Introduction
Together with allanite-(Ce), monazite-(Ce) and xenotime-(Y) are the most important carriers of rare earth elements (REE) in common magmatic rocks and metamorphic rocks with granitic and metapelitic protoliths (e.g. Bea, Reference Bea1996; Förster, Reference Förster1998a, Reference Förster1998b; Spear and Pyle, Reference Spear, Pyle, Kohn, Rakovan and Hughes2002; Gieré and Sorensen, Reference Gieré, Sorensen, Liebscher and Franz2004). A detailed study of their composition, stability and breakdown processes provides us with an understanding of REE relationships and processes that occur during petrogenesis and evolution of the parental rock (Wark and Miller, Reference Wark and Miller1993; Bea, Reference Bea1996; Gratz and Heinrich, Reference Gratz and Heinrich1997; Heinrich et al., Reference Heinrich, Andrehs and Franz1997; Förster, Reference Förster1998a, Reference Förster1998b; Poitrasson et al., Reference Poitrasson, Hanchar and Schaltegger2002; Pyle et al., Reference Pyle, Spear, Rudnick and McDonough2001; Spear and Pyle, Reference Spear, Pyle, Kohn, Rakovan and Hughes2002; Wing et al., Reference Wing, Ferry and Harrison2003; Kohn and Malloy, Reference Kohn and Malloy2004; Ondrejka et al., Reference Ondrejka, Uher, Putiš, Broska, Bačík, Konečný and Schmiedt2012, Reference Ondrejka, Putiš, Uher, Schmiedt, Pukančík and Konečný2016; Berger et al., Reference Berger, Gnos, Janots, Fernandez and Giese2008; Janots et al., Reference Janots, Engi, Berger, Allaz, Schwartz and Spandler2008; Uher et al., Reference Uher, Ondrejka and Konečný2009, Reference Uher, Ondrejka, Bačík, Broska and Konečný2015; Harlov et al., Reference Harlov, Wirth and Hetherington2011 and references therein). In addition, monazite-(Ce) and xenotime-(Y) are essential for petrochronology (e.g. Parrish, Reference Parrish1990; Montel et al., Reference Montel, Foret, Veschambre, Nicollet and Provost1996; Williams et al., Reference Williams, Jercinovic and Hetherington2007; Engi, Reference Engi, Kohn, Engi and Lanari2017).
Although these minerals commonly crystallise as primary magmatic or metamorphic accessory phases, younger post-magmatic or metamorphic hydrothermal fluids can cause their partial-to-complete transformation to low-grade neoblasts. For example, the commonly observed breakdown of monazite-(Ce) includes a subsolidus retrograde corona around its partly dissolved core with distinctive concentric mineral zones of apatite ± Th-silicate/oxide, and allanite-(Ce) to REE-rich epidote or clinozoisite (e.g. Broska and Siman, Reference Broska and Siman1998; Finger et al., Reference Finger, Broska, Roberts and Schermaier1998, Reference Finger, Krenn, Schulz, Harlov and Schiller2016; Budzyń et al., Reference Budzyń, Hetherington, Williams, Jercinovic and Michalik2010; Ondrejka et al., Reference Ondrejka, Uher, Putiš, Broska, Bačík, Konečný and Schmiedt2012, Reference Ondrejka, Putiš, Uher, Schmiedt, Pukančík and Konečný2016; Upadhyay and Pruseth, Reference Upadhyay and Pruseth2012; Lo Pò et al., Reference Pò D., Braga, Massonne, Molli, Montanini and Theye2016; Ji et al., Reference Ji, Harlov and Wang2021). This micro-texture is documented mostly in low- to medium-temperature metagranitoids with primary magmatic monazite-(Ce). However, it can also occur in metapelites if the high-temperature metamorphic monazite-(Ce) destabilises during post-peak metamorphic evolution (Majka and Budzyń, Reference Majka and Budzyń2006; Finger et al., Reference Finger, Krenn, Schulz, Harlov and Schiller2016; Lo Pò et al., Reference Pò D., Braga, Massonne, Molli, Montanini and Theye2016). Similar reaction coronae around xenotime-(Y) in granitoids and metapelites (Broska et al., Reference Broska, Williams, Janák and Nagy2005; Majka and Budzyń, Reference Majka and Budzyń2006; Janots et al., Reference Janots, Engi, Berger, Allaz, Schwartz and Spandler2008; Majka et al., Reference Majka, Pršek, Budzyń, Bačík, Barker and Łodziński2011; Broska and Petrík, Reference Broska and Petrík2015; Budzyń et al., Reference Budzyń, Sláma, Kozub-Budzyń, Konečný, Holický, Rzepa and Jastrzębski2018; Hentschel et al., Reference Hentschel, Janots, Trepmann, Magnin and Lanari2020), or the partial compositional alteration of xenotime-(Y) via dissolution–reprecipitation resulting in a texture characterised by thorite (ThSiO4) and uraninite (UO2) inclusions (Hetherington and Harlov, Reference Hetherington and Harlov2008; Ondrejka et al., Reference Ondrejka, Putiš, Uher, Schmiedt, Pukančík and Konečný2016), are less frequent. Experiments suggest the significant dissolution, etching, and alteration of monazite-(Ce) and xenotime-(Y) by common metamorphic and igneous fluids, and the formation of porous textures and various secondary phases, are dependent on the P–T–X conditions (Hetherington et al., Reference Hetherington, Harlov and Budzyń2010; Harlov et al., Reference Harlov, Wirth and Hetherington2011; Harlov and Wirth, Reference Harlov and Wirth2012; Budzyń et al., Reference Budzyń, Harlov, Williams and Jercinovic2011, Reference Budzyń, Harlov, Kozub-Budzyń and Majka2017), as well as on the presence of low-T metamorphic or post-magmatic-hydrothermal fluids in granitic systems (Budzyń and Kozub-Budzyń, Reference Budzyń and Kozub-Budzyń2015).
In this investigation, partially-decomposed primary magmatic monazite-(Ce) and xenotime-(Y) in mylonitised metagranites from the Variscan basement of the Fabova Hoľa granitic complex in the Veporic Unit, Central Slovakia are reported and interpreted (Fig. 1). Whereas retrograde destabilisation of monazite-(Ce) and remobilisation of light rare earth elements (LREE) results in an impressive corona of fluorapatite ± Th-silicate–allanite-(Ce)–clinozoisite, decomposition of xenotime-(Y) results in a novel type of reaction corona, including fluorapatite, hellandite-(Y) and hingganite-(Y) mineral zones, involving heavy rare earth elements (HREE) + Y, and also B and Be mobility. In this investigation, textural and compositional data, X-ray mapping, Th–U–total Pb age determination of monazite-(Ce) and Raman spectra all contribute to understanding the stability and breakdown products of REE, B and Be accessory minerals, their evolution, and behaviour during fluid-driven processes. Consequently, we describe a possible scenario regarding their origin and fluid sources responsible for their alteration. Moreover, the unusual Y–B–Be silicate reaction corona minerals, hellandite and hingganite, around xenotime-(Y) are documented for the first time.

Fig. 1. Geology of the Veporic Unit of the Central Western Carpathians (modified from Ondrejka et al., Reference Ondrejka, Putiš, Uher, Schmiedt, Pukančík and Konečný2016). Compiled on the basis of the 1:500,000 scale geological map of Slovakia (Biely et al., Reference Biely, Bezák, Elečko, Kaličiak, Konečný, Lexa, Mello, Nemčok, Potfaj, Rakús, Vass, Vozár and Vozárová1996). Subdivision of tectonic units from top to bottom: 1 – Upper Cretaceous, Palaeogene, Neogene and Quaternary sedimentary rocks, undivided (post-tectonic cover); 2 – Neogene volcanic and volcanoclastic rocks; 3 – Permian to Mesozoic sedimentary rocks undivided, in nappe position; 4 – Early to Late Palaeozoic – low-grade, metamorphosed sedimentary and volcanic rocks (Gemeric basement-cover complex, undivided; Lower Variscan Unit); 5 – Late Palaeozoic to Mesozoic para-autochthonous low-grade metamorphosed sedimentary and volcanic rocks of the South Veporic cover (Revúca Group, Foederata Unit); 6 – Permian to Mesozoic subautochthonous anchi- to low-grade, metamorphosed sedimentary and volcanic rocks of the North Veporic cover; 7 – Permian porphyric granites of the Hrončok type; 8 – Variscan granites, granodiorites and tonalites; 9 – gneisses to migmatites, orthogneisses, amphibolites, layered amphibolites, metagabbros, eclogites, metaperidotites, microgranites, pegmatites and aplites (Upper Variscan Unit); 10 – mica schists to gneisses, rare amphibolites (Middle Variscan Unit); 11 – phyllites, mica schists, greenschists to amphibolites, metasandstones, metavolcanics and metacarbonates (Lower Variscan Unit); 12 – Tatric basement-cover complex, undivided (Upper Variscan Unit); 13 – geological boundaries and faults: proved or assumed; 14 – nappe boundaries; 15 – ground elevation; 16 – town or city; and 17 – Fabova Hoľa metagranite (FAH-3 sample).
Geological setting
Regional geology
The Variscan basement structure of the Western Carpathians is composed of several structural units that are recognisable, even though there has been Alpine, mainly Cretaceous, tectono-metamorphic overprinting (Putiš, Reference Putiš1992). The Alpine structure of the Inner Western Carpathians is related to the evolution of the Cretaceous collisional wedge. The south–north progradation of this wedge has been dated between ca. 130 and 50 Ma according to 40Ar–39Ar radiometric age determination of phengitic white mica and muscovite from the thrust-fault shear zones (Putiš et al., Reference Putiš, Frank, Plašienka, Siman, Sulák and Biroň2009b).
The REE accessory minerals investigated occur in mylonitised granites of the Variscan basement, which are included in the Late Cretaceous Veporic Unit of the Inner Western Carpathians (e.g. Plašienka et al., Reference Plašienka, Grecula, Putiš, Kováč, Hovorka, Grecula, Hovorka and Putiš1997). Calc-alkaline tonalites to granites of I/S-type affinity intruded into the Early Palaeozoic crystalline basement complex of paragneisses, granitic orthogneisses, amphibolites, rare eclogites, calc-silicate marbles and migmatites of the Upper Variscan structural Unit or the Tatra Nappe (Putiš Reference Putiš1992; Bezák Reference Bezák1994; Putiš et al., Reference Putiš, Kotov, Petrík, Korikovsky, Madarás, Salnikova, Yakovleva, Berezhnaya, Plotkina, Kovach, Lupták and Majdán2003, Reference Putiš, Sergeev, Ondrejka, Larionov, Siman, Spišiak, Uher and Paderin2008, Reference Putiš, Frank, Plašienka, Siman, Sulák and Biroň2009b; Janák et al., Reference Janák, Méres and Ivan2007). Recent in situ U–Pb radiometric age determination in zircon indicates that their meso-Variscan, Upper Devonian to Carboniferous age interval of emplacement was ~365–350 Ma (Gaab et al., Reference Gaab, Poller, Janák, Kohút and Todt2005; Broska et al., Reference Broska, Petrík, Be´eri-Shlevin, Majka and Bezák2013; Kohút and Larionov Reference Kohút and Larionov2021).
Granitic rocks also occur in the form of individual small intrusions crosscutting the basement rocks (Ondrejka et al., Reference Ondrejka, Uher, Putiš, Kohút, Broska, Larionov, Bojar and Sobocký2021 and references therein). Similarly, dykes of Permian acidic-to-basic volcanic rocks, together with microgranites, intruded the crystalline basement rocks, or occur as intercalations within the Permian sedimentary successions (Kotov et al., Reference Kotov, Miko, Putiš, Korikovsky, Salnikova, Kovach, Yakovleva, Bereznaya, Král’ and Krist1996; Vozárová et al., Reference Vozárová, Rodionov, Vozár, Lepekhina and Šarinová2016). These are covered by Triassic metaquartzites, marbles and calc-schists of the sedimentary cover (Foederata Group) of the Veporic Unit crystalline basement (Plašienka et al., Reference Plašienka, Grecula, Putiš, Kováč, Hovorka, Grecula, Hovorka and Putiš1997).
Intrusions and lava flows of Miocene calc-alkaline diorites and andesites (~12 Ma; Konečný et al., Reference Konečný, Konečný, Kubeš and Pécskay2015a, Reference Konečný, Konečný, Kubeš and Pécskay2015b) belong to the youngest Neo-Alpine magmatic event in the part of the Veporic Unit studied. They also intruded the Variscan granitic rocks of the Fabova Hoľa Massif. These plutonic and volcanic rocks originated as a consequence of subduction of the Outer Western Carpathian basement underneath the Inner Western Carpathians (Pécskay et al., Reference Pécskay, Lexa, Szakacs, Seghedi, Balogh, Konečný, Zelenka, Marinel, Póka, Fülöp, Márton, Panaiotu and Cvetković2006; Lexa et al., Reference Lexa, Seghedi, Németh, Szakács, Konečný, Pécskay, Fülöp and Kovacs2010).
Petrographic characterisation of the metagranitic rocks
The Veporic crystalline basement south of the Late Cretaceous Pohorelá tectonic line consists mostly of metagranitoids (tonalites, granodiorites and porphyric-to-homogeneous granites) of the meso-Variscan Vepor pluton (Kohút et al., Reference Kohút, Uher, Putiš, Sergeev and Antonov2008). Relics of magmatic minerals, such as quartz, plagioclase, K-feldspar, biotite, muscovite, fluorapatite, zircon and REE accessory minerals occur in the newly formed or recrystallised mineral assemblage of quartz, albite, Ti-poor biotite, celadonite-rich muscovite (‘phengite’), chlorite, epidote, clinozoisite, calcite, titanite, rutile, and several tiny euhedral garnets that define the mylonitic foliation (Putiš, Reference Putiš1994). The lineation of the mylonitic foliation is defined by newly formed biotite, chlorite, and sericitic phengite in a roughly E–W (to WNW–ESE) direction. There are common transitions from granitic and tonalitic protomylonites to augen granite–gneisses (mylonites) and phyllonites (blastomylonites). These micro-structures indicate the ductile behaviour of quartz and the semi-ductile behaviour of feldspars in the mylonites. They reflect mostly the last top-to-the E(ESE) extensional deformation along the ductile low-angle normal faults in the basement and cover rocks during post-metamorphic exhumation (Putiš, Reference Putiš1994). The mylonitisation processes had an isochemical-to-allochemical character with increasing deformation in two different stages. The first stage of deformation, which was the transition from protomylonite to mylonite, usually is of an isochemical character, with restricted chemical changes. During the second stage, which was the transition from mylonite to ultramylonite/blastomylonite, local deformation occurred with the loss of Ca and Na and the gain of K, Mg (± Fe) in the mylonite/blastomylonite due to higher ductile strain localisation and increased fluid activity. The most immobile elements appear to be Si, Ti and Al (Putiš et al., Reference Putiš, Filová, Korikovsky, Kotov, Madarás, Grecula, Hovorka and Putiš1997a). The basement mylonites reflect deformational conditions of ~400–500°C at depths of 20–25 km (Putiš, Reference Putiš1994; Putiš et al., Reference Putiš, Filová, Korikovsky, Kotov, Madarás, Grecula, Hovorka and Putiš1997a, Reference Putiš, Unzog, Wallbrecher, Fritz, Grecula, Hovorka and Putiš1997b). The exhumation age of the granitoid rocks has been determined from the newly formed phengite at ~100−85 Ma (Maluski et al., Reference Maluski, Rajlich and Matte1993; Dallmeyer et al., Reference Dallmeyer, Neubauer and Putiš1993, Reference Dallmeyer, Neubauer, Handler, Fritz, Müller, Pana and Putiš1996; Putiš et al., Reference Putiš, Frank, Plašienka, Siman, Sulák and Biroň2009b). Post-collisional exhumation is constrained by zircon fission tracks with ages of 88 to 62 Ma from the Veporic tectonic units (Plašienka et al., Reference Plašienka, Broska, Kissová and Dunkl2007; Vojtko et al., Reference Vojtko, Králiková, Jeřábek, Schuster, Danišík, Fügenschuh, Minár and Madarás2016). The apatite fission track ages of 63–55 Ma and apatite (U–Th)/He ages of 62–31 Ma from the southern Veporic Unit (Vojtko et al., Reference Vojtko, Králiková, Jeřábek, Schuster, Danišík, Fügenschuh, Minár and Madarás2016) suggest the upper crustal level of the exhumation and cooling to temperatures below ca. 250°C (zircon fission tracks), 125°C (apatite fission tracks) or to ca. 70°C [apatite (U–Th)/He], respectively.
Analytical methods
The minerals were sampled from natural outcrops and investigated in polished thin sections of metagranites (15 rock samples). The petrography of the samples was investigated using a polarised optical microscope.
The composition of the minerals was determined by electron probe microanalysis (EPMA) in wavelength-dispersive spectrometry (WDS) mode and X-ray elemental mapping, using a JEOL JXA–8530 F field emission electron microprobe at the Earth Science Institute of the Slovak Academy of Sciences in Banská Bystrica, Slovakia. An accelerating voltage of 15 kV and a beam current of 40−20 nA was used with other relevant analytical conditions chosen according to the mineral type. The typical spot beam diameter varied from 2 to 8 μm; a more focused ≤1–3 μm beam was used for some Y–B–Be silicates, and occasionally for secondary and heterogeneous fluorapatite. The EPMA was calibrated using natural and synthetic standards (Supplementary table S1), and raw counts were converted to wt.% of oxides using a full ZAF matrix correction. Corrections of line interferences for Nd→Ce, Eu→Nd, Eu→Pr, Gd→Ho, Tm→Sm, Nd→Ce, Lu→Dy, Lu→Ho, U→Th, K→U and V→Ti followed Åmli and Griffin (Reference Åmli and Griffin1975). Element amounts of <0.01 wt.% are below the detection limit.
Monazite Th–U–total Pb age determination was performed using a CAMECA SX–100 microprobe at the Department of Electron Microanalysis at the State Geological Institute of Dionýz Štúr in Bratislava. Operating conditions consisted of 15 kV accelerating voltage, 180 nA beam current and a 3 μm beam diameter. The PAP matrix correction factors were used (Supplementary table S1). Analytical lines free of overlap were selected preferentially. Mutual interferences among the REE were corrected using empirical correction factors. The PbMα line is overlapped by LaLα, ThMζ1, ThMζ2 and YLγ2,3 lines. The weak interference by the LaLα line was ignored. The UMß line is overlapped by ThMζ, ThM3-N4 and ThM5-P3 lines. PbMα line overlaps were resolved with a curved exponential background. Monazite age determination included the measurement of 7–9 monazite age standards, as well as the application of correction dependencies derived from the measured compositions. Further details and description of the age determination procedure (MARC) can be found in Konečný et al. (Reference Konečný, Kusiak and Dunkley2018).
Raman analysis was performed with a Horiba Jobin-Yvon LabRAM-HR 800 spectrometer in the Earth Science Institute of the Slovak Academy of Sciences, Banská Bystrica, Slovakia. The spectrometer is equipped with a Czerny-Turner monochromator with a 600 gr/mm grating and a Peltier-cooled Synapse CCD detector (Horiba Jobin-Yvon), and is connected to an Olympus BX41 microscope with a long-working distance 100×0.8 objective. The lateral resolution of the system is <2 μm. Samples were irradiated with He–Ne (633 nm) and Nd:YAG (532 nm) lasers. The Rayleigh line and a Teflon standard were used for calibration. The spectra were collected in 10–25 acquisitions that lasted 600 s or 1000 s per spectral window and processed using LabSpec 5 (Horiba) and PeakFit1 programs (SeaSolve Software Inc.). The Raman spectra were also obtained using a Thermo Scientific DXR3xi Raman Imaging microscope at the Slovak National Museum – The Natural History Museum in Bratislava, Slovakia. Several lasers were used during the investigation, mainly a 532 nm doubled Nd: YVO4 DPSS excitation laser and a 633 nm He–Ne laser, 100 × objective, a 25 μm confocal pinhole and an EMCCD detector. Approximately 80 spectra were acquired from the mineral phases investigated at a laser power of 10−20 mW for between 0.5 and 2 s (20 scans for a cycle). The processing of spectra (including fitting by Voigt functions) was carried out using the Thermo Fisher Scientific OMNIC v. 9.11 software package.
Spectra were collected in the 100−1200 cm–1 and 100−4000/6000 cm–1 ranges and from approximately the same spots as the EPMA, but shifted slightly from the mark left by the electron beam. This confirmed the composition, and thus distinct Raman bands were obtained. For accurate interpretation of the spectra belonging to hingganite-(Y) [Y2□Be2Si2O8(OH)2], macroscopic samples of gadolinite-(Y) [Y2Fe2+Be2Si2O8O2] from the localities of Tinbo, Sweden and Hitterö, Norway from the collections at the Slovak Natural History Museum, Bratislava were also analysed by Raman spectroscopy.
The multi-element lithogeochemistry of powdered samples was performed by Bureau Veritas (AcmeLabs) in Vancouver, Canada, by X-ray fluorescence (XRF) for major elements, and inductively-coupled plasma atomic emission spectrometry (ICP–AES), together with inductively-coupled plasma mass spectrometry (ICP–MS) for the trace and rare earth elements.
Element contents in the mineral formulae are expressed in atoms per formula unit (apfu). The hellandite formulae (redefined by Oberti et al., Reference Oberti, Langone, Boiocchi, Bernabè and Hawthorne2019) were normalised on the basis of Si4+ + P5+ = 4 cations and the partition of total manganese between Mn2+ (Y site) and Mn3+ (Z site) was calculated from ideal stoichiometry and neutral charge balance. Gadolinite-group nomenclature and mineral formulae calculation (Bačík et al., Reference Bačík, Miyawaki, Atencio, Cámara and Fridrichová2017) were used. The hingganite-(Y) formulae were normalised on the basis of A = 2 cations, the OH– content was calculated as (2*M□) – F, where M□ is a vacancy at the M site. The B2O3 and BeO contents (wt.%) were calculated from the charge balance according to Be = 6 – [20 – ΣPC – OH–] (where PC is the sum of charges for all cations except B and Be) and B = 2 – Be. The formulae of epidote-group minerals were normalised on the basis of Σ(A + M + T) = 8 cations following the procedure of Armbruster et al. (Reference Armbruster, Bonazzi, Akasaka, Bermanec, Chopin, Gieré, Heuss-Assbichler, Liebscher, Menchetti, Pan and Pasero2006), the partition of total Fe between Fe2+ and Fe3+ was calculated from ideal stoichiometry and neutral charge balance, and the H2O content was calculated assuming OH– + F– + Cl– = 1 apfu. Fluorapatite was calculated on the basis of Σ(M + T) = 8 cations and OH– + F– + Cl– = 1 apfu (Pasero et al., Reference Pasero, Kampf, Ferraris, Pekov, Rakovan and White2010). Monazite-(Ce) and xenotime-(Y) formulae were normalised to 4 oxygen atoms.
Sample description and accessory mineral assemblage
We investigated 15 polished thin sections of mylonitised metagranitic rocks from the Fabova Hoľa Massif Vepor pluton, in the Slovak Ore Mountains (Veporic Unit). They usually contain accessory monazite-(Ce) with secondary reaction coronae of fluorapatite + allanite-(Ce) to epidote or clinozoisite. However, sample FAH-3 also contains accessory xenotime-(Y) with different, newly described fluorapatite + hellandite-(Y) + hingganite-(Y) reaction coronae. This sample is described in detail. FAH-3 was collected from a small, natural outcrop in the Hronec valley, ~500 m south from the Klátna gamekeeper's lodge on the western slope of Klátny Grúň hill, (48°47′41″N 19°56′26″E).
The rock studied (FAH-3) represents a foliated blastomylonitised porphyric metagranite. The texture is grano-lepidoblastic to lepidoblastic with blastomylonitic foliation. The primary magmatic minerals include quartz-1, plagioclase, K-feldspar, biotite-1 and muscovite-1, with accessory zircon, fluorapatite, monazite-(Ce), xenotime-(Y) and Fe–Ti oxides (ilmenite>magnetite). In addition, FAH-3 contains a newly-formed and recrystallised mineral assemblage, which includes quartz-2, albite, phengitic muscovite, epidote to clinozoisite, Ti-depleted biotite-2 and muscovite-2.
Quartz-1 forms anhedral crystals with distinct undulatory extinction and is rimmed by fine-grained aggregates of recrystallised quartz-2. Subhedral plagioclase (oligoclase with An20−15) is replaced extensively by fine-crystalline white mica aggregates (sericitisation) and irregular rim zones of secondary albite (~An01). White-to-grey K-feldspar (98 mol.% orthoclase/microcline, 2 mol.% albite, 1 mol.% celsian on average) forms large euhedral phenocrysts (up to 4 cm in size) locally, with Carlsbad twinning and inclusions of plagioclase and biotite-1. Biotite-1 has an annite-dominant composition [Fe/(Fe + Mg) = 0.55−0.57; TAl = 1.17−1.24 apfu] and forms subhedral, distinctly foliated crystals, locally baueritised to muscovite-2, or chloritised with tiny sagenitic inclusions of titanite and rutile. The existence of primary magmatic muscovite-1 is possible, however it was not clearly proved by optical or microprobe study. Muscovite-2 occurs as very fine-grained aggregates (‘sericite’) or subhedral to euhedral platy crystals (up to 0.3 mm in size), usually with phengitic (celadonite-rich muscovite) composition (3.1−3.3 apfu Si; Fe + Mg = 0.4−0.6 apfu). The representative assemblage of primary and secondary rock-forming minerals is shown in Fig. 2a.

Fig. 2. Back-scattered electron (BSE) images of a mylonitised metagranite and coronae around REE accessory phosphate minerals. (a) An assemblage of rock-forming minerals including quartz-1 (Qz 1), biotite-1 (Bt 1) to chlorite (Chl), feldspars (Fs), locally replaced by fine-crystalline white mica and clinozoisite (Czo). Accessory minerals are apatite-1 (Ap 1), zircon (Zrn) and monazite-(Ce) (Mnz). (b) Typical retrogade corona around monazite-(Ce) (Mnz) surrounded by polygonal apatite-2a (Ap 2a) + bright huttonite inclusions (Ht) and epitaxial intergrowths of allanite-(Ce) (Aln) to clinozoisite (Czo) in biotite-1 (Bt 1). (c) Lopsided corona with an asymmetric tail of allanite-(Ce)-clinozoisite (Aln-Czo) trailing outwards from the monazite-(Ce) (Mnz) in close association with apatite-1 (Ap 1). The other secondary minerals are apatite-2a (Ap 2a) and titanite (Ttn). The host rock-forming minerals are biotite-1 (Bt 1) to chlorite (Chl). (d) A tiny collar of allanite-(Ce) ± monazite-(Ce) relics outlining the euhedral habit of the former intergrown apatite-1 (Ap 1) and monazite-(Ce) (Mnz). The other secondary minerals are apatite-2a (Ap 2a) + Th-silicate huttonite (Ht) and clinozoisite (Czo). The host rock-forming mineral is biotite-1 (Bt 1). (e) Secondary corona with preserved xenotime-(Y) relic (Xtm) in the central part surrounded by an apatite-2b zone (Ap 2b). The external zone consists of hellandite-(Y) (Hld), hingganite-(Y) (Hin) and clinozoisite (Czo). The host rock-forming mineral is biotite-1 (Bt 1) with tiny inclusions of titanite (Ttn). (f) A similar corona with a central xenotime-(Y) relic (Xtm). A satellite chain-like aggregate of hellandite-(Y) (Hld) and hingganite-(Y) (Hin) is positioned between the apatite-2b zone (Ap 2b) and the distal zone of clinozoisite (Czo). The host rock-forming mineral is albite (Ab). (g) Close-up of the encircled area in (e) with hellandite-(Y) (Hld) and hingganite-(Y) (Hin) crystals in close vicinity to primary xenotime-(Y) (Xtm). The other secondary minerals are apatite-2b (Ap 2b), clinozoisite (Czo) and titanite (Ttn). (h) Close-up of the encircled area in (e) with hellandite-(Y) (Hld) and hingganite-(Y) (Hin) crystals in association with secondary apatite-2b (Ap 2b), clinozoisite (Czo) and rutile (Rt). IMA–CNMNC approved mineral symbols according to Warr (Reference Warr2021).
Primary fluorapatite (apatite-1) and zircon are the dominant accessory phases. Apatite-1 forms large, scattered euhedral-to-subhedral crystals up to 300 μm in size and associated with moderately chloritised biotite, quartz, albite and K-feldspar, together with accessory monazite-(Ce), xenotime-(Y) and their breakdown products. Zircon forms large euhedral prismatic crystals up to 300 μm in size (occasionally up to 500 μm), usually in association with apatite-1 and enclosed in biotite-1. Zircon has regular, magmatic oscillatory zoning, rare inherited xenocrystic cores and locally present inclusions of thorite and coffinite.
Representative whole-rock compositions are in Table 1. In general, the granites investigated have high SiO2 (67−75 wt.%), Al2O3 (14−17 wt.%) and Na2O + K2O – CaO (4−8 wt.%) values and low, but varying TiO2 (0.05−0.5 wt.%) and P2O5 (0.04−0.2 wt.%) values, together with moderate FeOtot/(FeOtot + MgO) (0.70−0.79). Their A/CNK and A/NK ratios range from 1.0 to 1.2 and 1.2 to 1.6, respectively, corresponding to a peraluminous composition. Trace-element geochemistry shows enrichment in Ba (390 to 1180 ppm) and Sr (230 to 400 ppm), as well as varying REE+Y (50 to 350 ppm) and Y (8 to 20 ppm). The concentration of light elements also shows enrichment, especially in B (~15 to 20 ppm; Marsina et al., Reference Marsina, Bodiš, Havrila, Janák, Káčer, Kohút, Lexa, Rapant and Vozárová1999) and Be (2 to 4 ppm). In general, they show an S-type or hybrid S/I-type affinity and are characterised by calc-alkaline compositions and low contents of Mg, Ca, Sr, Ba, Zr and REE when compared with I-type granites (cf. Broska and Uher, Reference Broska and Uher2001; Broska et al., Reference Broska, Williams, Uher, Konečný and Leichmann2004).
Table 1. Whole-rock compositions of the Fabova Hoľa metagranite (sample FAH-3).

* Boron content from Marsina et al. (Reference Marsina, Bodiš, Havrila, Janák, Káčer, Kohút, Lexa, Rapant and Vozárová1999).
Minerals of the reaction coronae
Fluorapatite–allanite-(Ce)–clinozoisite corona around monazite-(Ce)
The primary magmatic monazite-(Ce) is a relatively abundant accessory mineral of the FAH-3 metagranite sample. It is distributed equally throughout the host metagranite, and it is usually enclosed in chloritised biotite-1, or albite, with the association of zircon and apatite-1; close overgrowths and intergrowths of primary monazite-(Ce) with apatite-1 are common. The monazite is mostly preserved as partly-dissolved relics up to 200 μm in the centre of the coronae mineral aggregates. The monazite coronae aggregates attain ~100 to 250 μm in size and have well-developed concentric mineral zoning (Fig. 2b−d). The inner corona zone consists of polygonal, secondary fluorapatite (apatite-2a) with minute prismatic ThSiO4 inclusions (≤1 μm), which are most probably huttonite rather than dimorphous thorite. This is concluded from their high Th, Si, and LREE>HREE EDS signals and several WDS analyses of the average composition. More precise WDS analyses of these ThSiO4 inclusions are not possible due to analytical limitations. The outer corona zone comprises acicular to prismatic aggregates of secondary allanite-(Ce) to REE-rich epidote or clinozoisite with diffuse boundaries and radial intergrowth with the host-rock matrix (Fig. 2b−d). The corona has mostly a symmetrical shape (Fig. 2b), consisting sometimes of a lopsided corona, or an asymmetrical coronal tail of allanite-(Ce)–clinozoisite outwards from the monazite-(Ce) as seen in Fig. 2c, or a tiny collar of allanite-(Ce) ± monazite-(Ce) relics that defines the euhedral habit of the former intergrowths of apatite-1 and monazite-(Ce) (Fig. 2d). In some places, apatite-2–allanite-(Ce)–clinozoisite coronae without a monazite-(Ce) core occur, which either represent a different orientation of the non-centred cut in the corona structure or indicate a complete replacement of the monazite-(Ce).
The preserved monazite-(Ce) relics have a relatively homogeneous composition and contain only minor amounts of the cheralite (Ca0.5Th0.5PO4) and huttonite (ThSiO4) components (X chrl ≤10, occasionally ≤20 mol.%; X hutt ≤5 mol.%, Table 2). The chondrite-normalised REE distribution patterns shows a typical LREE>HREE slope (LaN/YbN = 77) and a distinct Eu negative anomaly (Fig. 3). The relatively high actinide content permits in situ Th–U–total Pb EPMA age determination of primary magmatic monazite-(Ce), which gave a Carboniferous (Mississippian, Tournaisian) age of 355 ± 1.9 Ma (n = 200, MSWD: 1.68; Fig. 4).

Fig. 3. Average chondrite normalised REE distribution patterns of REE-bearing minerals. Normalised values from Barrat et al. (Reference Barrat, Zanda, Moynier, Bollinger, Liorzou and Bayon2012). Note: TbN in the Y–B–Be silicates is interpolated between GdN and DyN due to a probable EPMA artefact. Yttrium is plotted in the position of Ho.

Fig. 4. Th–U–total Pb EPMA age determination. (a) Histogram and (b) isochron diagram of monazite ages from the Fabova hoľa granitoids. Pb values are in wt.%, Th* = Th + 3.15*U wt.%.
Table 2. Representative EPMA compositions of monazite-(Ce), xenotime-(Y), fluorapatite and epidote-group minerals (in wt.% and apfu). Abbreviations are as follows: monazite-(Ce) (Mnz), xenotime-(Y) (Xtm), fluorapatite (Ap), allanite-(Ce) (Aln), epidote (Ep), clinozoisite (Czo).

*H2O contents calculated on the basis of ideal stoichiometry. n.a. = not analysed, - = not calculated. 0.00 wt.% values indicate below the detection limit.
The composition of apatite-2a is relatively uniform and represents an almost pure Ca5(PO4)3F end-member (Table 2). The total REE content is generally low and never exceeds 3.5 wt.% (REE,Y)2O3 (0.1 apfu REE+Y), thus indicating only a subordinate presence of the fluorbritholite [(REE,Ca)5(SiO4)3F] component. Among REE, it has LREE>HREE+Y (av. LREE2O3 = 1.84 wt.% vs. av. HREE,Y2O3 = 0.44 wt.%). The concentrations of actinides (especially Th) rarely reach up to 5.5 wt.% ThO2 + UO2 (0.11 apfu Th and U), and together with elevated Si content (2.24 wt.% SiO2, 0.2 apfu Si) suggest the presence of Th-silicate nanoinclusions.
The secondary epidote-group minerals have a large variation in REE + Y + Th (0.02–1.00 apfu) and total Al values (1.57–2.47 apfu), thus indicating that varying amounts of allanite–ferriallanite and clinozoisite–epidote solid-solution components are present (Table 2). In contrast, clinozoisite has Fe3+ enrichment in the M3 position and a lower Al content, which indicates a significant epidote component. In general, the composition of the epidote-group minerals solid solution is controlled by a dominant A 2REE + M 3Fe2+ ↔ A 2Ca + M 3(Fe, Al)3+ substitution mechanism.
Fluorapatite–hellandite-(Y)–hingganite-(Y) coronae around xenotime-(Y)
Primary xenotime-(Y) is a relatively scarce, accessory mineral, but, when present, it always occurs as solitary crystals that are >100 μm in size in albite or biotite and surrounded by a secondary reaction corona. The reaction coronae around the primary xenotime-(Y) has an inner zone of typically highly-porous secondary fluorapatite (apatite-2b), which is analogous to the monazite coronae, though differs distinctly in the REE minerals of the outer zone. This novel type of secondary reaction micro-texture consists of Y–B–Be silicate minerals instead of the expected allanite-(Y) and/or HREE+Y-rich epidote. These Y–B–Be phases are represented by hellandite-(Y) [(Ca,REE)4Y2Al□2(B4Si4O22)(OH)2] and hingganite-(Y) [Y2□Be2Si2O8(OH)2]. Both minerals occur as numerous tiny anhedral to subhedral satellite crystals and/or chain-like aggregates and intergrowths (usually ~1–5 μm, occasionally ≤10 μm in size) positioned between the apatite-2 and the clinozoisite zone (Fig. 2e−h). The presence of these minerals is also confirmed by X-ray compositional mapping, including B (Fig. 5) and Raman spectra (see section on Raman spectroscopy). A visible disproportion between the massive apatite-2b zone and the thin hellandite–(Y)-hingganite-(Y) zone is a typical feature and contrasts with the conspicuous development of the REE-bearing zone around monazite-(Ce).

Fig. 5. False colour X-ray map showing the distribution of elements in the reaction corona around xenotime-(Y).
Xenotime-(Y) has a distinct dominance of Y over other HREE components and the chondrite-normalised REE distribution pattern shows a HREE>LREE slope (LaN/YbN = 0.001) (Fig. 3). The relatively low, though homogeneous, distribution of B is confirmed by X-ray compositional mapping (Fig. 5). This systematic presence of B together with a slightly increased signal of Nb + Ta in xenotime-(Y), indicates the possible, but very limited schiavinatoite (NbBO4) + béhierite (TaBO4) substitution mechanism: (Nb,Ta)BY–1P–1.
The apatite-2b generation differs slightly from apatite-2a in REE and Y concentrations, where apatite-2b has a higher HREE2O3 and an especially higher Y2O3 content (average value 0.80 wt.%; 0.03 apfu HREE + Y), although the LREE content is below the EPMA detection limit. In contrast, the measured concentrations of Th and U in apatite-2b are lower than apatite-2a, with ≤0.5 wt.% (Th,U)O2 (≤0.01 apfu Th and U) (Table 2).
The hellandite-(Y) compositions (Table 3) show a predominance of Y (22.9–27.8 wt.% Y2O3; 2.2–2.4 apfu Y) over other REE (⩽15.9 wt.% REE2O3; 0.92 apfu REE) and have an atomic ratio of Y/(Y + REE) = 0.70–0.81. Among the REE, hellandite-(Y) has HREE>LREE, which is also visible in the chondrite-normalised REE pattern, in accordance with its Y dominance (Fig. 3). This HREE>>LREE slope (average LaN/YbN = 0.004) is observed typically in other Y-dominant REE minerals and is analogous to the primary xenotime-(Y). Aluminium dominates strongly over Fe3+ among the Z site cations (4.1–5.3 wt.% Al2O3; 0.77–1.0 apfu Al and 0.0–1.4 wt.% Fe2O3; 0.0–0.20 apfu Fe respectively) with an atomic ratio of Al/(Al + Fe) = 0.84–1.00. Calcium is the dominant cation at the X site, ranging from 14.6 to 16.7 wt.% CaO (2.8–2.9 apfu Ca). However, all analytical results include a small Ca deficiency compared to the ideal formula, and this imbalance is compensated by an appropriate amount of REE. As a consequence, hellandite-(Y) compositions are close to the end-member formula with a small Fe3+Al–1 substitution. Other possible exchange vectors are negligible (Fig. 6).
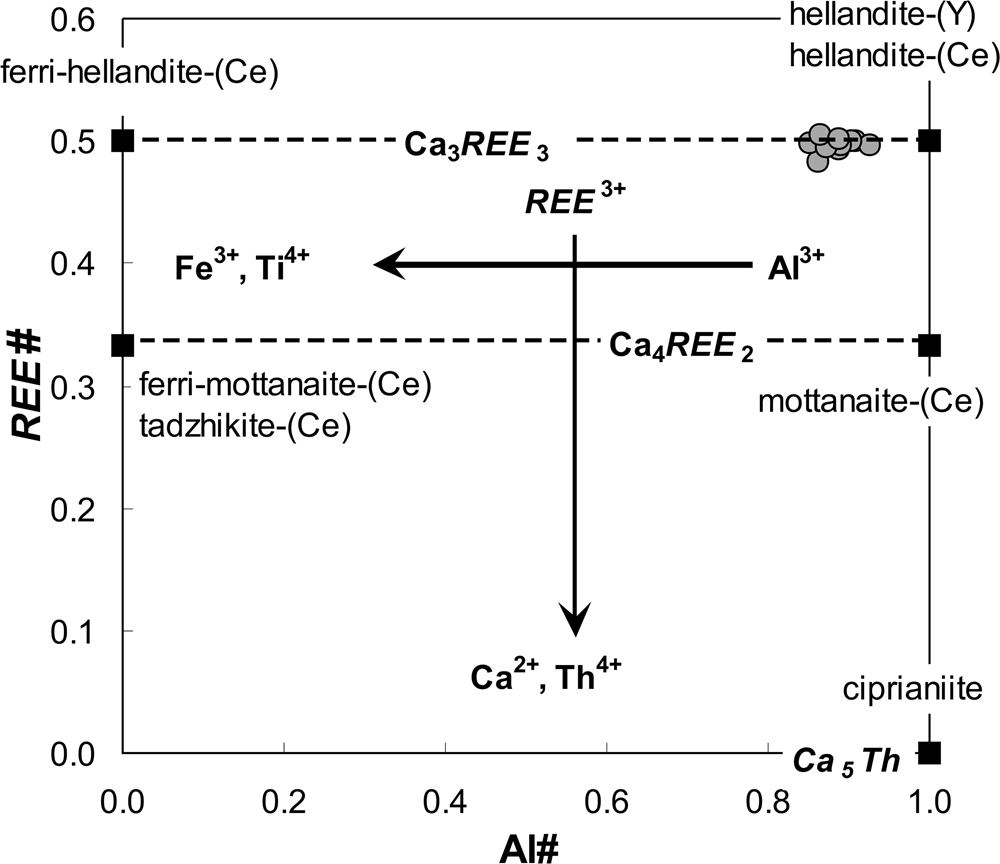
Fig. 6. Compositional diagram of the hellandite-group minerals with the principal substitution vectors and positions of the hellandite-(Y) from the Fabova Hoľa metagranite. Al# = Al/((Al + Fe3+ + Mn3+ + Ti) apfu; REE# = REE/(REE + Ca + Mn2+ + Th + U) apfu; REE = ∑(La + Ce + … + Lu + Y) apfu.
Table 3. Representative compositions from EPMA of hellandite-(Y) (in wt.% and apfu).

*B2O3 content calculated on the basis of ideal stoichiometry ** The partition of total Mn between Mn2+ and Mn3+ was calculated from ideal stoichiometry and neutral charge balance. ***H2O content calculated from charge balance. - = not analysed/calculated. 0.00 wt.% values indicate below the detection limit.
The hingganite-(Y) composition (Table 4) shows a dominance of Y (26.7–33.4 wt.% Y2O3; 1.0–1.3 apfu Y) over other REE, mainly HREE (10.2–15.1 wt.% REE2O3; 0.23–0.38 apfu REE) on the A site. The atomic ratio of Y/(Y + REE) for hingganite-(Y) (0.77–0.82) is similar, though in a narrower range compared to hellandite-(Y). In addition, the chondrite-normalised REE pattern shows an identical trend with the enrichment of HREE (slope (LaN/YbN = 0.006) (Fig. 3). Additionally, Ca is the other significant cation at the A site (4.5–10.6 wt.% CaO; 0.36–0.79 apfu Ca), which indicates the significant presence of datolite [Ca2□B2Si2O8(OH)2] and homilite (Ca2Fe2+B2Si2O8O2) molecules (Fig. 7). Hingganite-(Y) has a dominant vacancy (0.49–0.65 pfu) over the M site cations, especially the Fe content, which is markedly low (5.5–7.8 wt.% FeO; 0.34–0.50 apfu Fe) with a Fe/(Fe + vacancy) atomic ratio of 0.34–0.51. Conversely, hingganite-(Y) has a negligible and usually zero Al content at the M site, thereby indicating a strong Al and Fe distinction between hellandite-(Y) and hingganite-(Y). Whereas hellandite-(Y) has an almost constant B content (cf. Oberti et al., Reference Oberti, Della Ventura, Ottolini, Hawthorne and Bonazzi2002; Miyawaki et al., Reference Miyawaki, Momma, Yokoyama, Shigeoka, Matsuraba, Ito, Nakai and Kristiansen2015), the B concentration in hingganite-(Y) from the Fabova Hoľa metagranite is controlled by the datolite CaB(REE)–1Be–1 substitution with B/(B + Be)calc = 0.0–0.38. It has an intermediate solid solution between the Be-dominant [hingganite-(Y)–gadolinite-(Y)], and B-dominant (homilite, datolite) end-members of the gadolinite-group minerals.

Fig. 7. Classification diagram of the gadolinite-group minerals from Bačík et al. (Reference Bačík, Miyawaki, Atencio, Cámara and Fridrichová2017) with the positions of hingganite-(Y) from the Fabova Hoľa metagranite.
Table 4. Representative compositions from EPMA of hingganite-(Y) from the gadolinite group (in wt.% and apfu).

*B2O3 and BeO contents calculated from charge balance, **H2O content calculated on the basis of ideal stoichiometry. - = not analysed/calculated. 0.00 wt.% values indicate below the detection limit.
Raman spectra of hellandite-(Y) and hingganite-(Y)
The presence of B- and Be-bearing silicate phases in the breakdown products was determined from their Raman spectra. The Raman spectra of the hingganite-(Y) can be divided into several regions with relatively intensive bands and these vary due to different crystal orientations (Fig. 8a). In the region between 100 and 400 cm–1, the most prominent peaks are at 189 cm–1 (with a peak on the shoulder at 153 cm–1) and 324 cm–1. The region of 400 to 800 cm–1 includes several overlapping, less-intensive bands with two prominent peaks at 453 and 639 cm–1. The third most intensive peak, together with 189 and 639 cm–1, is located at 917 cm–1 with two shoulder peaks at 969 and 998 cm–1. In the high-frequency region, there is a sharp peak at 3546 cm–1 located on the broad band spreading between 3100 and 4000 cm–1.

Fig. 8. (a) Raman spectra of hingganite-(Y) excited by a 532 nm laser; two different orientations of the crystal (A and B). (b, c, d) Raman spectra of hellandite-(Y) excited by a 532 nm laser for two different crystals and spectrum of hellandite-(Y) excited by a 633 nm laser (dashed line).
All μ-Raman depolarised spectra of randomly oriented hellandite-(Y) crystals (FAH-3) correspond to their minor compositional variations. In the low-frequency region (200−1000 cm–1), the spectra show numerous peaks superimposed on an increased background luminescence. The most dominant features are in the regions of 250−370, 500−550, and 560−750 cm–1 (Fig. 8b−d), in addition to the complexes of numerous, less-intensive bands. Two relatively sharp peaks at 3454 and 3662 cm–1 located on top of the broad band are present in the high-frequency region. (Fig. 8d).
Discussion and conclusions
Raman spectra interpretation
Raman spectra of the investigated hingganite-(Y) were interpreted in context with published data, including that of isostructural datolite and gadolinite (Frost et al., Reference Frost, Xi, Scholz, Lima, Horta and Lopez2013; Goryainov et al., Reference Goryainov, Krylov, Vtyurin and Pan2015; Thomas and Davidson, Reference Thomas and Davidson2017; Škoda et al., Reference Škoda, Plášil, Čopjaková, Novák, Jonsson, Vašinová Galiová and Holtstam2018; Paulmann et al., Reference Paulmann, Zietlow, McCammon, Salje and Bismayer2019; Gorelova et al., Reference Gorelova, Vereshchagin, Cuchet, Shilovskikh and Pankin2020; Tomašić et al., Reference Tomašić, Škoda, Bermanec and Šoufek2020) and were also compared with spectra of macroscopic gadolinite samples. Škoda et al. (Reference Škoda, Plášil, Čopjaková, Novák, Jonsson, Vašinová Galiová and Holtstam2018) assigned bands in the region of 200−750 cm–1 to bending modes of Si–O and Be–O, stretching vibrations of REE–O and Fe–O, as well as to lattice vibrations. According to Hofmeister et al. (Reference Hofmeister, Hoering and Virgo1987), modes below 350 cm–1 in silicates are generally considered to be translations. The Raman band at 3546 cm–1 is attributed to stretching vibrations of OH– units in the mineral structure (Fig. 8a).
Part of the bands in the region from 450 cm–1 to 650 cm–1 could be assigned to bending vibrations of the layer formed by (SiO4)4– and (BeO4)6– tetrahedra and were also detected in the spectra of macroscopic samples (Gorelova et al., Reference Gorelova, Vereshchagin, Cuchet, Shilovskikh and Pankin2020). Raman peaks at 969 cm–1 and 917 cm–1 (in the range of 969−992 and 906−917 cm–1 in the fitted spectra) are assigned to stretching vibrations of Si–O and Be–O in tetrahedral coordination (Škoda et al., Reference Škoda, Plášil, Čopjaková, Novák, Jonsson, Vašinová Galiová and Holtstam2018; Gorelova et al., Reference Gorelova, Vereshchagin, Cuchet, Shilovskikh and Pankin2020). The presence of a peak at 917 cm–1 correlates well with the fitted spectra of the macroscopic gadolinite samples. Weak Raman bands in the range of 650−800 cm–1 correspond to Be–O stretching vibrations (Hofmeister et al., Reference Hofmeister, Hoering and Virgo1987).
However, not all the bands can easily be attributed to known causes, according to the published data (Leite et al., Reference Leite, Guimarães, Fernandes, Continentino, Paschoal, Ayala and Guedes2001; Škoda et al., Reference Škoda, Plášil, Čopjaková, Novák, Jonsson, Vašinová Galiová and Holtstam2018; Gorelova et al., Reference Gorelova, Vereshchagin, Cuchet, Shilovskikh and Pankin2020). The Raman spectra of all the measured mineral phases, compositionally corresponding to hingganite-(Y), are essentially obscured by strong photoluminescence, as both broad and narrow bands. Even a minor lanthanoid content in the structure may cause intense luminescence (Panczer et al., Reference Panczer, De Ligny, Mendoza, Gaft, Seydoux-Guillaume, Wang, Dubessy, Caumon and Rul2012; Lenz et al., Reference Lenz, Nasdala, Talla, Hauzenberger, Seitz and Kolitsch2015; Gaft et al., Reference Gaft, Reisfeld and Panczer2005; de Bettencourt-Dias, Reference de Bettencourt-Dias2014). Taking into consideration the concentrations of Gd, Dy, Eu, Yb, Lu and Tm (Table 4), there is a strong indication that some of features in the spectra are in fact photoluminescence bands. Some of these bands can be present in the range of 453–562 cm–1 in the 532 nm spectrum of hingganite-(Y). If recalculated to wavelength, 540–550 nm bands could correspond to luminescence bands that were caused by the 4S3/2–4I15/2 electronic transition in Er3+ (Bodył et al., Reference Bodył, Czaja and Mazurak2009; de Bettencourt-Dias, Reference de Bettencourt-Dias2014).
The presence of 3546 cm–1 correlates well with the hingganite-(Y) composition, which contains the OH– group located at the φ5 site, in contrast to gadolinite, which contains O at φ5 (Bačík et al., Reference Bačík, Miyawaki, Atencio, Cámara and Fridrichová2017). However, the peak is located on the top of the strong broad band (Fig. 8a), which was recalculated, considering that the 532 nm excitation has a centre at ca. 660 nm and therefore, can be interpreted as a luminescence band induced by the 4F9/2→6H15/2–9/2 electronic transition in Dy3+ (de Bettencourt-Dias, Reference de Bettencourt-Dias2014).
The stacked spectra of hellandite-(Y) measured with 532 nm and 633 nm excitation show several overlapping peaks that might be attributed to Raman scattering. The shape and band intensities of individual spectra vary due to different crystal orientation. The position of the broad bands partly matches the spectrum of hellandite-(Ce) (RRUFF ID: R061013, Lafuente et al., Reference Lafuente, Downs, Yang, Stone, Armbruster and Danisi2015), where the tetrahedrally coordinated T site is vacant. The high number and broadening of the bands results from a varying composition. In the Raman spectra, as in the gadolinite-group minerals, stretching vibrations of REE–O and Fe–O and lattice vibrations are observed in the spectral region 200–750 cm–1 (Leite et al., Reference Leite, Guimarães, Fernandes, Continentino, Paschoal, Ayala and Guedes2001; Škoda et al., Reference Škoda, Plášil, Čopjaková, Novák, Jonsson, Vašinová Galiová and Holtstam2018). The vibrations in the 320–600 cm–1 region may be attributed to out-of-plane and in-plane bending vibrations of the (SiO4)4– tetrahedron. Hofmeister et al. (Reference Hofmeister, Hoering and Virgo1987) assigned the wavenumbers of the Si–O and Be–O vibrations in phenakite (Be2SiO4), which is a mineral with (SiO4)4– and (BeO4)6– tetrahedra and roughly analogous to the layers of tetrahedra in hellandite. The observed bands above 875 cm–1 thus correspond to Si–O antisymmetric stretching vibrations. Raman bands at 780–810 cm–1 and 768–778 cm–1 may possibly relate to Si–O and Be–O stretching vibrations, respectively. The band intensity at 662 cm–1 (in 633 nm excited spectrum) can be influenced by photoluminescence of Dy3+, which occurs in some minerals at ~660 nm (Gaft et al., Reference Gaft, Reisfeld and Panczer2005; de Bettencourt-Dias, Reference de Bettencourt-Dias2014). This luminescence band results from the 4F9/2→6H15/2–9/2 electronic transition in Dy3+, similarly as in hingganite-(Y) (de Bettencourt-Dias, Reference de Bettencourt-Dias2014). In the range of 2500–4000 cm–1 spectra possess continuum background topology.
The strong broad band below the two intensive lines at 3454 cm–1 and 3662 cm–1 was only detected with 532 nm excitation. The relatively sharp bands could be attributed to OH– vibrations. Della Ventura et al. (Reference Della Ventura, Bonazzi, Oberti and Ottolini2002) described spectral bands in this region as an expression of a wide variety of OH– environments due to local short-range disorder at the next-nearest-neighbour cation sites bonded to O5. However, the broad band with a position similar to the 532 nm spectrum of hingganite-(Y) can also result in the 4F9/2→6H15/2–9/2 electronic transition in Dy3+ (de Bettencourt-Dias, Reference de Bettencourt-Dias2014).
On the basis of our interpretations, Er and Dy are the main sources of photoluminescence features in the spectra of both hingganite-(Y) and hellandite-(Y). This correlates perfectly with the chemical data. Erbium and Dy are the most abundant REE together with Gd and Yb. However, the most prominent photoluminescence band of Gd3+ (6F7/2→8S7/2) is in the UV region around 315 nm, and the most emissive 2F5/2→2H7/2 electronic transition of Yb3+ manifests itself in the NIR region around 980 nm (de Bettencourt-Dias Reference de Bettencourt-Dias2014). The ranges of measurement were 535 to 675 nm and 640 to 855 nm for the 532 and 633 nm excitations, respectively. The content of other REE with possible photoluminescence bands in the measured region (Pr, Sm, Eu, Tb, Ho and Tm; de Bettencourt-Dias, Reference de Bettencourt-Dias2014) are lower, usually below 0.5 oxide wt.%. Therefore, these REE may contribute to the dominant photoluminescence features, or to an increase in the background, however they do not manifest in a resolvable photoluminescence band.
Crystal chemistry of contrasting coronae formation and breakdown reactions
The metamorphic-hydrothermal breakdown of monazite-(Ce) to the secondary fluorapatite–allanite-(Ce)–clinozoisite corona is a widely reported process in granitic, gneissic and metapelitic lithologies during post-magmatic cooling, or low- to high-grade prograde/retrograde metamorphic conditions (Broska and Siman, Reference Broska and Siman1998; Finger et al., Reference Finger, Broska, Roberts and Schermaier1998, Reference Finger, Krenn, Schulz, Harlov and Schiller2016; Broska et al., Reference Broska, Williams, Janák and Nagy2005; Majka and Budzyń, Reference Majka and Budzyń2006; Petrík and Konečný, Reference Petrík and Konečný2009; Ondrejka et al., Reference Ondrejka, Uher, Putiš, Broska, Bačík, Konečný and Schmiedt2012, Reference Ondrejka, Putiš, Uher, Schmiedt, Pukančík and Konečný2016; Regis et al., Reference Regis, Cenki-Tok, Darling and Engi2012; Upadhay and Pruseth, Reference Upadhyay and Pruseth2012; Lo Pò et al., Reference Pò D., Braga, Massonne, Molli, Montanini and Theye2016; Hentschel et al., Reference Hentschel, Janots, Trepmann, Magnin and Lanari2020). It has also been experimentally replicated (Budzyń et al., Reference Budzyń, Harlov, Williams and Jercinovic2011, Reference Budzyń, Harlov, Kozub-Budzyń and Majka2017). The analogous breakdown of xenotime-(Y) is not as common as monazite, but, when present, it usually results in similar corona structures, with an inner zone of fluorapatite (commonly HREE+Y-rich) and/ or an outer zone of HREE-bearing epidote-group minerals, albeit in much smaller amounts (Broska et al., Reference Broska, Williams, Janák and Nagy2005; Majka and Budzyń, Reference Majka and Budzyń2006; Janots et al., Reference Janots, Engi, Berger, Allaz, Schwartz and Spandler2008; Broska and Petrík, Reference Broska and Petrík2015; Budzyń et al., Reference Budzyń, Sláma, Kozub-Budzyń, Konečný, Holický, Rzepa and Jastrzębski2018; Hentschel et al., Reference Hentschel, Janots, Trepmann, Magnin and Lanari2020). Experiments involving xenotime-(Y) replacement indicate that the reaction products are roughly similar to those found in natural occurrences over a wide P–T range, and reflect the relatively high mobilisation of HREE+Y into the apatite-supergroup minerals (fluorapatite, fluorcalciobritholite) or epidote, which depends on the CaO/Na2O ratio (Budzyń and Kozub-Budzyń, Reference Budzyń and Kozub-Budzyń2015; Budzyń et al., Reference Budzyń, Harlov, Kozub-Budzyń and Majka2017). These experiments also suggest that the reactivity of xenotime-(Y) is temperature-dependent, and that the stability of xenotime-(Y) and HREE+Y-rich epidote is strongly controlled by pressure (Budzyń et al., Reference Budzyń, Harlov, Kozub-Budzyń and Majka2017). These results suggest that xenotime-(Y) is more stable than monazite-(Ce) due to a lesser mobility of HREE+Y compared with LREE during low- to medium-grade metamorphism.
The effects of retrograde metamorphism during, and after, deformation on primary magmatic monazite-(Ce) and xenotime-(Y) are distinctive in the Fabova Hoľa metagranites. Similar corona reaction zones around monazite-(Ce) and xenotime-(Y) usually suggest that they were most probably formed contemporaneously, and that the mechanism of their formation was similar (cf. Broska et al., Reference Broska, Williams, Janák and Nagy2005; Majka et al., Reference Majka, Pršek, Budzyń, Bačík, Barker and Łodziński2011). In some cases, the fluorapatite–hingganite-(Y) coronae, as a product of fluid-induced breakdown of xenotime-(Y) and beryl, have been described from the Skoddefjellet pegmatite, Svalbard (Majka et al., Reference Majka, Pršek, Budzyń, Bačík, Barker and Łodziński2011), whereas the partial replacement of xenotime-(Y) by hingganite-(Y) and Y-rich allanite-(Ce) was documented in the Piława Górna pegmatite, Poland (Budzyń et al., Reference Budzyń, Sláma, Kozub-Budzyń, Konečný, Holický, Rzepa and Jastrzębski2018). Alteration of primary monazite-(Ce) and xenotime-(Y) resulted in the formation of discrete grains of secondary hingganite-(Y) to hingganite-(Nd) in association with secondary Sr,S-rich monazite-(Ce) in the Bacúch metamorphic magnetite deposit, Veporic Unit, Slovakia (Pršek et al., Reference Pršek, Ondrejka, Bačík, Budzyń and Uher2010), only ~13 km NW from the FAH-3 metagranite sample. All of these examples indicate a substantial supply of Be during the xenotime (± monazite) breakdown.
However, in our example, the REE remobilisation during alteration of the primary monazite-(Ce) and xenotime-(Y) resulted in a slightly different secondary assemblage. Whereas LREE released from the altered monazite-(Ce) were transported through the apatite-2a zone and accumulated in an allanite-(Ce) zone, the HREE and Y from xenotime-(Y) were transported the same distance, though precipitated as hellandite-(Y) and hingganite-(Y) (Fig. 9). The reported corona assemblage, which includes Y–B–Be silicates and most notably hellandite-(Y), represents a new type of breakdown micro-texture with the involvement of light elements (B and Be) in the reaction, which have not been reported previously. The metamorphic/metasomatic replacement during blastomylonitisation including fluids rich in B and Be can be expressed by the following generalised reactions:
Mnz + (Ca, Fe, Si, Al and F)-rich fluid → FAp + Ht + Aln + Czo;
Xtm + (Ca, Fe, Si, Al, F, B and Be)-rich fluid → FAp + Hld + Hin + Czo.

Fig. 9. Summary sketch of the main stages of monazite/ xenotime metasomatic alteration transformation.
Hellandite-(Y), which is a relatively rare borosilicate mineral, occurs mostly as a late-magmatic to hydrothermal mineral of NYF (Nb–Y–F) granitic pegmatites (Hogarth et al., Reference Hogarth, Chao and Harris1972; Mellini and Merlino, Reference Mellini and Merlino1977; Ma et al., Reference Ma, Zhang and Yang1986; Miyawaki et al., Reference Miyawaki, Nakai, Nagashima, Okamoto and Isobe1987, Reference Miyawaki, Momma, Yokoyama, Shigeoka, Matsuraba, Ito, Nakai and Kristiansen2015; Pieczka et al., Reference Pieczka, Hawthorne, Cooper, Szełęg, Szuszkiewicz, Turniak, Nejbert and Ilnicki2015) and in the Predazzo granite, Italy (Emiliani and Gandolfi, Reference Emiliani and Gandolfi1965; Mellini and Merlino, Reference Mellini and Merlino1977). In contrast, its occurrence, which has been documented in a late greenschist-facies regional metamorphic assemblage in the form of micro-veins transecting chloritite rock from the Abitibi greenstone belt, Ontario and Quebec, Canada, indicates that Y and B were mobilised during the low-grade metamorphism (Pan et al., Reference Pan, Fleet and Barnett1994). Other members of hellandite-group minerals [general chemical formula: X 4Y 2ZT 2(B4Si4O22)W 2; Oberti et al. Reference Oberti, Langone, Boiocchi, Bernabè and Hawthorne2019] show a strong dominance of Ce over Y and LREE>HREE. They have also been described in alkaline rocks, mainly in miarolitic cavities and vugs of syenitic ejecta in volcanic rocks from Latium, Italy [hellandite-(Ce), mottanaite-(Ce) Ca4Ce2Al(Be1.5□0.5) (B4Si4O22)O2, ferri-mottanaite-(Ce) Ca4Ce2Fe3+(Be1.5□0.5)(B4Si4O22)O2, ciprianiite Ca4(ThCa)Al(Be1.5□0.5)(B4Si4O22)O2; Della Ventura et al., Reference Della Ventura, Williams, Cabella, Oberti, Caprilli and Bellatreccia1999, Reference Della Ventura, Bonazzi, Oberti and Ottolini2002; Oberti et al., Reference Oberti, Ottolini, Cámara and Della Ventura1999, Reference Oberti, Langone, Boiocchi, Bernabè and Hawthorne2019; Perna et al., Reference Perna, Zaccaria, Rosatelli, Stoppani, Curti, Spratt, Humphreys-Williams, Najorka, Brownscombe, Nestola and Stoppa2021] or in metasomatic rocks associated with alkaline magmatic rocks from the Dara-i-Pioz complex, Tajikistan, and the Hodza-Achkan massif, Kyrgyzstan [tadzhikite-(Ce) Ca4Ce2Ti□2(B4Si4O22)O2; Reguir et al., Reference Reguir, Chakhmouradian and Evdokimov1999; Pautov et al., Reference Pautov, Karpenko and Agakhanov2013]. Mottanaite-(Ce), ferri-mottanaite-(Ce), ciprianiite and some hellandite-(Ce) also contain a notable concentration of Be (1.8–3.0 wt.% BeO; 0.7–1.2 apfu; Della Ventura et al., Reference Della Ventura, Bonazzi, Oberti and Ottolini2002; Oberti et al., Reference Oberti, Langone, Boiocchi, Bernabè and Hawthorne2019; Perna et al., Reference Perna, Zaccaria, Rosatelli, Stoppani, Curti, Spratt, Humphreys-Williams, Najorka, Brownscombe, Nestola and Stoppa2021), which occupies a special tetrahedral T site, usually vacant in hellandite-(Y), hellandite-(Ce) and tadzhikite-(Ce) (e.g. Oberti et al., Reference Oberti, Della Ventura, Ottolini, Hawthorne and Bonazzi2002, Reference Oberti, Langone, Boiocchi, Bernabè and Hawthorne2019).
The crucial factors in controlling the competitive crystallisation of hellandite-(Y) or hingganite-(Y) are most probably the local Al–Fe redistribution and the Fe2+/Fe3+ ratio; where hellandite-(Y) contains a significant Al content and only minor amounts of Fe3+ (cf. Pan et al., Reference Pan, Fleet and Barnett1994), whereas the gadolinite–hingganite series is Al-free and favours Fe2+ instead of Fe3+ (Tables 3 and 4) (cf. Miyawaki et al., Reference Miyawaki, Nakai and Nagashima1984; Demartin et al., Reference Demartin, Pilati, Diella, Gentile and Gramaccioli1993, Reference Demartin, Minaglia and Gramaccioli2001b; Cámara et al., Reference Cámara, Oberti, Ottolini, Della Ventura and Bellatreccia2008; Bačík et al., Reference Bačík, Fridrichová, Uher, Pršek and Ondrejka2014). Extremely rare ferri-mottanaite-(Ce), the first Fe3+-dominant hellandite group mineral from the Vico volcanic province, Italy (Oberti et al., Reference Oberti, Langone, Boiocchi, Bernabè and Hawthorne2019), and ferri-hellandite-(Ce) [(Ca3Ce)Ce2Fe3+□2Si4B4O22(OH)2] from the Sagåsen larvikite quarry, Mørje, Porsgrunn, Vestfold and Telemark, Norway (Friis et al., Reference Friis, Škoda, Larsen, Vašinová-Galiová, Čtvrtlík and Filip2021), were described only recently. Nevertheless, a second important factor could prevent the crystallisation of Fe3+-rich hellandite-group minerals; namely the local Be:B ratio. Amounts of Be could rise to give a ratio of 1.5:4 in the hellandite group (Oberti et al., Reference Oberti, Langone, Boiocchi, Bernabè and Hawthorne2019; Della Ventura et al., Reference Della Ventura, Bonazzi, Oberti and Ottolini2002), and more than 1:1 in the gadolinite-group minerals (Bačík et al., Reference Bačík, Miyawaki, Atencio, Cámara and Fridrichová2017). Our data on hellandite-(Y) do not exclude the presence of Be – some of the Raman bands at 810–790 cm–1 and 770–768 cm–1 could be attributed not only to Si–O but also Be–O stretching vibrations. However, the presence of sharp O–H peaks at 3454 and 3662 cm–1 indicate a significant proportion of OH– groups in the T site cavity. Therefore, we can assume that the possible Be (or Li) occupancy at the T site of hellandite-(Y) is very probably limited, however without accurate measurement, this limit cannot be quantified. Consequently, if the Be:B ratio significantly exceeds 1:1 in the environment, hingganite-(Y) preferentially crystallises and binds Be, Fe2+, and also a small amount of remaining B.
Although allanite-(Ce) and LREE-rich epidote are typical reaction products which accumulate LREE during monazite-(Ce) alteration in common granitic and metapelitic rocks over a wide P–T range, the analogous replacement of xenotime-(Y) produces only a limited amount of allanite-(Y), HREE+Y-rich epidote, and a significant amount of HREE+Y can be accommodated by apatite-supergroup minerals (cf. Broska et al., Reference Broska, Williams, Janák and Nagy2005; Budzyń et al., Reference Budzyń, Harlov, Kozub-Budzyń and Majka2017; Hentschel et al., Reference Hentschel, Janots, Trepmann, Magnin and Lanari2020). Moreover, allanite-(Y) and epidote-group minerals, rich in HREE+Y, are very rare in nature and occur mainly in highly evolved felsic rocks, e.g. granitic pegmatites, rare-metal (leuco)granites, and associated metamorphic REE mineralisation veins (e.g. Gieré and Sorensen, Reference Gieré, Sorensen, Liebscher and Franz2004; Alekseev et al., Reference Alekseev, Marin and Gembitskaya2013, Reference Alekseev, Marin and Gembitskaya2016). These data, together with our observations, raise a question regarding the relative stability between LREE-rich epidote-group minerals and HREE+Y-rich epidote-group minerals in magmatic and metamorphic systems, and suggest that the HREE and Y mainly reside in other minerals (Gieré and Sorensen, Reference Gieré, Sorensen, Liebscher and Franz2004). A possible explanation for this disparity in the Fabova Hoľa granite samples is a higher B activity during mylonitisation (~15–20 ppm B in the metagranite; Marsina et al., Reference Marsina, Bodiš, Havrila, Janák, Káčer, Kohút, Lexa, Rapant and Vozárová1999), which favours the formation of borosilicates instead of epidote-group minerals. A similar scenario for hydrothermal tourmalinisation, and its influence on LREE vs. HREE accumulation and allanite formation was proposed by Balashov (Reference Balashov1976) and later by Alekseev et al. (Reference Alekseev, Marin and Gembitskaya2016) regarding allanite-(Ce) and allanite-(Y) paragenesis in a tourmalinite from the Severnyi pluton, Chukchi Peninsula, Russia. The stability of LREE-rich epidote-group minerals vs. HREE+Y-rich gadolinite-group minerals and the HREE+Y-rich (boro)silicate alternatives are also evident in the Strange Lake peralkaline pluton in Canada, where LREE mobility was controlled largely by the stability of ferriallanite-(Ce), whereas HREE was controlled by gadolinite-(Y) (Gysi et al., Reference Gysi, Williams-Jones and Collins2016; Vasyukova and William-Jones, Reference Vasyukova and Williams-Jones2019). However, little is currently known regarding the formation of HREE+Y-rich epidote-group minerals vs. HREE+Y-rich gadolinite-group minerals during alteration of natural xenotime-(Y). Moreover, though a few experiments have focused on the relative stabilities of xenotime-(Y)–HREE+Y-rich epidote–HREE+Y-rich fluorapatite in high Ca and Na–Ca environments under different P–T conditions (e.g. Budzyń et al., Reference Budzyń, Harlov, Kozub-Budzyń and Majka2017), none have considered a B-(Be) saturated environment.
Crystal-chemical data for secondary (boro)silicates indicate solid solutions with a general Ca–REE+Y substitution trend (Fig. 10). This suggests that a major change in fluid chemistry occurred during hydrothermal alteration of monazite-(Ce) and xenotime-(Y), which was substantially controlled by the activity of Ca. Moreover, the remobilisation and distribution of LREE vs. HREE+Y was controlled by the competition between hydrothermal fluids and the stability of primary REE phosphates, as well as by the stability of secondary LREE epidote-group minerals and HREE+Y gadolinite-group minerals (cf. Gysi et al., Reference Gysi, Williams-Jones and Collins2016). Formation of hellandite-(Y) and hingganite-(Y) in the Fabova Hoľa metagranite may have resulted from localised heterogeneity and a Y–B–Be saturated environment occurring in the proximity of the altered xenotime-(Y), instead of the more common accessory epidote-group minerals or tourmaline. This has been documented in metagranites in the broader vicinity of the FAH-3 sample (Dubík Reference Dubík1991; Putiš Reference Putiš1994). Hingganite-(Y) would have crystallised from Y–Be–B-rich fluids in the same manner.

Fig. 10. Composition of secondary (boro)silicates in REE+Y vs. total Ca substitution diagram (atomic proportions) with ideal Ca:REE+Y substitution vectors (straight lines). Note: the REE+Y content in hellandite-(Y) is restricted to the X site only.
Age of primary monazite-(Ce), formation of coronae as a function of P–T conditions, and the relationship to Alpine mylonitisation
An age of 355 ± 1.9 Ma obtained by in situ Th–U–total Pb EPMA of primary magmatic monazite-(Ce) are consistent with the SIMS zircon U–Pb radiometric age determination of the corresponding I-type granitic rocks from the Tatric and Veporic units (~350–360 Ma; Broska et al., Reference Broska, Petrík, Be´eri-Shlevin, Majka and Bezák2013), including the Sihla type tonalite dated to ca. 355 Ma directly from the Vepor pluton (Kohút et al., Reference Kohút, Uher, Putiš, Sergeev and Antonov2008). It represents a primary magmatic age for emplacement of the granite during the main stage of Variscan plutonic activity in the West-Carpathian Tatric and Veporic crystalline basement (Poller et al., Reference Poller, Janák, Kohút and Todt2000; Gaab et al., Reference Gaab, Poller, Janák, Kohút and Todt2005; Burda and Gawęda Reference Burda and Gawęda2009; Kohút et al., Reference Kohút, Uher, Putiš, Sergeev and Antonov2008; Broska et al., Reference Broska, Petrík, Be´eri-Shlevin, Majka and Bezák2013; Burda et al., Reference Burda, Gawęda and Klötzli2013a, Reference Burda, Gawęda and Klötzli2013b; Gawęda et al., Reference Gawęda, Burda, Klötzli, Golonka and Szopa2016; Kohút and Larionov, Reference Kohút and Larionov2021).
Determining the age from analysis of marginal parts of the monazite-(Ce) grains and isolated tiny monazite-(Ce) relics resulted in a scatter of younger ages (300 to 90 Ma). This less precise age interval indicates a disturbance in Th–U–Pb abundances, which was probably induced during alteration and coronae formation around monazite-(Ce) that resulted in partial-to-total Pb removal and partial resetting of the geochronometer (cf. Harlov et al., Reference Harlov, Wirth and Hetherington2011; Williams et al., Reference Williams, Jercinovic, Harlov, Budzyń and Hetherington2011).
The exhumation of mylonitised metagranites of the Fabova Hoľa Complex at ca. 90–85 Ma was constrained by 40Ar–39Ar phengite ages from the extensional shear zones (Putiš, Reference Putiš1994; Dallmeyer et al., Reference Dallmeyer, Neubauer, Handler, Fritz, Müller, Pana and Putiš1996; Putiš et al., Reference Putiš, Ivan, Kohút, Spišiak, Siman, Radvanec, Uher, Sergeev, Larionov, Méres, Demko and Ondrejka2009a). The Late Cretaceous to Palaeocene uplift of the Veporic Unit is also documented by the zircon fission track age of the Sihla tonalite (64.9 ± 4.8 Ma; Plašienka et al., Reference Plašienka, Broska, Kissová and Dunkl2007). Deformation here terminated in a brittle–ductile regime, and the opened ‘en-echelon’ or ‘pinch and swell structures’ are still infilled by late- to post-metamorphic mineralisation. The greenschist-facies tectono-metamorphic conditions during the metagranite exhumation and blastomylonitisation may alternatively constrain the conditions of coronae formation (Putiš, Reference Putiš1994; Putiš et al., Reference Putiš, Unzog, Wallbrecher, Fritz, Grecula, Hovorka and Putiš1997b; Janák et al., Reference Janák, Plašienka, Frey, Cosca, Schmidt, Lupták and Méres2001; Lupták et al., Reference Lupták, Janák and Plašienka2000; Jeřábek et al., Reference Jeřábek, Stünitz, Heilbronner, Lexa and Schulmann2007, Reference Jeřábek, Faryad, Schulmann, Lexa and Tajčmanová2008a, Reference Jeřábek, Janák, Faryad, Finger and Konečný2008b). The coronae-bearing domains could be indicators of a channelised fluid flow during the exhumation. These domains are always located in a granitic blastomylonitic groundmass composed of newly formed medium-pressure greenschist-facies minerals, such as quartz-2, albite, chlorite, phengite, biotite-2, epidote, clinozoisite, ±grossular-rich garnet and calcite. Therefore, the estimated P–T conditions of corona formation are from ca. 450 to 300°C following the exhumation ductile deformation regime and still a high late-metamorphic fluid activity. The outer rims of coronae are frequently ingrowing the hosting deformed biotite-1, being partly altered to phengite and chlorite (Fig. 9). Similarly, the brittle fractures of the neighbouring semiductile feldspars are infilled by quartz, albite and chlorite.
Possible sources of B and Be
The presence of hellandite-(Y) and hingganite-(Y) in the coronae mineral aggregates requires a source of B and Be, which could have been remobilised during fluid-mediated breakdown of xenotime-(Y). Two principal sources of B and Be could be: (1) internal origin from rock-forming or accessory minerals of the host metagranite; or (2) resulting from external sources such as adjacent magmatic, metamorphic and sedimentary rocks.
The B content in common plutonic and metamorphic plagioclase and K-feldspar can usually be up to 100 ppm B, which could be incorporated as the (Na,K)BSi3O8 molecule in the feldspars. Boron could have been leaked into the circulating fluid during the post-magmatic orthoclase to microcline ordering of K-feldspar (Grew, Reference Grew, Grew and Anowitz2002a). Plagioclase is a principal source of B in common tourmaline-free granites. It contributes up to 69 wt.% of the whole B content in the rock (Sauerer and Troll, Reference Sauerer and Troll1990). The mica-group minerals, especially muscovite, also represent an important carrier of B among granitic rock-forming minerals, where B substitutes for Al in the tetrahedral site as a boromuscovite molecule (Grew, Reference Grew, Grew and Anowitz2002a). The concentration of Be in rock-forming minerals of common two-mica granites is generally very low, usually ≤10 ppm (Kretz et al., Reference Kretz, Loop and Hartree1989).
Alternatively, or even additionally, the potential in situ source of B could be the altered xenotime-(Y) itself. The xenotime contains traces of B, which has been documented by X-ray compositional mapping (Fig. 5i). The incorporation of B into the xenotime-(Y) structure is most probably controlled by coupled heterovalent substitution (Nb,Ta)BY–1P–1 (Nb5+, Ta5+ + B3+ ↔ Y3+ + P5+), though in a limited range due to a very low Nb content, (close to the detection limit) in the xenotime-(Y). The natural occurrences of schiavinatoite (NbBO4) and béhierite (TaBO4), together with the Nb–Ta orthoborates with a zircon–xenotime type crystal structure (Mrose and Rose, Reference Mrose and Rose1962; Range et al., Reference Range, Wildenauer and Andratschke1996; Demartin et al., Reference Demartin, Diella, Gramaccioli and Pezzotta2001a; Finch and Hanchar, Reference Finch, Hanchar, Hanchar and Hoskin2003), together with previous indications of B replacing P in xenotime-(Y) (Oftendal, Reference Oftendal1964), support the presence of B in the xenotime-(Y) and the existence of a possible limited xenotime-(Y)–schiavinatoite–béhierite solid solution as a source of B.
The second possibility represents an external source of B and Be during the Alpine tectono-thermal overprint of the Fabova Hoľa metagranites The B-bearing environment, necessary for hellandite-(Y) and hingganite-(Y) formation, is supported by the occurrence of abundant newly formed tourmaline neoblasts in the adjacent Variscan Veporic metatonalites and tourmalinite pebbles in the Permian basal conglomerates (Vozárová et al., Reference Vozárová, Rodionov, Vozár, Lepekhina and Šarinová2016), as well as in the surrounding Palaeozoic micaceous metapelites (cf. Lupták et al., Reference Lupták, Janák and Plašienka2000; Janák et al., Reference Janák, Plašienka, Frey, Cosca, Schmidt, Lupták and Méres2001; Jeřábek et al., Reference Jeřábek, Janák, Faryad, Finger and Konečný2008b). Schorlitic tourmaline is a mineral commonly found in the Permian Klenovec-type granite, which occurs as several small intrusions in the Veporic Unit (Hraško et al., Reference Hraško, Broska, Finger, Michalik, Šimon and Vozár2002; Villaseñor et al., Reference Villaseñor, Catlos, Broska, Kohút, Hraško, Aguilera, Etzel, Kyle and Stockli2021).
Consequently, B and Be could have been mobilised during Alpine mylonitisation, and in the associated tectono-thermal fluid that overprinted the in situ rock-forming minerals, especially muscovite and feldspars. These are the principal host phases for B and also Be, considering their high modal abundance in the parental granitic rocks (cf. Domanik et al., Reference Domanik, Hervig and Peacock1993; Leeman and Sisson, Reference Leeman, Sisson, Grew and Anovitz1996; London et al., Reference London, Morgan VI, Wolf, Grew and Anovitz1996; Grew, Reference Grew, Grew and Anowitz2002a, Reference Grew and Grew2002b; and references therein).
Acknowledgements
This work is dedicated to the memory of Jarmila Luptáková, PhD, who sadly passed away aged 45 on Sunday 27th June, 2021. This work was supported by the Slovak Research and Development Agency under contracts APVV–15–0050, APVV–18–0065, APVV–19–0065, and VEGA Agency Nos. 1/0467/20 and 1/0151/19. We thank P. Konečný and V. Kollárová for providing the EPMA facilities, as well as Michael J. Sabo for reviewing the English content. Finally, we thank Daniel Harlov, Fernando Cámara, one anonymous reviewer and Roger Mitchell (Principal Editor) for their constructive suggestions.
Supplementary material
To view supplementary material for this article, please visit https://doi.org/10.1017/S0007123422000011