraphic architecture of the Monviso meta-ophiolite Complex (MO hereafter), which represents one of the best-preserved eclogite-facies ophiolite complexes in the Western Alps (Fig. 1). We present here the first geological map of the whole MO and, on the basis of systematic structural, stratigraphic and petrological observations, we re-interpret its former structural segmentation and partitioning into different Alpine tectonic units. Through the (i) restoration of the meta-ophiolite succession, which is characterized by a fossil mantle–sediment interface, and of preserved unconformities within the metasedimentary succession, and (ii) the detection of the main structures which define the present-day architecture of the MO, we extended our recent published results (Balestro, Fioraso & Lombardo, Reference Balestro, Fioraso and Lombardo2011; Balestro, Festa & Tartarotti, Reference Balestro, Festa, Dilek and Tartarotti2015; Festa et al. Reference Festa, Balestro, Dilek and Tartarotti2015) to the whole MO, documenting that its Alpine tectonic evolution was strongly influenced by its primary intra-oceanic tectonosedimentary physiography. The latter was inherited from the Late Jurassic oceanic core complex formed during extensional tectonics in a slow-spreading system related to the opening of the Ligurian–Piedmont ocean basin (Alpine Tethys). We provide new data to further discuss the role played by the occurrence of a Late Jurassic intra-oceanic ‘detachment fault’ (see ‘Baracun Shear Zone’ in Festa et al. Reference Festa, Balestro, Dilek and Tartarotti2015) in the Cenozoic Alpine evolution of the MO.

Figure 1. (a) Tectonic map of the Western Alps (modified from Balestro et al. Reference Balestro, Festa, Dilek and Tartarotti2015) with the location of the studied sector. (b) Location of map in (a).
Our data and observations from the MO indicate that apparently incomplete and highly deformed meta-ophiolite sequences that are juxtaposed across major shear zones in high-pressure belts around the world may not exclusively represent the product of subduction-related deformation. The distribution and orientation of seafloor spreading structural inheritances with respect to the orientation of the strain field related to subsequent subduction and collisional stages may, in fact, play a significant role in controlling the propagation of subsequent tectonic deformation, isolating and preserving remnants of the pre-orogenic tectonostratigraphic architecture of oceanic basins, and thus providing complementary data to better constrain palaeogeographic reconstructions.
2. Regional geological setting of the Western Alps and the Monviso Ophiolite
The Western Alps (Fig.1) resulted from the convergence of the Adria upper plate and the European lower plate with the interposed Ligurian–Piedmont oceanic basin (i.e. the Alpine Tethys; see e.g. Coward & Dietrich, Reference Coward, Dietrich, Coward, Dietrich and Park1989), which developed as a result of Middle to Late Jurassic rift-drift and seafloor spreading (e.g. Lemoine et al. Reference Lemoine, Bas, Arnaud-Vanneau, Arnaud, Dumont, Gidon, Graciansky, Rudkiewicz, Mégard-Galli and Tricart1986; Michard et al. Reference Michard, Goffe, Chopin and Henry1996; Vissers et al. Reference Vissers, Van Hinsbergen, Meijer and Piccardo2013). The present-day setting of the Western Alps consists of a double verging collisional belt, along which the collisional zone (i.e. the axial sector of the Alpine belt) involves an exhumed fossil subduction complex overthrusting the European foreland and the Adriatic retroforeland, to the W and E, respectively (e.g. Ricou & Siddans, Reference Ricou, Siddans, Coward and Ries1986; Bigi et al. Reference Bigi, Castellarin, Coli, Dal Piaz, Sartori, Scandone and Vai1990; Polino, Dal Piaz & Gosso, Reference Polino, Dal Piaz and Gosso1990). Remnants of the Ligurian–Piedmont oceanic basin correspond to different meta-ophiolite units (i.e. the Piedmont Zone; see e.g. Martin, Tartarotti & Dal Piaz, Reference Martin, Tartarotti and Dal Piaz1994; Dal Piaz, Bistacchi & Massironi, Reference Dal Piaz, Bistacchi and Massironi2003), which are tectonically sandwiched between the European and Adria continental margin units (Fig. 1a), and are classically distinguished into eclogite-facies (i.e. Zermatt–Saas Zone) and blueschist-facies units (i.e. Combin Zone; see Bearth, Reference Bearth1967).
The MO is one of the major and better investigated eclogitized remnants of the Ligurian–Piedmont oceanic lithosphere in the Western Alps (Lombardo et al. Reference Lombardo, Nervo, Compagnoni, Messiga, Kienast, Mevel, Fiora, Piccardo and Lanza1978; Lombardo, Rubatto & Castelli, Reference Lombardo, Rubatto and Castelli2002; Balestro, Fioraso & Lombardo, Reference Balestro, Fioraso and Lombardo2013 and references therein), and is tectonically juxtaposed against the eclogitized Dora Maira Massif, which was part of the European continental margin. A major ductile normal fault (i.e. the West Viso Detachment; Ballèvre, Lagabrielle & Merle, Reference Ballèvre, Lagabrielle and Merle1990; Tricart et al. Reference Tricart, Schwartz, Sue and Lardeaux2004) separates the MO from the overlying Queyras Schistes Lustrés Complex (Fig. 1a). The latter was metamorphosed under blueschist-facies conditions and consists of carbonate-rich metasedimentary rocks with meta-ophiolite bodies (Tricart & Lemoine, Reference Tricart and Lemoine1991; Tricart & Schwartz, Reference Tricart and Schwartz2006). The present-day architecture of the MO resulted from three main tectonic stages (named D1, D2 and D3) developed during (i) the Late Cretaceous to Middle Eocene subduction, (ii) the Late Eocene – Early Oligocene collision and W-verging accretion, and (iii) the Late Oligocene to Neogene westward tilting, which was driven by deep crust/mantle indentation and doming of the underlying Dora Maira Massif (Philippot, Reference Philippot1990; Rosenbaum & Lister, Reference Rosenbaum and Lister2005; Lardeaux et al. Reference Lardeaux, Schwartz, Tricart, Paul, Guillot, Béthoux and Masson2006; Balestro, Festa & Tartarotti, Reference Balestro, Festa, Dilek and Tartarotti2015). The D1 was coeval with the subduction-related eclogite-facies metamorphism, and is mainly recorded by an early foliation (i.e. the S1). The S1 overprinted ocean-related structures such as primary stratigraphic surfaces and magmatic foliation (i.e. the S0). The D2 was coeval with the early-exhumation-related blueschist- to greenschist-facies re-equilibration, and was characterized by W-verging non-cylindrical folding associated with shearing. D2 folds are tight to isoclinal and developed an axial plane foliation (i.e. the S2), corresponding to the gently to moderately W- to SSW-dipping regional foliation, which is parallel to the lithological contacts within the MO and to the basal tectonic contact with the underlying Dora Maira Massif. The D3 developed during late exhumation under lower-greenschist-facies conditions, and consists of open folds, which accommodated westward-extension along major shear zones (e.g. the contact between the MO and the Queyras Schistes Lustrés Complex). During the D3, as a consequence of doming of the Dora Maira Massif, the D1 and D2 structures were tilted.
The MO consists of serpentinite, metagabbros, metabasalt and metasediments (Compagnoni & Fiora, Reference Compagnoni and Fiora1976; Lombardo et al. Reference Lombardo, Nervo, Compagnoni, Messiga, Kienast, Mevel, Fiora, Piccardo and Lanza1978; MONVISO, 1980). The serpentinite hosts large bodies of Mg–Al metagabbro, with minor bodies of Fe–Ti-oxide metagabbro and very minor metaplagiogranite (Castelli, Rostagno & Lombardo, Reference Castelli, Rostagno and Lombardo2002; Castelli & Lombardo, Reference Castelli and Lombardo2007; Compagnoni, Rolfo & Castelli, Reference Compagnoni, Rolfo and Castelli2012), which gave Callovian–Oxfordian (163 ± 2 Ma; Rubatto & Hermann, Reference Rubatto and Hermann2003) and Kimmeridgian–Tithonian (152 ± 2 Ma, Lombardo, Rubatto & Castelli, Reference Lombardo, Rubatto and Castelli2002) crystallization ages, respectively. In the northern sector of the MO, Festa et al. (Reference Festa, Balestro, Dilek and Tartarotti2015) documented the remnants of an intra-oceanic detachment fault (i.e. the Baracun Shear Zone), which separates massive serpentinite and Middle–Upper Jurassic metagabbros in its footwall from metabasalt and metasediments in its hanging wall. Balestro, Festa & Tartarotti (Reference Balestro, Festa, Dilek and Tartarotti2015) highlighted that those metasediments derived from two different successions, consisting of Upper Jurassic calcschist, interfingered by ophiolite-derived detrital horizons (i.e. the syn-extensional succession), and Lower Cretaceous carbonate-rich calcschist devoid of any ophiolite-derived material (i.e. the post-extensional succession).
Following Lombardo et al. (Reference Lombardo, Nervo, Compagnoni, Messiga, Kienast, Mevel, Fiora, Piccardo and Lanza1978) and MONVISO (Reference Panayiotou1980), who subdivided the central part of the MO into six main tectonic units on the basis of the occurrence of tectonic contacts among different lithologies (Fig. 2a, b), different authors proposed new subdivisions (see Fig. 2c; e.g. Blake, Moore & Jayko, Reference Blake, Moore, Jayko, Coleman and Wang1995; Lombardo, Rubatto & Castelli, Reference Lombardo, Rubatto and Castelli2002; Angiboust et al. Reference Angiboust, Langdon, Agard, Waters and Chopin2012). The estimation of different P–T metamorphic peaks for the tectonic units (Fig. 2c; Blake, Moore & Jayko, Reference Blake, Moore, Jayko, Coleman and Wang1995; Messiga et al. Reference Messiga, Kienast, Rebay, Riccardi and Tribuzio1999; Schwartz et al. Reference Schwartz, Lardeaux, Guillot and Tricart2000; Groppo & Castelli, Reference Groppo and Castelli2010; Angiboust et al. Reference Angiboust, Langdon, Agard, Waters and Chopin2012) allowed the interpretation that different subduction metamorphic histories were documented within the MO. However, the structural ‘break’ separating these different subduction histories was controversially attributed to different tectonic contacts, located in different structural positions (see Fig. 2c).

Figure 2. (a) Simplified geological cross-section through the central part of the Monviso meta-ophiolite Complex, showing its early subdivision in different tectonic units according to Lombardo et al. (Reference Lombardo, Nervo, Compagnoni, Messiga, Kienast, Mevel, Fiora, Piccardo and Lanza1978) and Lombardo, Rubatto & Castelli (Reference Lombardo, Rubatto and Castelli2002). (b) Simplified geological map with traces of the geological cross-sections each referring to tectonostratigraphic interpretations and tectonometamorphic data summarized in (c). (c) Correlation between simplified tectonostratigraphic and tectonometamorphic columns of the MO, according to literature; P–T metamorphic peaks from Blake, Moore & Jayko (Reference Blake, Moore, Jayko, Coleman and Wang1995) (column 1: Lower Oceanic Unit, Serpentinite Mèlange; column 2: Forciolline Unit), Schwartz et al. (Reference Schwartz, Lardeaux, Guillot and Tricart2000) (column 2: Passo Gallarino and Viso Mozzo units), Messiga et al. (Reference Messiga, Kienast, Rebay, Riccardi and Tribuzio1999) (column 2: Lago Superiore Unit), Groppo & Castelli (Reference Groppo and Castelli2010) (column 2: Basal Serpentinite Unit) and Angiboust et al. (Reference Angiboust, Langdon, Agard, Waters and Chopin2012) (column 3: Monviso and Lago Superiore units).
The inner architecture of the MO is interpreted as either the product of subduction-related or collision-related tectonics. For example, Blake & Jayko (Reference Blake and Jayko1990) and Guillot et al. (Reference Guillot, Schwartz, Hattori, Auzende and Lardeaux2004) interpreted the MO as a fossilized serpentinite subduction channel, wherein eclogite blocks were tectonically incorporated forming a ‘serpentinite mélange’. Alternatively, Angiboust et al. (Reference Angiboust, Agard, Raimbourgh, Yamato and Huet2011) suggested that the MO corresponds to a primary, almost continuous, fragment of upper oceanic lithosphere subducted at ~ 80 km depth and cross-cut by different eclogite-facies shear zones. Finally, Schwartz et al. (Reference Schwartz, Lardeaux, Guillot and Tricart2000) proposed that the tectonic juxtaposition of the MO units occurred during collision-related exhumation, under blueschist-facies metamorphic conditions.
3. Tectonostratigraphy and structural architecture
Our geological mapping of the whole MO (1:10 000 scale), and detailed structural and lithostratigraphic observations, allow us to re-interpret the tectonostratigraphy of the whole MO (Fig. 3).

Figure 3. Geological map (a) and representative geological cross-sections (b) of the Monviso meta-ophiolite Complex (note that the scale is different from the map).
3.a. The meta-ophiolite sequence
Based on the above-mentioned literature data and original observations, the different lithostratigraphic units of the MO (Fig. 3a) are described in the following sections, as pertaining to mantle-derived rocks and syn- to post-extensional sequences (see Balestro, Festa & Tartarotti, Reference Balestro, Festa, Dilek and Tartarotti2015 and Festa et al. Reference Festa, Balestro, Dilek and Tartarotti2015), where extension is related to the Late Jurassic rifting and seafloor oceanic spreading of the Ligurian–Piedmont oceanic basin.
3.a.1. Mantle rocks and metagabbro sequence
This sequence consists of massive serpentinite and metagabbros. The serpentinite (Fig. 4a) is made of antigorite and magnetite and derives from lherzolite and minor harzburgite. The primary mineral assemblage is preserved as relics of clinopyroxene and orthopyroxene porphyroclasts, and as textural relics of spinel and olivine. Rare relics of bastite and lizardite mesh textures highlight that serpentinization of the peridotite began during oceanization. The uppermost part of the serpentinite is locally characterized by the occurrence of both massive and sheared meta-ophicarbonate (Fig. 4b), which well documents the original exhumation of mantle rocks at the seafloor. The meta-ophicarbonate horizons range from a few metres to a few tens of metres in thickness (e.g. S of Colle di Luca), and consist of irregular calcite and dolomite vein networks which lace around centimetre to decimetre ‘clasts’ of serpentinite. The massive serpentinite and the meta-ophicarbonate horizons pass upwards into the mylonitic serpentinite and the talc-chlorite schist of the Baracun Shear Zone (BSZ hereafter; see below).

Figure 4. Field images of main lithological units, contact relationships and structures in the mantle rocks and metagabbro, syn-extensional and post-extensional sequences. (a) Rodingitized metagabbro dyke in massive serpentinite (E of Viso Mozzo). (b) Close-up view of a meta-ophicarbonate horizon, consisting of serpentinite clasts (s) meshed in a calcite-dolomite vein network (c) (S of Colle di Luca). (c) Mg–Al metagabbro deformed by recumbent D2 folds with stretched and boudinaged long limbs (E of Viso Mozzo). (d) Close-up view of a mylonitic Fe–Ti-oxide metagabbro (NE of Casteldelfino). (e) Preserved magmatic relationship between Fe–Ti-oxide metagabbro and metaplagiogranite (NW of Sampeyre). (f) Metabasalt with well-preserved pillow structure (Colle delle Lobbie). (g) Close-up view of a contact between Mg–Al metagabbro and metabasaltic dyke (E of Viso Mozzo). (h) Metabasalt showing relict brecciated texture and penetrative D3 fracture cleavage (dashed lines) (NW of M. Granero). (i) Overturned stratigraphic contact between massive serpentinite and metasandstone of gabbroic composition (NW of M. Granero). (j) Close-up view of a layer of matrix-supported mafic metabreccia characterized by sub-angular clasts of gabbroic composition (Colle del Baracun). (k) Overturned primary contact between the metabasalt of the syn-extensional sequence and the marble of the post-extensional one (SE of Colle di Luca). (l) D2 non-cylindrical fold hinge in a block of post-extensional calcschist (Colle del Baracun). (m) D2 tight folds highlighted by alternating layers of calcschist, marble and quartz-rich schist (Colle del Baracun). Hammer for scale is 30 cm long.
The metagabbros, which were intruded into the original peridotite, range in size from decimetre dykes (Fig. 4a) and pods to hundreds of metres thick and kilometres long bodies. The latter, although also occurring in the northern (i.e. Colle del Baracun area) and southern (i.e. M. Nebin area) sectors of the MO, are more abundant and continuous between Colle Armoine and NE of Casteldelfino (i.e. the central part of the MO; Fig. 3a). In this sector, depending on both the primary tectonostratigraphy and subsequent strain partitioning during Alpine deformation, the metagabbro bodies show different characteristics. Metagabbro occurring ENE of Colle Armoine and between Monviso and P. Caprera, partly preserves its primary intrusive shape and almost shows coarse-grained magmatic fabrics, whereas between Pian del Re and Colle di Luca, metagabbro bodies are elongated in shape and their primary fabric is widely overprinted by Alpine deformation and recrystallization. In particular, strongly elongated bodies of mylonitic metagabbro (Fig. 4d) occur between N of Cima delle Lobbie and NE of Casteldefino.
Metagabbros consist of both Mg–Al-rich and Fe–Ti-rich compositions, ranging in texture from coarse-grained pegmatitic to prevalent medium-grained foliated (Fig. 4c). The former (Fig. 4c) is prevalent, showing a mineral assemblage characterized by (i) relics of a magmatic pyroxene almost replaced by Cr-omphacite, (ii) rare relics of magmatic chromite and ilmenite, and (iii) textural relics of the original plagioclase replaced by aggregates of clinozoisite and albite. The olivine-metagabbro, occurring between Monviso and P. Caprera, shows a magmatic fabric with pyroxene of chromian-diopside composition and is characterized by levels of cumulate metatroctolite. The Fe–Ti-oxide metagabbro (Fig. 4d) almost occurs within the Mg–Al metagabbro as differentiated pods. It displays a fine-grained banded texture and shows relics of a magmatic pyroxene of augite composition, which are retained in a mineral assemblage mainly consisting of garnet, omphacite and rutile (for details see Balestro et al. Reference Balestro, Lombardo, Vaggelli, Borghi, Festa and Gattiglio2014).
The metagabbro dykes are almost transformed into rodingite (Fig. 4a), mainly consisting of Mg-chlorite, diopside, vesuvianite and garnet of grossular composition. Some rodingite dykes (e.g. E of Casteldelfino and close to Colle di Sampeyre) are poorly deformed and lack the pervasive metamorphic overprint (Bogatto & Castelli, Reference Bogatto and Castelli1997), highlighting that metasomatism is linked to early oceanic serpentinization.
A tens of metres thick body of medium-grained metaplagiogranite (152 ± 2 Ma; Lombardo, Rubatto & Castelli, Reference Lombardo, Rubatto and Castelli2002), which occurs NW of Sampeyre, shows well-preserved intrusive relationships with Fe–Ti-oxide metagabbro (Fig. 4e). It consists of albite, quartz and jadeite, and is cross-cut by albite–quartz-bearing leucocratic dykes (Castelli, Rostagno & Lombardo, Reference Castelli, Rostagno and Lombardo2002).
3.a.2. Syn-extensional sequence
This sequence consists of metabasalt (Fig. 4f, g, h) and metasediments. The former, ranging in thickness from a few metres to several hundreds of metres, corresponds to (i) massive aphyric metabasalt, widespread between Monviso and Cima delle Lobbie, (ii) tholeiitic meta-pillow lavas (Fig. 4f) and (iii) brecciated metabasalt (Fig. 4h), grading into volcaniclastic-derived layers. The latter are characterized by a medium-grained banded texture, which is defined by alternating light-green–yellow layers, mainly consisting of albite, epidote and clinozoisite, and dark-green layers of prevalent Na–Ca amphibole. Fine-grained dykes of basaltic composition (Fig. 4g), with well-preserved magmatic relationships, occur within metagabbro N and E of Viso Mozzo, and between Monviso and P. Caprera.
The syn-extensional metasediments (see also Lagabrielle, Reference Lagabrielle1994) consist of calcschist interbedded with decimetre to sub-decimetre thick horizons of mafic metasandstone and metabreccia (Fig. 4i, j). The calcschist is made of calcite, quartz and white mica, and includes aggregates of white mica, zoisite/clinozoisite and graphite, pseudomorphic after lawsonite, and clast-derived mafic to ultramafic aggregates of Mg-chlorite and Cr-rich white mica (for details see Balestro et al. Reference Balestro, Lombardo, Vaggelli, Borghi, Festa and Gattiglio2014). The interbeds of mafic metasandstone and metabreccia are of gabbroic composition and commonly show a graded texture. The metabreccia laterally grades, at the tens of metres scale, into metasandstone of the same composition. Clasts of metabreccia are sub-angular in shape and up to a few centimetres in size (Fig. 4j); they consist of aggregates of omphacite, chlorite, Cr-rich white mica and epidote. In addition to these minerals, the matrix is characterized by the occurrence of quartz and white mica. The thickness of the syn-extensional metasedimentary unit shows significant variations towards both E–W and N–S directions. In the Colle del Baracun, N of the Colle Armonie and E of the M. Nebin sectors (Fig. 3), it shows an increase in thickness from 0 to about 70 m towards the W and away from the underlying talc-chlorite schist of the BSZ (see below).
Although in a few sectors (e.g. NW of M. Granero) metasediments, up to a few metres thick, are stratigraphically interposed between serpentinite and metabasalt, the direct relationships between the thickest syn-extensional metasediment and metabasalt successions are difficult to observe. However, geological mapping suggests a lateral heteropic contact.
3.a.3. Post-extensional sequence
This sequence is extensively exposed in the southern sector of the MO, while in other sectors it defines narrow horizons, up to a few tens of metres thick. It consists of calcschist devoid of any ophiolite-derived detrital material, and characterized by the occurrence of levels of marble and/or quartz-rich schist, centimetres to decimetres in thickness (Fig. 4k, l, m). The age of this succession, based on a correlation with the similar unmetamorphosed sediments of the Northern Apennines, was referred to the Early Cretaceous (Lagabrielle, Reference Lagabrielle1994). The calcschist mainly consists of calcite, ankerite, quartz, white mica and epidote, whereas the quartz-rich schist is made of quartz, white mica and chlorite, with minor albite and garnet. In the Colle del Baracun area, the post-extensional metasediments unconformably overlie both the mantle-derived rocks, syn-extensional sequence and rocks of the BSZ (see below). The characteristics of its basal contact, which lacks any Alpine mylonitic structure and superposes the post-extensional metasediments onto different lithostratigraphic units, allowed it to be interpreted as a primary unconformity surface (Fig. 4k). The occurrence of quartz-rich schist levels documents a significant terrigenous input into the basin, showing that the post-extensional sequence consists of distal carbonate and mixed siliciclastic–carbonate turbidites sourced from a continental margin area.
3.b. Architecture of the Monviso meta-ophiolite complex
The present-day architecture of the MO (Fig. 3b) mainly results from D2 non-cylindrical folding and from shearing along major shear zones of different ages: (i) the ocean-related BSZ (sensu Festa et al. Reference Festa, Balestro, Dilek and Tartarotti2015), (ii) the D2-related Granero–Casteldelfino Shear Zone, here defined for the first time, and (iii) the D3-related Villanova–Armoine Shear Zone (Fig. 3a).
D2 folds are W-verging and, because of the subsequent D3-related tilting, are characterized by a recumbent geometry (i.e. synformal anticlines and antiformal synclines). They correspond to kilometer macroscale structures which pervasively folded the above-described meta-ophiolite sequence, and are linked with second-order folds occurring at different scales. The core of the synformal anticlines consists of serpentinite, whereas the core of the antiformal synclines consists of metabasalt and metasediments. The best map evidences of D2 folding are the occurrence, in different sectors of the MO (Fig. 3a), of both syn-extensional and post-extensional metasediments at the top and the bottom (i.e. the overturned ones) of the massive serpentinite (e.g. the M. Nebin area), or the occurrence of serpentinite above the metabasalt (e.g. N and S of Colle di Vallanta). Tight to isoclinal D2 folds (Fig. 4m) deform the previous S1-parallel fabric and developed an axial plane foliation (S2). D2 folding is characterized by a strong component of shearing, which is highlighted at the mesoscale by sheath folds (Fig. 4l), and by the occurrence of rootless fold hinges and stretched and boudinaged long limbs (Fig. 4c).
3.b.1. The Baracun Shear Zone (BSZ)
The BSZ (Fig. 3a) extends along the whole MO and, because of pervasive D2 folding (Fig. 5a), it occurs at different structural levels, including the highest one (i.e. N of Colle di Vallanta; Fig. 5b). It separates mantle rocks and metagabbros in its footwall from the syn-extensional sequence (metabasalt and calcschist with mafic intercalations) in its hanging wall. The Lower Cretaceous post-extensional carbonate-rich calcschist unconformably overlies the BSZ (Fig. 5a) and both the footwall and hanging wall successions, constraining the age of this shear zone to the pre-Early Cretaceous (see Balestro et al. Reference Balestro, Festa, Dilek and Tartarotti2015). The BSZ consists of metres to tens of metres thick talc-chlorite schist and mylonitic serpentinite, embedding blocks of metagabbro (Fig. 5a), decimetres to several metres wide, tectonically wrenched from the footwall mantle-rocks assemblage. This scale-independent, block-in-matrix fabric (sensu Dilek et al. Reference Dilek, Festa, Ogawa and Pini2012; Festa et al. Reference Festa, Dilek, Pini, Codegone and Ogata2012 and references therein) is significantly devoid of any material derived from the syn- and post-extensional sequences. The talc-chlorite schist matrix (Fig. 5c) mainly consists of talc, Mg-chlorite and amphibole of tremolite-actinolite composition, and, among minor minerals, calcite, magnetite, garnet and apatite occur. The associated mylonitic serpentinite mainly consists of antigorite and magnetite, with minor talc, calcite and crysotile. Blocks of metagabbro are mainly of an Fe–Ti-richer composition and consist of omphacite-garnet-and-rutile mineral assemblages, with relics of a magmatic pyroxene of augite composition. The latter locally retains fine inclusions of talc, quartz and, remarkably, Mg-hastingsitic hornblende. In contrast to those occurring in the massive serpentinite, these metagabbros do not show rodingitic metasomatic reactions with the embedding talc-chlorite schist and serpentinite matrix. Blocks of metagabbro were elongated during D1-related stretching deformation and subsequently deformed by D2 folds. The external part of some blocks consists of decimetres thick layers of clast-supported metabreccia of tectonic origin (see Balestro, Festa & Tartarotti, Reference Balestro, Festa, Dilek and Tartarotti2015) in which the clasts have the same gabbroic compositions as the blocks. Observation that the metabreccia is overprinted by S1 foliation and D2 folds at both the micro- and mesoscale constrains brecciation processes to before the D1 phase (for details see Festa et al. Reference Festa, Balestro, Dilek and Tartarotti2015). At the microscale, the talc-chlorite schist matrix shows pluri-millimetre thick chlorite-rich and talc-rich domains, which correspond to rootless hinges of D2 folds. Although the BSZ is locally overprinted and reactivated (see below) by Alpine-related deformation, these data overall constrain the BSZ development to before the D1 subduction-related deformation and metamorphic overprint.

Figure 5. (a) Panoramic view of the folded Baracun Shear Zone at Colle del Baracun: the meta-ophiolite sequence is here deformed by D2 folds and consists of massive serpentinite, talc-chlorite schist embedding blocks (B) of metagabbro (the BSZ), syn-extensional metasediments and post-extensional ones. Note that the post-extensional metasediments rest unconformably (dotted line) on both the syn-extensional ones and the rocks of the BSZ (modified after Balestro et al. Reference Balestro, Festa, Dilek and Tartarotti2015). (b) Massive serpentinite and talc-chlorite schist of the BSZ occurring at the top of the MO, along the tectonic contact with the overlying Queyras Schistes Lustrés Complex (W of M. Granero). (c) Close-up view of talc-chlorite-schist of the BSZ, wherein the S2-parallel fabric (black lines) is cross-cut by D3 extensional shear planes (dashed lines) (Colle del Baracun). (d) Panoramic view of the Granero–Casteldelfino Shear Zone: the GCSZ (dashed lines) consists of post-extensional mylonitic calcschist and serpentine schist, and it juxtaposes metabasalt (in the hanging wall) on metabasalt and post-extensional calcschist (in the footwall), deformed by D2 folds (SSW of M. Granero). (e) Close-up view of the serpentine schist of (d), showing the structural relationships between the mylonitic foliation (dashed lines) and the S2 (black lines). (f) Close-up view of the post-extensional mylonitic calcschist of (d). (g) View of the Villanova–Armoine Shear Zone: the VASZ (dashed lines) consists of serpentine schist and juxtaposes massive serpentinite (in the hanging wall) on metabasalt (in the footwall) (NW of Villanova). Hammer for scale is 30 cm long.
3.b.2. The Granero–Casteldelfino Shear Zone (GCSZ)
The GCSZ (Fig. 3a) is roughly 15 km long and up to tens of metres thick, and controlled the architecture of the MO. This low-angle W- to SW-dipping (i.e. roughly parallel to the S2 foliation) shear zone (Fig. 5d) superposes two overturned successions which consist of metabasalt and post-extensional metasediments in the hanging wall, and either serpentinite or metagabbro, a BSZ-rocks assemblage, metabasalt and both syn- and post-extensional metasediments, in the footwall. The localization of the GCSZ, along rootless hinges and highly stretched long limbs of second-order D2 folds (Fig. 5d), highlights that it accommodated a component of shearing related to W-verging D2 deformation. The displacement along the GCSZ gradually decreases down to 0 m towards both the N (i.e. in the M. Granero area) and S (i.e. NE of Casteldelfino), where the shear zone ends within the metabasalt. Remarkably, the evidence that the lateral terminations of the GCSZ roughly correspond to the lateral terminations of the narrow horizons of the post-extensional metasediments, which mark at the base its hanging wall, suggests that shearing was controlled by rheological contrast between those metasediments and the footwall rocks.
At the mesoscale, the GCSZ is defined by centimetres thick shear zones displaying a mylonitic foliation, which is roughly parallel to the S2. These shear zones particularly developed along talc-chlorite schist, mylonitic serpentinite (Fig. 5e) and post-extensional calcschist with levels of marble and quartz-rich schist (Fig. 5f). In these metasediments, the mylonitic fabric almost overprinted the primary compositional banding. Also the metagabbro occurring in the footwall of the GCSZ (e.g. the metagabbro between N of Cima delle Lobbie and NE of Casteldefino) displays a mylonitic fabric (Fig. 4d). Where the mylonitic foliation and the S2 are not parallelized, the shear zone shows an orientation at a low angle to the S2. The mylonitic foliation generally shows shallow dips to the SW and stretching lineations gently plunging to the SSW. The shear zone usually exhibits a sinistral sense of shear and structural associations (i.e. S-C structures) consistent with top-to-SW kinematics (i.e. consistent with the W-verging D2 folds).
3.b.3. The Villanova–Armoine Shear Zone (VASZ)
The VASZ (Fig. 3a) corresponds to a few metres thick, W-dipping, extensional shear zone, which displaces by about a few hundred metres both the whole meta-ophiolite sequence and the D2-related structural architecture of the northern sector of the MO. It represents the most persistent D3 structure occurring in the MO and it is well defined by the gradual steepening of the S2 foliation and of the S2-parallel lithological contacts. The VASZ extends for ~ 10 km between the Villanova area and Colle Armoine, and, in different sectors, it juxtaposes metabasalt, rocks of the BSZ and narrow massive serpentinite, in the hanging wall, to metabasalt and syn- and post-extensional metasediments, in the footwall (Fig. 5g). It is structurally associated with the D3 tectonic contact which separates the MO and the overlying Queyras Schistes Lustrés Complex. At the mesoscale, D3 deformation consists of widespread fracture cleavages (Fig. 4h) and extensional shear planes (Fig. 5c), millimetres to centimetres thick and centimetres to metres spaced, which are often associated with W-verging open folds (i.e. the D3 folding). These structures mainly developed within post-extensional metasediments, which are locally severely reworked by shearing-related deformation. Where they are absent (i.e. N of Colle del Baracun), shearing is localized along rocks of the BSZ (Fig. 5c). Both shear planes and related slickenside lineations dip moderately to the W. Normal sense of shear is defined by S-C structures.
4. Discussion
Our description of the tectonostratigraphic architecture of the MO allows (i) discrimination between inherited intra-oceanic structures and Alpine ones, and (ii) understanding the role of those inherited structures in controlling the Alpine tectonic evolution of the MO. The addition of these new observations to our published data (Balestro, Festa & Tartarotti, Reference Balestro, Festa, Dilek and Tartarotti2015; Festa et al. Reference Festa, Balestro, Dilek and Tartarotti2015) allows us to describe in detail the complete tectonic evolution of the MO from the Late Jurassic seafloor spreading, to subduction, continental collision and exhumation stages.
4.a. Tectonic evolution of the MO and role of inherited structures
An intra-oceanic tectonic stage and three Alpine-related ones can be differentiated in the evolution of the MO (Fig. 6).
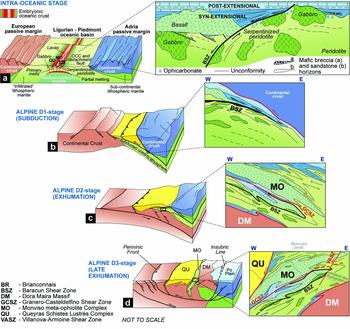
Figure 6. Cartoons showing the different stages of the tectonic and geodynamic evolution of the Monviso meta-ophiolite Complex from the Jurassic intra-oceanic stage (a) to the Alpine subduction (b), early exhumation (c) and late exhumation (d) stages (modified from Lardeaux et al. Reference Lardeaux, Schwartz, Tricart, Paul, Guillot, Béthoux and Masson2006; Balestro et al. Reference Balestro, Festa, Dilek and Tartarotti2015; Balestro, Festa & Tartarotti, Reference Balestro, Festa, Dilek and Tartarotti2015). See text for explanation. Details of each tectonic and geodynamic stage are represented, showing the role of the inherited intra-oceanic tectonostratigraphic architecture in controlling the subsequent Alpine deformation of the Monviso meta-ophiolite Complex. Detail of (a) is modified from Balestro et al. (Reference Balestro, Festa, Dilek and Tartarotti2015) and Festa et al. (Reference Festa, Balestro, Dilek and Tartarotti2015).
4.a.1. Seafloor spreading stage and oceanic core complex development
The early seafloor spreading stage coincided with the production of magma by decompressional melting of the asthenosphere during its slow upwelling. This process led to the intrusion of gabbroic dykes and plutons in an upper mantle, consisting of lherzolite with minor harzburgite. Intrusions mainly consisted of Mg–Al gabbro with differentiated pods of Fe–Ti-richer composition, which crystallized in late Middle Jurassic time (i.e. 163 ± 2 Ma; Rubatto & Hermann, Reference Rubatto and Hermann2003), and minor Late Jurassic plagiogranite (152 ± 2 Ma; Lombardo, Rubatto & Castelli, Reference Lombardo, Rubatto and Castelli2002). Exhumation at the seafloor of both gabbro and serpentinized peridotite is highlighted by the occurrence of (i) meta-ophicarbonate at the top of the massive serpentinite, (ii) stratigraphic contacts between syn-extensional/post-extensional metasediments and massive serpentinite, and (iii) intercalations of gabbroic detrital material within the syn-extensional metasediments. This exhumation was driven by the BSZ (Fig. 6a), whose composition and tectonostratigraphic relationships document that it corresponds to an intra-oceanic detachment fault and cannot be interpreted as a subduction-related ‘serpentinite mélange’ as suggested by some authors (Blake & Jayko, Reference Blake and Jayko1990).
The BSZ controlled seafloor spreading and extensional tectonics by simple shear (Festa et al. Reference Festa, Balestro, Dilek and Tartarotti2015); this produced a Late Jurassic oceanic core complex (OCC; see Fig. 6a). The development of the BSZ was promoted by early intrusion of gabbroic bodies and by later serpentinization-related rheological weakening, which was coeval with intrusion of differentiated gabbroic bodies. Continued faulting and a related temperature decrease as the footwall was exhumed, developed the BSZ as a narrow deformation band. When faulting reached a relatively shallow depth (1–3 km), percolation of seawater down the fault may have induced the hydration of mantle rocks along the shear zone, producing serpentinite and hydrothermal metasomatism, which formed talc-chlorite schist (Balestro, Festa & Tartarotti, Reference Balestro, Festa, Dilek and Tartarotti2015). The latter is well comparable with those observed in hydrothermally metasomatized oceanic rocks along detachment fault zones in the Mid-Atlantic Ridge (Mével, Reference Mével2003; Boschi, Früh-Green & Delacour, Reference Boschi, Früh-Green and Delacour2006), where these rocks are documented to represent weak and highly mobile horizons (e.g. Moore & Lockner, Reference Moore and Lockner2007), able to migrate for long distances along faults and lubricating them (Byerlee & Brace, Reference Byerlee and Brace1968; Dengo & Logan, Reference Dengo and Logan1981; Reference HirauchiHirauchi, 2006a ,b; Dick, Tivey & Tucholke, Reference Dick, Tivey and Tucholke2008). In present-day oceanic core complexes, the highly deformed peridotite and talc-chlorite schist associated with detachment faults commonly entrains high-temperature mylonite blocks, produced during the earliest stage of fault nucleation and localized igneous intrusion. Similarly, the BSZ embeds blocks of differentiated metagabbro which are mainly of Fe–Ti-richer composition and locally retain relics of hornblende, which testify to the occurrence of ocean-related medium-T metamorphism, as also documented by Rubatto & Angiboust (Reference Rubatto and Angiboust2015). In contrast to those occurring in the massive footwall serpentinite, these metagabbro blocks do not show rodingitic reactions with the hosting rocks (i.e. the talc-chlorite and serpentinite schists), highlighting that the original peridotite was already serpentinized at the time of intrusion. The occurrence of tectonic metabreccia in the external part of some metagabbro blocks of the BSZ indicates that this gabbro was exposed along the BSZ, cross-cut and fragmented by brittle faults at shallow structural levels (1–2 km depth). The time constraints for the seafloor spreading stage and oceanic core complex development are provided by the unconformable deposition of Lower Cretaceous post-extensional calcschist (Festa et al. Reference Festa, Balestro, Dilek and Tartarotti2015; Tartarotti et al. Reference Tartarotti, Festa, Benciolini and Balestro2017; see Fig. 6a). Therefore, the extensional stage took place between middle Callovian – early Oxfordian (163 ± 2 Ma) and middle Kimmeridgian – early Tithonian (152 ± 2 Ma) times.
4.a.2. Alpine-related tectonic stages
The widespread preserved oceanic tectonostratigraphy highlights that, during convergence-related tectonics (i.e. the D1 stage), the MO was subducted and detached from the main slab as a coherent slice of oceanic lithosphere, a few kilometres thick (Fig. 6b). The whole meta-ophiolite sequence was metamorphosed and sheared under eclogite-facies conditions but not tectonically dismembered into different units or eclogitic blocks in a serpentinite matrix, forming a mélange (i.e. the serpentinite channel of Guillot et al. Reference Guillot, Schwartz, Hattori, Auzende and Lardeaux2004). The absence of metasediments younger than Early Cretaceous in age suggests that they may have been tectonically delaminated and/or detached at shallow structural levels during the earlier stage of subduction (i.e. Late Cretaceous), without being buried at deeper structural levels along the subduction thrust interface. If compared with the Ligurian Units in the Northern Apennines, which correspond to the non-metamorphosed succession deposited in the Ligurian–Piedmont oceanic basin (e.g. Marroni, Meneghini & Pandolfi, Reference Marroni, Meneghini and Pandolfi2010; Festa et al. Reference Festa, Dilek, Codegone, Cavagna and Pini2013 and references therein), it is possible to suggest that also in the MO the stratigraphic base of the Upper Cretaceous successions may have acted as a regional-scale detachment level, separating units which were differently (or only in part) involved in the subduction processes.
The occurrence of remnants of an intra-oceanic detachment fault (i.e. the BSZ) and of the related syn-extensional sequence, which are sealed by the Lower Cretaceous post-extensional sequence, highlights that the BSZ was not significantly reactivated during D1 tectonics (Fig. 6b), suggesting that possibly it was not favourably oriented with respect to the orientation of the strain field during subduction. Although primary stratigraphic surfaces (e.g. the S0 sedimentary bedding) were overprinted by the early S1 foliation, the tectonostratigraphic relationships between the primary lithostratigraphic units were mostly preserved (Fig. 6b), and the development of the S1 was almost only associated with stretching and boudinage of the meta-ophiolite sequence. The best-preserved D1 structures occur at the meso- and microscale. D1 stretching is defined by elongated blocks of metagabbro within the BSZ (Balestro, Festa & Tartarotti, Reference Balestro, Festa, Dilek and Tartarotti2015), whereas boudinage is well highlighted by pinch-and-swell structures and eclogite-facies veining within layered metagabbro (Philippot & Kienast, Reference Philippot and Kienast1989). These veins consist of tension gashes and shear fractures filled by omphacite, garnet and rutile, and formed by locally sourced fluids at the peak of the prograde metamorphic path of the MO (Nadeau, Philippot & Pineau, Reference Nadeau, Philippot and Pineau1993; Spandler, Pettke & Rubatto, Reference Spandler, Pettke and Rubatto2011).
The subsequent D2 deformation records the continental collisional stage (Fig. 6c), which developed during early exhumation-related metamorphic re-equilibration in the epidote-blueschist to greenschist-facies transition (Schwartz et al. Reference Schwartz, Lardeaux, Guillot and Tricart2000; Balestro et al. Reference Balestro, Lombardo, Vaggelli, Borghi, Festa and Gattiglio2014). This deformational stage played a primary role in forming the present-day structural architecture of the MO (Fig. 6c). D2 folds occur at all scales and developed a pervasive axial plane foliation (i.e. the S2), which is parallel to the lithological contacts. At the macroscale, W-verging non-cylindrical D2 folds deformed the inherited tectonostratigraphic setting, forming structures which correspond to present-day synformal anticlines and antiformal synclines (Fig. 6c). Remarkably, the inherited tectonostratigraphic setting did not corresponded to a flat and regular multilayer of mantle rocks, gabbros and overlying sedimentary covers but was characterized by a heterogeneous tectonostratigraphic sequence with lateral and vertical variations of both facies and thickness controlled by intra-oceanic faults (i.e. the BSZ), which strongly controlled D2 folding (Fig. 6c).
Owing to strain partitioning, as well as the differential strength and viscosity of the rocks, some of the metagabbro and massive metabasalt (e.g. the metabasalt occurring in the core of the antiformal syncline) were preserved from the pervasive D2 deformation, while metasediments and schists of the BSZ were severely deformed. In particular, the BSZ represented a weak horizon along which the highest degree of D2-related shearing and folding was concentrated, producing a mylonitic fabric in the talc-chlorite and serpentine schists (Fig. 6c). The occurrence of these mylonites at different structural levels was recognized as Alpine-related tectonic contacts (Lombardo et al. Reference Lombardo, Nervo, Compagnoni, Messiga, Kienast, Mevel, Fiora, Piccardo and Lanza1978) and led to the distinction of different tectonometamorphic units in the central sector of the MO (Schwartz et al. Reference Schwartz, Lardeaux, Guillot and Tricart2000; Guillot et al. Reference Guillot, Schwartz, Hattori, Auzende and Lardeaux2004). Nevertheless, P–T peak estimations across the BSZ are very similar (compare, e.g. Groppo & Castelli, Reference Groppo and Castelli2010, Angiboust et al. Reference Angiboust, Langdon, Agard, Waters and Chopin2012 and Balestro et al. Reference Balestro, Lombardo, Vaggelli, Borghi, Festa and Gattiglio2014) and meaningful metamorphic gaps do not occur. Detection of tectonic structures and their relationships with the primary lithostratigraphic units at the scale of the whole MO highlights that the BSZ occurs at different structural levels only because of D2 folding and did not acquire a significant displacement during the subduction and collisional stages.
D2 non-cylindrical folding accommodated a significant component of tectonic shearing. At the macroscale, the most important D2 shearing structure corresponds to the GCSZ, along which the meta-ophiolite sequence was detached (Fig. 6c), and the hanging wall unit (i.e. metabasalt and post-extensional metasediments) was juxtaposed against the footwall one (i.e. serpentinite, BSZ-rocks and metabasalt). Mesoscale structural observations suggest that the detachment enucleated along hinges and long limbs of second-order D2 folds. Within the folded meta-ophiolite sequence, two lithological units, corresponding to the post-extensional metasediments and the schists of the BSZ, acted as preferred sites for concentration and propagation of deformation (Fig. 6c). The post-extensional carbonate-rich calcschist in the hanging wall was severely stretched and now consists of a narrow zone, suggesting that the rheology of those metasediments controlled the localization and propagation of shearing associated with D2 non-cylindrical sheath folding. Notably, the gradual decrease in displacement down to 0 m of the GCSZ towards both the N and S (Fig. 3a) contrasts with its supposed role in juxtaposing two different tectonometamorphic units (i.e. the Upper Shear Zone of Angiboust et al. Reference Angiboust, Langdon, Agard, Waters and Chopin2012). The possible interpretation of the GCSZ as a scissor fault accommodating local tearing within the subducted mantle is also to be excluded because of the stratigraphical constraints, which show that roughly the same lithostratigraphic units are juxtaposed along both its hanging wall and footwall. Our new constraints about the GCSZ highlight that it should be interpreted as a D2-related tectonic contact, as also suggested by Schwartz et al. (Reference Schwartz, Lardeaux, Guillot and Tricart2000) and Balestro, Fioraso & Lombardo (Reference Balestro, Fioraso and Lombardo2013). Although beyond the aim of this paper, the different T peaks estimated by Angiboust et al. (Reference Angiboust, Langdon, Agard, Waters and Chopin2012) in the hanging wall (T peak of 480–500 °C) and in the footwall (T peak of 530–550 °C) of the GCSZ can be explained by considering that, after subduction, early exhumation of the MO was characterized by a strong decompression and a moderate cooling. Balestro et al. (Reference Balestro, Lombardo, Vaggelli, Borghi, Festa and Gattiglio2014) documented, in fact, that in metasedimentary rocks, occurring close to the tectonic contact with the overlying Queyras Schistes Lustrés Complex, the blueschist- to greenschist-facies metamorphic assemblage is characterized by a T of 500 °C, which is a value very similar to those calculated from metasediments sampled in the hanging wall of the GCSZ. Remarkably, metabasalt sampled away from the shear zone in the hanging wall gave a higher T peak (T = 510 ± 30 °C; Blake, Moore & Jayko, Reference Blake, Moore, Jayko, Coleman and Wang1995). The lowest T values from rocks sampled along the GCSZ are, however, an important point of discussion, which would suggest that a specific study about heat transfer across the shear zone and within rocks with different hydromechanical behaviours is needed. As a matter of fact, during continental collision the increase (and decrease) of temperature (and pressure) commonly occurs along significant shear zones which developed in mechanically heterogeneous sequences (see e.g. Li, Gerya & Burg, Reference Li, Gerya and Burg2010; Li, Reference Li2014; Reuber et al. Reference Reuber, Kaus, Schmalholz and White2016). Comparison of available P–T estimates (see Angiboust et al. Reference Angiboust, Langdon, Agard, Waters and Chopin2012 with ref., their fig. 9) for the eclogite-facies peak stage in the MO shows that the estimated P–T values are very similar as stated earlier, and differences could be partially related to different approaches used in the calculation.
The D1 and D2 deformation stages were followed by the D3 deformation developed during late exhumation of the MO, under lower-greenschist-facies conditions (Fig. 6d). Folding and shearing occurred in relation to the doming of the underlying Dora Maira Massif, which was driven by deep crust/mantle indentation (Lardeaux et al. Reference Lardeaux, Schwartz, Tricart, Paul, Guillot, Béthoux and Masson2006). This doming caused the progressive westward tilting of the previous D1 + D2 architecture (Fig. 6d). W-verging D3 folds developed in the footwall of new formed extensional shear zones. The main D3 structure corresponds to the VASZ, which cross-cut the meta-ophiolite sequence and the D2-related structural architecture in the northern sector of the MO (Fig. 6d). It is an extensional shear zone that, similarly to the GCSZ, developed along weak lithological contacts such as horizons of different compositions and rheology occurring within both the post-extensional metasediments and the BSZ. The VASZ is structurally associated with the tectonic contact which separates the MO from the overlying Queyras Schistes Lustrés Complex (i.e. the West Viso Detachment; Ballèvre, Lagabrielle & Merle, Reference Ballèvre, Lagabrielle and Merle1990; Tricart et al. Reference Tricart, Schwartz, Sue and Lardeaux2004). This tectonic contact is localized along a structural level which corresponds to the overturned limb of the first-order D2 antiformal syncline, and consists of talc-chlorite schist (i.e. the BSZ) and syn-extensional metasediments resting above metabasalt. Even along the macroscale structures that bound the MO, concentration and propagation of deformation are controlled by the inherited intra-oceanic tectonostratigraphic architecture and related occurrence of weak rocks.
5. Conclusions
Although subducted up to eclogite-facies metamorphic conditions, the MO represents a well-preserved continuous fragment of upper oceanic lithosphere and related Upper Jurassic – Lower Cretaceous sedimentary covers, which significantly records the evolution of an oceanic core complex formed by mantle exhumation along an intra-oceanic detachment fault (i.e. the BSZ). The documented Late Jurassic irregular physiography of this sector of the Ligurian–Piedmont basin ocean, which is well comparable with those observed in oceanic core complexes documented along the slow- (Atlantis Massif, Mid-Atlantic Ridge) and ultraslow-spreading (Atlantis Bank, Southern Indian Ridge) ridges (e.g. Cannat, Reference Cannat1993; Tucholke, Lin & Kleinrock, Reference Tucholke, Lin and Kleinrock1998; Boschi, Früh-Green & Delacour, Reference Boschi, Früh-Green and Delacour2006; Karson et al. Reference Karson, Früh-Green, Kelley, Williams, Yoerger and Jakuba2006; Dick, Tivey & Tucholke, Reference Dick, Tivey and Tucholke2008; Miranda & Dilek, Reference Miranda and Dilek2010), strongly influenced subduction- and exhumation-related Alpine tectonics up to the present-day structural setting of the MO.
Although strongly deformed during the different Alpine stages, the MO was not completely dismembered into different tectonic or tectonometamorphic units as suggested in previous interpretations. The occurrence of remnants of an intra-oceanic Jurassic detachment fault (the BSZ), sealed by the Lower Cretaceous post-extensional sequence, highlights that the BSZ was not significantly reactivated during the subduction-related Alpine stage (D1-related deformation), probably because it was not favourably oriented with respect to the orientation of the strain field. Conversely, the GCSZ represents a D2-related tectonic contact, developed during the continental collision stage (D2) along a particular detachment level enucleated along the long limb of macroscale D2 folds, conditioned by the inherited heterogeneous Upper Jurassic – Lower Cretaceous tectonostratigraphic physiography of the Ligurian–Piedmont oceanic basin. Its gradual decrease in displacement down to zero towards both the N and S and the juxtaposition of the same lithostratigraphic units along both its hanging wall and footwall, invalidate the former inferred role of the GCSZ in juxtaposing two different tectonometamorphic units recording different subduction histories.
Our findings show that recognizing inherited ocean-related architectures represents a key factor that is useful for reconstructing the whole tectonic evolution of meta-ophiolite units in orogenic belts. Our results demonstrate the importance of carrying out multidisciplinary analytical studies (e.g. structural, stratigraphic, petrological) on detailed geological maps, to fully understand the present-day architecture and the tectonometamorphic evolution of exhumed orogenic belts, and thus to better constrain palaeogeographic reconstructions.
Acknowledgements
This paper is dedicated to the memory of Bruno Lombardo who contributed greatly to advancement of geological knowledge of the Monviso meta-ophiolite Complex. We thank Editor G. Capponi for his careful editorial handling, and we would like to express our sincere thanks to the two anonymous reviewers for their constructive and thorough reviews, from which we have benefited greatly in revising our manuscript. Research has been supported by the Italian Ministry of University and Research (Cofin-PRIN 2010/2011 “GEOPROB – GEOdynamic Processes of Oceanic Basins” grant no. [2010AZR98L_002] to AF, and grant no. [2010AZR98L_008] to PT); and by the University of Torino (Ricerca Locale “ex 60 %–2014-2015”, Università degli Studi di Torino, to GB).