Intentional reductions in energy intake are frequently implemented by the general population and athletes alike, typically for the goal of body fat loss. One important consideration associated with such hypoenergetic dietary conditions is the potential loss of lean body mass( Reference Dulloo, Jacquet and Miles-Chan 1 ). Not only is lean mass critical for functional ability and athletic performance, but reductions in lean mass may drive overeating and promote regain of fat mass following weight loss( Reference Dulloo 2 ). In addition, maintaining lean mass could contribute to superior maintenance of energy expenditure due to its contribution to RMR( Reference Ravussin, Burnand and Schutz 3 ). Therefore, optimal fat loss programmes should promote maximal retention of lean body mass.
Dietary recommendations for fat loss typically involve daily energy restriction, meaning that a normal eating schedule and frequency is followed but smaller portions and less energy are consumed at each meal( Reference Varady 4 ). However, an alternative method of reducing food consumption has gained popularity over the past decade. This method, termed intermittent fasting (IF), employs repeated short-term fasts in an effort to reduce food consumption, modify body composition and improve overall health( Reference Tinsley and Bounty 5 ). These short-term fasts are longer than a typical overnight fast, but are typically no longer than 24 h in duration. An increasing body of research indicates that IF is effective for fat mass reduction, although some forms of IF may not be superior to traditional energetic restriction for long-term weight loss maintenance( Reference Tinsley and Bounty 5 – Reference Tinsley and Horne 7 ).
In addition to traditional concerns of retaining lean body mass during hypoenergetic conditions, IF programmes implement fasting periods that necessitate periods of 12–24 h without dietary amino acid-induced stimulation of muscle protein synthesis. During this time, it is expected that muscle protein breakdown exceeds muscle protein synthetic activity, producing a negative protein balance in skeletal muscle( Reference Rennie, Edwards and Halliday 8 ). Skeletal muscle tissue may be broken down in short-term fasting in order to provide amino acid substrate for hepatic gluconeogenesis( Reference Tsalikian, Howard and Gerich 9 ). Despite these concerns, we have previously demonstrated that resistance training can prevent the loss of lean body mass during IF programmes utilising 16- to 20-h fasting periods for 8 weeks( Reference Moro, Tinsley and Bianco 10 , Reference Tinsley, Forsse and Butler 11 ). However, known difficulties meeting physical activity recommendations in the general population necessitate the exploration of non-exercise strategies to ameliorate a potential loss of skeletal muscle tissue during fat loss programmes such as IF( Reference Lee, Shiroma and Lobelo 12 ).
One potential candidate is supplementation with the leucine metabolite β-hydroxy β-methylbutryate (HMB). HMB has been demonstrated to decrease proteolysis through modulation of the ubiquitin-proteasome and caspase protease systems in environments conducive to muscle loss( Reference Smith, Mukerji and Tisdale 13 – Reference Hao, Jackson and Wang 15 ). It has also been examined in the contexts of reducing muscle damage( Reference Shirato, Tsuchiya and Sato 16 , Reference Wilson, Kim and Lee 17 ) and myofibrillar proteolysis( Reference Nissen, Sharp and Ray 18 ). However, there are currently no reports examining the effects of HMB during short-term fasts, such as those employed during IF, in humans. These fasting periods elicit a unique metabolic state in which substrate utilisation and energy expenditure may be altered( Reference Tinsley and Bounty 5 , Reference Soeters, Soeters and Schooneman 19 ). Due to HMB’s demonstrated ability to reduce proteolysis, it could potentially promote retention of skeletal muscle mass during IF programmes.
Therefore, the primary aim of the present investigation was to examine whether HMB free acid (HMB-FA) alters a marker of muscle protein breakdown and select anabolic/catabolic hormones (i.e. cortisol and testosterone) during an acute 24-h fast. Secondary aims were to evaluate the hormonal and energy expenditure responses to a 24-h fast in order to inform future IF research. It was hypothesised that HMB-FA supplementation would lead to a reduction in myofibrillar proteolysis, as measured by 3-methylhistidine:creatinine ratio (3MH:CR). In addition, it was hypothesised that lower cortisol concentrations would be observed with HMB-FA relative to placebo, with reductions in testosterone in both conditions due to acute energy deprivation( Reference Rojdmark 20 ), leading to a higher testosterone:cortisol ratio (T:C) with HMB-FA supplementation. Finally, based on previous investigations reporting increases in resting energy expenditure (REE) after 24 to 48 h of fasting( Reference Zauner, Schneeweiss and Kranz 21 – Reference Norrelund, Nair and Jorgensen 24 ), it was hypothesised that REE would increase during the fasting period, independent of supplementation.
Methods
Overview
In a randomised, double-blind, placebo-controlled cross-over design, participants completed two conditions. Each condition consisted of a 3-d meat-free diet followed by a single 24-h fast (Fig. 1). During each 4-d condition, participants consumed three dietary supplement packets per day. Each packet contained either 1 g of β-hydroxy-β-methylbutyrate in FA form or placebo. Urine was collected for 8 h before the fast, as well as throughout the fast, for the analysis of 3MH, CR and HMB. Saliva was collected at the beginning and end of the fast for assessment of cortisol and testosterone. The cortisol awakening response (CAR) on the day of the fast was also assessed. Participants were provided with meat-free food items for the 3 d before the fast and reported to the laboratory after 16 h of fasting. Participants remained in the laboratory under observation from 16 to 24 h of fasting, during which sample collection and REE assessments took place. After a washout period of 3–10 d, the second condition was completed.

Fig. 1 Study design. In a randomised, placebo-controlled cross-over design, two conditions were completed: β-Hydroxy β-methylbutyrate (HMB) supplementation and placebo. Each condition lasted 4 d. During a 3-d meat-free diet, participants consumed three supplement packets/d, each containing 1 g of HMB free acid or placebo (). Beginning in the morning of 3rd day of consuming a standardised meat-free diet, participants collected their urine for 32 h, broken up into four 8-h collections. Urine was analysed for 3-methylhistidine, HMB and creatinine. Participants began a 24-h fasting period in the evening of the 3rd day of the meat-free diet. At five points during the 24-h fast, participants provided saliva samples for analysis of cortisol and testosterone (
). From 16 to 24 h of fasting, participants remained under researcher supervision. Resting energy expenditure (REE) was assessed at 16 and 24 h of fasting. After completion of the first condition, participants entered a washout period of 3–10 d before completing the second condition.
Participants
To be eligible for participation, individuals were required to meet the following criteria: 18–50 years of age, generally healthy (defined as no history of diabetes, CVD, or other acute or chronic health condition that could potentially be impacted by an acute 24-h fast), regular breakfast consumer (defined as consuming >200kcal (837kJ) before 10.00 hours on at least 5 d/week), weight stable (defined as no weight changes >5 kg in the past 3 months), non-smoker, not pregnant and no known allergies to food items used in the study.
Consent and baseline session
This study was conducted according to the guidelines laid down in the Declaration of Helsinki and all procedures involving human participants were approved by the Texas Tech University institutional review board (IRB00000276). Written informed consent was obtained from all participants. Baseline assessment of REE was conducted via indirect calorimetry (TrueOne 2400; ParvoMedics) for prescription of dietary intake, and body composition was estimated via multi-frequency bioelectrical impedance analysis (mBCA 514; Seca).
Meat-free diet
Before each 24-h fast, participants were required to follow a meat-free diet and consume the provided supplements for 3 d. The meat-free diet was necessary to ensure that urinary 3MH content was due to protein degradation within body tissues rather than dietary content( Reference Nissen, Sharp and Ray 18 , Reference Elia, Carter and Bacon 25 , Reference Elia, Carter and Bacon 26 ). Energetic intake was prescribed based on baseline REE assessments, using an activity factor of 1·5, and participants were instructed to consume the prescribed amount of energy and remain weight stable during this period. In order to promote compliance with the assigned diet, participants were provided with a selection of meat-free meals and snacks. Although the majority of food was provided by research personnel, participants were also allowed to consume their own meat-free items provided that they recorded all intake and remained within the energy intake requirements. Dietary records were reviewed by study personnel and analysed using manual entry of nutrition information from food labels, as well as data from the US Department of Agriculture SuperTracker tool( 27 ).
Supplementation
Beginning on the 1st day of the meat-free diet, participants were instructed to consume one packet of the provided dietary supplement three times per d with food (i.e. at breakfast, lunch and dinner). Each serving of HMB-FA and placebo was provided in identical white sachet packaging. The HMB-FA supplement (marketed as BetaTor®; Metabolic Technologies) contained Litesse® polydextrose, β-hydroxy β-methylbutyrate FA, reverse osmosis water, debittering agent, orange flavour, stevia extract, potassium carbonate and potassium sorbate. Each serving of the placebo contained a similar amount of Litesse® polydextrose, reverse osmosis water, maize syrup, debittering agent, orange flavouring, stevia extract, citric acid, potassium sorbate and xanthine gum powder. To assess compliance, urine was analysed for the concentration of HMB.
Fasting period
In each condition, the 24-h fasting period began in the evening on the 3rd day of the meat-free diet. All fasting periods began between 16.00 and 19.00 hours and terminated at the same time the following day. Participants were instructed to consume all food items for the 3rd day of the meat-free diet before the beginning of the fasting period. They were also asked to minimise physical activity and perform no exercise on the 3rd day of the meat-free diet or during the fasting period. Participants slept in their own homes and were unsupervised from 0 to 16 h of fasting (i.e. the evening after beginning the fast and overnight). However, participants reported to the laboratory upon waking and remained there under direct supervision of study personnel from 16 to 24 h of fasting. Participants were asked not to consume water after waking until they were provided water in the laboratory. Male participants were provided up to 1·5 litres of water during the period corresponding to 16 to 20 h of fasting, and an additional up to 1·5 litres during 20 to 24 h. Females were provided with up to 1·0 litre during each of these periods. During the first 24-h fast, the actual amount of water consumed during each 4-h period of time was recorded, and this precise amount of water was provided during the second 24-h fast.
Urine collection and analysis
Four consecutive 8-h urine collections took place: the final 8 h of the meat-free diet, 0–8 h of fasting, 8–16 h of fasting and 16–24 h of fasting. Participants were provided with clearly marked urine collection containers that specified which days and times each container should be used. The first three urine collections were performed by the participant outside of the laboratory, and the final urine collection took place in the laboratory. Participants were instructed to keep the urine collection containers in a refrigerator at all times when not in use until the containers were returned to study investigators.
Upon delivery to the laboratory by the participant, each urine collection was measured for total volume and mixed. Aliquots were obtained from each collection container and stored at −80°C until shipment (i.e. 0–2 months). At the conclusion of the study, urine samples were shipped on dry ice for analysis at a Clinical Laboratory Improvement Amendments certified laboratory (Heartland Assays Inc.). Urinary HMB was analysed by GC/MS as previously described( Reference Nissen, Van Koevering and Webb 28 ). Urine 3MH was determined by previously described GC–MS methods( Reference Rathmacher, Link and Flakoll 29 ). In brief, internal standard, 3-methyl-(methyl-2H3)-histidine (d3-3MH), is added to urine, acidified and absorbed onto cation exchange columns. It is then eluted from columns, dried and derivatised with MTBSTFA (Regis Technologies Inc.) for GC/MS. 3MH is monitored at 238·2 amu and 3-methyl-(methyl-2H3)-histidine (d3-3MH) at 241·2 amu. CR was measured using a colorimetric assay kit (Cayman Chemical).
Saliva collection and analysis
Each participant was familiarised with the saliva collection procedures at the baseline visit. Saliva collection took place using the passive drool method, with allows for saliva to be transferred from the mouth to a small vial according to manufacturer recommendations( 30 ). Five saliva samples were collected during each condition. The first and last sample were collected at the beginning and end of the 24-h fast, respectively. The sample at the beginning of the 24-h fast was collected by the participant in his or her home, and the sample at the end of the fast was collected under researcher supervision. Each participant was contacted by the principal investigator in the hours leading up to the at-home collections in order to confirm the exact time the samples should be collected. The time of sample collection was also recorded by study participants using a collection log provided by the researchers. An additional three saliva samples were collected the morning after the beginning of the fast. These samples were collected at 0, 30 and 45 min after waking and allowed for the evaluation of the CAR (i.e. the characteristic sharp increase then decrease in cortisol concentrations upon waking( Reference Stalder, Kirschbaum and Kudielka 31 )). The importance of collecting the saliva sample exactly as instructed was strongly emphasised to research participants. Participants were provided with paper signs to place by their bed and were instructed to set alarms for all three saliva collections in the morning. The actual time of sample collection was recorded by the research participant, and saliva samples were stored in the freezer until delivery to the laboratory.
Upon delivery to the laboratory, each vial of saliva was stored at −80°C until shipment (i.e. 0–2 months after collection). Samples were packaged according to recommended procedures and shipped to a saliva testing facility for analysis (Salimetrics LLC Saliva Lab). Samples were assayed in duplicate using the Salimetrics high sensitivity enzyme immunoassay kits for cortisol and testosterone, without modifications to the manufacturers’ protocol. The average CV for all samples tested was 5·6 % for cortisol and 7·2 % for testosterone, which meets the manufacturer’s criteria for accuracy and repeatability in salivary bioscience. Sample test volume was 25 μl of saliva per determination. The cortisol assay has a lower limit of sensitivity of 0·007 μg/dl (0·0193nmol/l), standard curve range from 0·012 to 3·0 μg/dl (0·331 to 82·67nmol/l). The testosterone assay has a lower limit of sensitivity of<1·0 pg/ml. The relationship between serum and salivary concentrations of cortisol and testosterone have been previously described( Reference Dorn, Lucke and Loucks 32 – Reference Nahoul, Rao and Scholler 35 ), and salivary testosterone exhibits a stronger correlation to serum free testosterone than total testosterone( Reference Vittek, L’Hommedieu and Gordon 36 ). The manufacturer-reported serum-saliva correlations for cortisol and testosterone are 0·91 and 0·96, respectively( Reference Salimetrics 37 , Reference Salimetrics 38 ).
Resting energy expenditure
During each condition, REE was assessed at 16 and 24 h of fasting. The indirect calorimeter was calibrated before each assessment according to manufacturer recommendations. Assessment procedures were based on the recommendations of Compher et al.( Reference Compher, Frankenfield and Keim 39 ). Participants rested in the supine position in a dimly lit room for the duration of testing. The temperature in the room was approximately 23°C, and participants were provided a blanket if desired. The initial 5 min of each test were discarded, and the test continued until the CV for VO2 and VCO2 was ≤7 % over a period of 5 consecutive minutes. The average CV for REE in all tests was 3·1 %.
Washout period
After completing the first condition, participants entered a washout period of 3–10 d before completing the second condition. The variable duration between conditions was necessary due to participant availability. Participants were instructed to maintain their normal lifestyle and attempt to remain weight stable during the washout period.
Statistical analysis
To estimate the required sample size, an a priori power analysis was performed for using 3MH as the dependent variable of interest (G*Power version 3.1.9.2). An effect size was estimated from information contained in a previous study of HMB supplementation and 3MH excretion( Reference Nissen, Sharp and Ray 18 ). Using effect sizes of 0·3–0·35, an α error probability of 0·05, and power of 0·8, it was estimated that ten to twelve participants were needed to detect changes in 3MH.
Data were examined for potential outliers via studentised residuals, and normality of data were assessed using the Shapiro–Wilk test. Residuals ≥±3 were considered outliers. No outliers were present for testosterone, cortisol, T:C, REE or RQ. One outlier was present for 3MH:CR. The analyses were performed with and without this participant’s urinary data, and the outcome did not differ (i.e. the lack of statistical significance did not change). REE, RQ and T:C were normally distributed, but some violations of normality were present for 3MH:CR, testosterone and cortisol. Therefore, log10 transformations were applied to the data, which resolved the initial violations of normality. Results from the analyses with the raw and transformed data were compared. No differences in the results for 3MH:CR, testosterone, or cortisol were present. Therefore, the results of the raw data analyses are presented for ease of interpretation. 3MH:CR was analysed using 2×4 (condition×time) ANOVA with repeated measures.
Due to known sex differences in concentrations and responses of the hormones of interest( Reference Paris, Franco and Sodano 40 ), testosterone, cortisol and T:C were analysed using three-way mixed ANOVA with condition and time specified as within-subjects factors and sex as the between-subjects factor. To evaluate the CAR, two analyses were performed. First, a three-way mixed ANOVA was performed using cortisol concentrations immediately upon waking, 30 min after waking, and 45 min after waking. In addition, the AUC was calculated using the trapezoid method. CAR AUC was analysed using two-way mixed ANOVA. REE and RQ were analysed using two-way ANOVA with repeated measures. To follow-up significant interactions in these analyses, two-way ANOVA with repeated measures, one-way ANOVA, paired or independent samples t tests, and pairwise comparisons were used as appropriate. Average dietary intake over the 3 d before the fast was compared via paired samples t tests. To examine compliance with the supplementation assignments, urinary HMB concentrations between conditions were compared via two-way ANOVA. Statistical significance was set at P≤0·05. Cohen’s d effect sizes were calculated for major dependent variables.
Due to the cross-over design, carryover effects were evaluated via a linear mixed model for each dependent variable of interest (3MH:CR, cortisol, testosterone, CAR AUC, REE and RQ). The condition, sequence, carryover, and time point were specified as fixed effects and the participant nested within the sequence was specified as a random effect, as recommended( 41 ). There were no statistically significant carryover effects for any major dependent variable (3MH:CR: P=0·24, 24-h cortisol: P=0·69, CAR: P=0·09, CAR AUC: P=0·08, testosterone: P=0·71, T:C: P=0·98, REE: P=0·14 and RQ: P=0·52).
Results
Participant characteristics are presented in Table 1. All participants were compliant with the meat-free diet based on interview and diet records. During the 3 d before the fast, there were no significant differences between conditions for dietary intake (average daily intake: 9406 (sd 2402) kJ (2248 (sd 574) kcal), 75 (sd 26) g fat, 315 (sd 80) g carbohydrate and 79 (sd 31) g protein). Urinary HMB concentrations were significantly greater in the HMB-FA condition (12 555 (sd 14 542) nmol/ml; range: 2244–28 202) than the PL condition (281 (sd 183) nmol/ml; range: 23–470; P=0·002), indicating compliance with the supplementation protocol.
Table 1 Participant characteristics (Mean values and standard deviations)

Changes in 3MH:CR during the fast were not statistically significant and were unaffected by HMB supplementation (Table 2). In the HMB-FA condition, most participants experienced changes in 3MH:CR between −4 and +26 %, although three individual participants had larger increases (+94, 138 and 314 %). In the PL condition, most participants experienced changes in 3MH:CR between −24 and +33 %, although one participant had a decrease of 74 % and two had larger increases (+216 and 307 %).
Table 2 3-Methylhistidine:creatine (3MH:CR) ratio during fastingFootnote * (Mean values and 95 % confidence intervals)

HMB-FA, β-hydroxy β-methylbutyrate free acid; PL, placebo.
* Data were analysed using two-way ANOVA with repeated measures.
A significant time by sex interaction was present for cortisol concentrations at the beginning and end of the fast (P=0·01), but no significant differences between conditions were present. Cortisol was higher in males than females at the beginning of the 24-h fast (P=0·05), but did not differ at 24 h of fasting (P=0·20). In addition, cortisol exhibited apparently disparate, although not statistically significant, responses in males and females (Fig 2(a) and (c)). A condition by time interaction was present for the CAR (P=0·03; Fig. 2(b)). In both conditions, cortisol increased from waking to 30 min post-waking (PL: +60 %, d=1·4, P=0·01; HMB-FA: +33 %, d=0·8, P=0·01). Cortisol was reduced from 30 to 45 min after waking in HMB-FA (−32 %, d=−1·0, P=0·04), but not PL (−6 %, d=−0·2, P=0·14). However, no condition by sex interaction or condition effects were seen for CAR AUC (P=0·75 and P=0·82, respectively). No significant changes in testosterone were seen during the fast in either condition (Table 3).
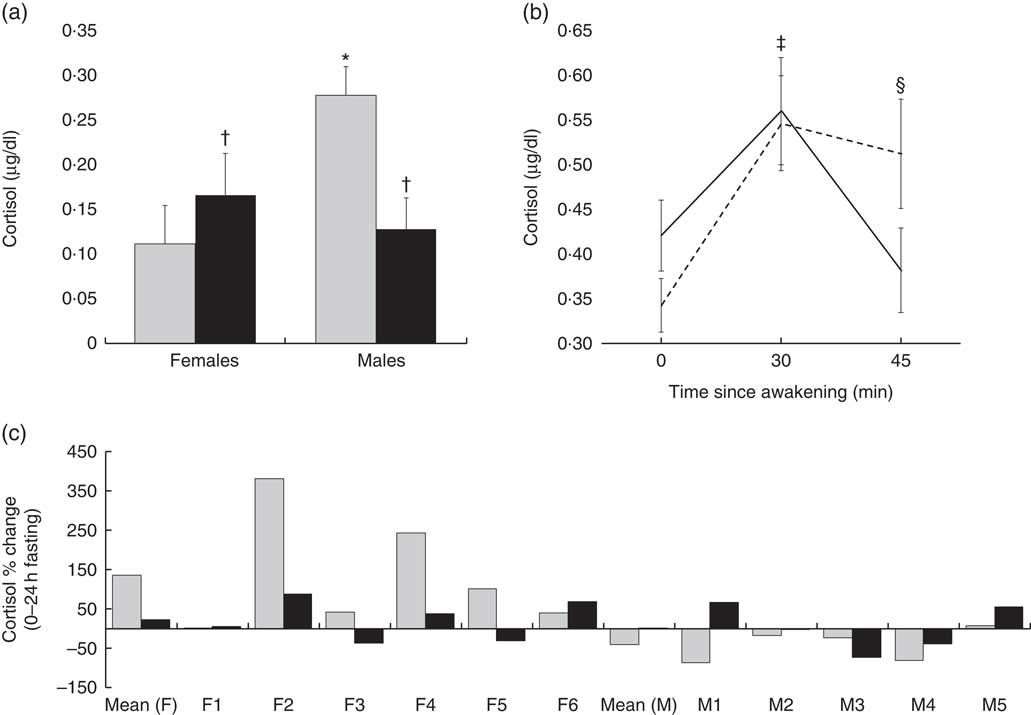
Fig. 2 Cortisol responses. Cortisol was evaluated at the beginning and end of the 24-h fast, as well as part of the cortisol awakening response (i.e. samples taken upon waking and 30 and 45 min after waking). (a) A three-way mixed ANOVA revealed the presence of a significant time×sex interaction for cortisol concentrations at 0 () and 24 h (
) of fasting (P=0·01). Follow-up via paired samples t tests revealed non-significant increases of cortisol in females (+50 %; d=0·9, P=0·08) and decreases of cortisol in males (−54 %, d=−0·9, P=0·09). (b) A three-way mixed ANOVA revealed the presence of a significant condition by time interaction for the cortisol awakening response (P=0·03). Follow-up analysis indicated that cortisol increased significantly from waking to 30 min post-waking in both conditions (P=0·01 for both). However, cortisol was reduced from 30 to 45 min after waking in β-hydroxy β-methylbutyrate free acid (HMB-FA,
) (−32 %, d=−1·0, P=0·04), but remained elevated in the placebo (PL,
) condition (−6 %, d=−0·2, P=0·14). (c) Mean and individual cortisol responses between 0 and 24 h of fasting are displayed. F, female; M, male; c:
, HMB-FA;
, PL. Values are means and standard deviations represented by vertical bars. * P=0·05 (comparison with 0 h). † 0·05<P<0·10 (comparison with 0 h). ‡ P=0·01 in both conditions (comparison with 0 min). § P<0·05 in PL condition only (comparison with 0 min). To convert cortisol in μg/dl to nmol/l, multiply by 27·59.
Table 3 Hormonal responses to fastingFootnote † (Mean values and 95 % confidence intervals)

F, females; M, males; HMB-FA, β-hydroxy β-methylbutyrate free acid; PL, placebo.
* P<0·05, ** P<0·005.
† Data were analysed using three-way mixed ANOVA. One-way ANOVA with repeated measures and pairwise comparisons were used to follow-up the time×sex interaction for cortisol (see text for details).
‡ To convert cortisol in μg/dl to nmol/l, multiply by 27·59.
A significant three-way interaction was present for T:C (P=0·009). Follow-up indicated that a simple two-way interaction (condition by time) was present in males (P=0·05), but not females (P=0·20). Paired samples t tests revealed that the T:C increased from 0 to 24 h of fasting in the HMB-FA condition (+162 %, d=3·0, P=0·001), but not the PL condition (+13 %; d=0·4, P=0·60; Fig. 3(a)). Although T:C was 48·3 % lower on average in HMB-FA than PL at the beginning of the fast, this was not statistically significant (P=0·12). In addition, the difference between HMB-FA and PL at 24 h of fasting was not significant (P=0·21) although T:C in HMB-FA was 19·9 % higher than PL on average. Individual responses in T:C are shown in Figs. 3(b)–(e).

Fig. 3 Testosterone:cortisol (T:C) ratio responses. The T:C ratio was determined at the beginning and end of the 24-h fast and analysed using three-way mixed ANOVA. (a) A three-way interaction was present for T:C (P=0·009). Follow-up via two-way ANOVA with repeated measures indicated that a condition by time interaction was present in males (P=0·05), but not females (P=0·20). In males, the T:C ratio increased from 0 () to 24 h (
) of fasting in the hydroxy β-methylbutyrate free acid (HMB-FA) condition (+162 %, d=3·0, P=0·001) but not in the placebo (PL) condition (+13 %; d=0·4, P=0·60). The difference in T:C between HMB-FA and PL was not statistically significant at the beginning (P=0·12) or end (P=0·21) of the fast. Individual responses in T:C for females in the PL (b) and HMB-FA conditions (c), as well as males in the PL (d) and HMB-FA conditions (e) are displayed. * P=0·001 (comparison with 0 h). Values are means and standard deviations represented by vertical bars. F, female; M, male.
No differences between HMB-FA and PL were seen for indirect calorimetry measures. However, a time main effect was present for REE, indicating that it was higher at 24 h of fasting than 16 h (+4·0 %, d=0·3, P=0·04). The magnitude of change was 293 kJ/d (70 kcal/d) on average, but individual changes ranged from −4·5 to +17 % (−91 to +320 kcal/d). RQ did not change from 16 to 24 h of fasting (RQ=0·79 and 0·80; P=0·55).
Discussion
The primary purpose of this investigation was to evaluate the impact of HMB-FA on muscle protein breakdown, cortisol and testosterone during acute fasting. The major findings were that neither fasting nor HMB-FA alter myofibrillar protein degradation during the first 24 h of fasting, as indicated by urinary 3MH:CR, but HMB-FA influences the CAR in both sexes and T:C in males. The lack of increase in 3MH:CR observed in the present study prevents a conclusion concerning the effects of HMB during fasting durations that elicit increased muscle catabolism. Early literature indicated that protein catabolism did not increase until the 3rd day of fasting( Reference Benedict, Goodall and Ash 42 ), but more recent investigations have reported increased whole body or skeletal muscle proteolysis after 30 to 72 h of fasting( Reference Tsalikian, Howard and Gerich 9 , Reference Nair, Woolf and Welle 43 – Reference Vendelbo, Moller and Christensen 46 ). In contrast to these early fasting periods, it has long been known that muscle protein is well-conserved during longer-term fasting in order to prevent wasting and functional impairment( Reference Benedict, Goodall and Ash 42 , Reference Cahill 47 , Reference Saudek and Felig 48 ). Indeed, by 7 d of fasting, whole body proteolysis is lower than after only 12 h of fasting( Reference Henson and Heber 49 ). However, the temporal changes in protein balance during fasts of the duration often employed in IF programmes (i.e. ≤24 h) have not been fully elucidated. Giesecke et al.( Reference Giesecke, Magnusson and Ahlberg 50 ) reported that 24-h 3MH excretion increased on the 2nd day of fasting in 6 non-obese males and females. However, all urine from each 24-h period was combined, precluding an appropriate determination of whether 3MH began to increase during the later hours of the 1st day of fasting. In addition, 3MH excretion was not expressed relative to CR or muscle mass, as is recommended( Reference Seashore, Huszar and Davis 51 ). Other investigations of 3MH excretion during fasting have either failed to exert sufficient control of the antecedent diet or neglected to evaluate changes in excretion during the first 24 h of fasting( Reference Elia, Carter and Bacon 26 , Reference Young, Haverberg and Bilmazes 52 , Reference Lowry, Horowitz and Jeevanandam 53 ). Although the results of the present study indicate that myofibrillar protein degradation is not significantly elevated after 24 h of fasting, it is possible that more advanced techniques, such as analysis of the absolute breakdown rate within skeletal muscle( Reference Hector, McGlory and Damas 54 ), could have revealed changes.
In the present investigation, the T:C increased with HMB-FA supplementation, but not placebo, during 24 h of fasting in males. However, this result should be viewed critically. Although not statistically significant, T:C was 48 % lower in the HMB-FA condition at the commencement of the fast. Although the cause of this depression is not readily apparent, the significant increase in T:C during the fast is likely due to the relatively lower starting point as compared with placebo. In addition, although T:C was approximately 20 % higher with HMB-FA than placebo at the end of the fast, the difference between conditions was not statistically significant. Finally, it should be noted that the increase of T:C was driven by greater reductions in cortisol rather than increases in testosterone. The differential effects on T:C could be interpreted as an indication of a greater shift towards the catabolic actions of cortisol in the absence of HMB-FA supplementation; however, the substantive physiological impact of this change is highly questionable. T:C has previously been used as an indicator of anabolic/catabolic balance( Reference Urhausen, Gabriel and Kindermann 55 ), although the physiological roles of these hormones are multifaceted( Reference Lee, Kim and Choi 56 , Reference Kelly and Jones 57 ). Limited information is available concerning the modulation of T:C in dietary programmes incorporating fasting, although one investigation found that this ratio did not change during Ramadan IF in middle-distance runners( Reference Chennaoui, Desgorces and Drogou 58 ). The majority of previous research has indicated that HMB supplementation does not reduce cortisol levels in the context of acute( Reference Wilson, Lowery and Joy 59 ) or chronic( Reference Durkalec-Michalski and Jeszka 60 – Reference Crowe, O’Connor and Lukins 64 ) exercise, although some investigations have indicated that HMB-FA may reduce cortisol concentrations relative to placebo during a resistance training programme( Reference Wilson, Lowery and Joy 65 , Reference Asadi, Arazi and Suzuki 66 ). However, prior investigations have not reported the effects of HMB in the context of acute fasting.
An examination of the CAR revealed a more rapid decline in cortisol after peak morning concentrations with HMB-FA than PL in both sexes. However, no difference in the AUC for cortisol was observed. The CAR is used to assess the function of the hypothalamus–pituitary–adrenal (HPA) axis by integrating circadian rhythms upon waking with a reactivity index (i.e. the response to the stress of awakening)( Reference Stalder, Kirschbaum and Kudielka 31 ). Abnormal activity of the HPA axis results in alterations of its end product, cortisol, and can lead to a variety of adverse effects on body composition and health( Reference Chrousos 67 ). Importantly, the CAR is believed to be distinct from other cortisol measures taken throughout the day, indicating that it provides additional information beyond these measurements( Reference Stalder, Kirschbaum and Kudielka 31 ). Although we observed modification of the CAR in response to HMB-FA supplementation, the tangible importance of this acute hormonal change is uncertain.
Previous reports have indicated that alterations of metabolic rate may occur during fasts that extend beyond a typical overnight duration. Relative to an overnight fast, increases in REE of 3·6–13 % have been reported after 36–48 h of fasting( Reference Zauner, Schneeweiss and Kranz 21 – Reference Norrelund, Nair and Jorgensen 24 ). In addition, Klein et al.( Reference Klein, Sakurai and Romijn 23 ) reported that REE did not change from 12 to 18 h of fasting, but increased by 5·9 % from 18 to 24 h of fasting in young adult males. Furthermore, they reported that over the course of a 72-h fast, the greatest increases in lipolysis and fat oxidation occurred between 18 and 24 h of fasting. In the present study, we report that REE increased by 4·0 % from 16 to 24 h of fasting in males and females. The body of evidence describing acute elevations in REE and alterations in lipid kinetics between 16 and 24 h of fasting may provide a theoretical rationale for IF programmes whose fasts extend to 24 h in duration( Reference Tinsley and Bounty 5 ). Furthermore, Wijngaarden et al. reported altered expression of over 900 genes in skeletal muscle after 24 h of fasting( Reference Wijngaarden, Bakker and van der Zon 68 ), indicating that major molecular responses occur over this duration of food deprivation.
The present investigation has several limitations. The responses to acute food deprivation in those unaccustomed to fasting may be different than those who are habituated to this style of eating. As such, the results of this investigation should be interpreted with caution when considering regular incorporation of fasts, such as in IF programmes. In addition, our sample size was relatively small and the participants had a broad range of body types. It is possible that a larger sample size would allow for detection of differences based on body size or adiposity. Although some sex differences were detected, it is possible that greater numbers would allow for more thorough evaluation of these potential differences for each dependent variable. While 3MH:CR is generally accepted as a surrogate measure of myofibrillar proteolysis, more direct measures are available. As such, it is possible that fasting or HMB-FA supplementation exerted effects on catabolic processes in muscle that we were unable to detect. Alternatively, it is possible that the short duration of HMB-FA supplementation was insufficient to exert measurable effects on skeletal muscle. Although the period from 16 to 24 h of fasting was highly controlled, our study did rely on some aspects of self-report outside of this time period. Future research should clarify the metabolic events in the early stages of fasting (≤24 h), which may provide a stronger theoretical rationale for an optimal fasting duration in IF programmes, as well as evaluating whether dietary supplementation holds promise for modulating muscle processes in a way that could enhance the benefits of IF. Finally, due to evidence that HMB may promote insulin resistance in rodent models( Reference Yonamine, Teixeira and Campello 69 , Reference Nunes, Goncalves-Neto and Ferreira 70 ), the impact of this supplement on insulin sensitivity and glucose handling in fasting humans should be considered in future research.
In conclusion, HMB-FA supplementation did not alter myofibrillar proteolysis during the first 24 h of fasting in the present study. However, supplementation did modify the CAR in both sexes and the T:C in males. REE increased from 16 to 24 h of fasting regardless of supplementation. These results should be confirmed and expanded upon in future research in order to inform IF interventions to optimise body composition and health.
Acknowledgements
The authors would like to acknowledge John Rathmacher, Supriya Gaitonde, Danielle Hardin, Victor Uribe, Austin Martin and Tyler Cook for their assistance with various aspects of this study. They would also like to acknowledge the participants for their dedication and effort.
Metabolic Technologies Inc. (MTI) provided the dietary supplements for this study and paid for the urinary analysis at Heartland Assays Inc., a subsidiary of MTI. Before this research project, a formal research agreement was established between Texas Tech University and MTI. MTI provided a small contribution to the study design by recommending the duration of meat-free diet and urine collection schedule. MTI played no other role in the study design and had no role in the data analysis, decision to publish or preparation of the manuscript for publication.
G. M. T. designed the study and obtained support for the research, oversaw all data acquisition, performed all statistical analyses and wrote the manuscript. A. H. G., A. J. G., M. I. V. and A. G. C. made substantial contributions to data acquisition and aided in revision of the manuscript. All authors read and approved the final version of the submitted manuscript.
The authors declare that there are no conflicts of interest.