Introduction
Returning samples of the Martian regolith to Earth is a complex goal that NASA and ESA are studying together. It requires intricate planning and novel engineering in order to meet the stringent planetary protection requirements established to keep Earth safe. Planetary protection is the practice of protecting solar system bodies from ‘forward contamination’ by Earth life and protecting Earth from ‘backward contamination’ due to possible adverse effects that may be returned from other solar system bodies (NID 8020.109A).
One of the key factors in understanding any potential extant or extinct biology on Mars is that it is not to be irrevocably contaminated before its existence can be confirmed, a critical facet of forward planetary protection. Should such life exist, it becomes critically important to prevent harmful backward contamination from a putative extraterrestrial life form, during sample return missions to Earth. While there have been previous successful sample return missions from solar system bodies (e.g. Stardust, Genesis and Hayabusa), these destinations were deemed to not have the potential to support life, and thus, were classified as ‘unrestricted Earth return’ missions as those destinations were deemed not to be of direct interest for understanding the process of chemical or biological evolution or where exploration would not be jeopardized by terrestrial contamination.
The challenge of preventing biological contamination by potential extraterrestrial sources was last addressed by NASA in 1969 when the Apollo 11 and 12 astronauts returned from the Moon and were quarantined until it was determined that contact with lunar dust and rocks did not present a biological threat to Earth (Interagency Committee on Back Contamination 1967). Given the present developments in increased capability in government, commercial and private spacecraft to reach the Moon and beyond, it is conceivable that astronauts could land and explore the surface of Mars within the next two decades. Consequently, it is imperative to demonstrate the ability to safely handle material returned from Mars through deployment of reliable spacecraft, robust containment, actively engineered inactivation processes and an understanding of passive inactivation processes. The Committee on Space Research (COSPAR) established by the International Council for Science in 1958 further outlines an international planetary protection policy (Kminek et al. Reference Kminek, Conley, Hipkin and Yano2017; Committee on Space Research 2020). The foundation of the COSPAR policy is established in the 1967 Outer Space Treaty, Article IX, which allows for the study of celestial bodies and their moons such that exploration of those bodies ‘avoids their harmful contamination and also adverse changes in the environment of the Earth resulting from the introduction of extraterrestrial matter, and where necessary shall adopt appropriate measures for this purpose’ (United Nations 1967). In accordance with COSPAR language, NASA adopted similar planetary protection policies which categorize space missions according to the type of encounter (i.e. flyby, orbiter, lander or sample return) and the target destination, including any bodies encountered en-route (National Aeronautics and Space Administration 2011, 2017).
Sample return missions (Category V missions) are classified depending on the target body from where the samples are planned to be collected. If the body has no indication of potential indigenous life, it is categorized as ‘unrestricted’; however, if there is scientific evidence to support potential indigenous biological life, then the mission is ‘restricted’ (NID 8020.109A; Committee on Space Research 2020). As the complexity of these biological questions increases, the stringency of NASA planetary protection requirements imposed on the mission also increases in direct proportion. For ‘restricted’ missions, NASA policy expresses the highest level of backward planetary protection (BPP) concerns for the mission; specifically ‘the need for containment throughout the return phase of all returned hardware which directly contacted the target body or unsterilized material from the body, and the need for containment of any unsterilized sample collected and returned to Earth’. Additionally, samples must remain contained ‘unless treated by an effective sterilizing procedure’.
NASA's Interim Directive governing restricted sample return (NID 8020.109A) stipulates that ‘Unless the sample to be returned is subjected to an accepted, approved, sterilization process, the sample container must be sealed after sample acquisition, and a redundant, fail-safe containment … shall be required’ and ‘… no uncontained hardware that contacted Mars, directly or indirectly, may be returned to Earth unless sterilized’.
To date, for BPP, there have been previous reports from planetary protection working groups (Rummel et al. Reference Rummel, Race, DeVincenzi, Schad, Stabekis, Viso and Acevedo2002; Allwood et al. Reference Allwood, Beaty, Bass, Conley, Kminek, Race, Vance and Westall2013; Kminek et al. Reference Kminek, Conley, Allen, Bartlett, Beaty, Benning, Bhartia, Boston, Duchaine, Farmer, Flynn, Glavin, Gorby, Hallsworth, Mogul, Moser, Price, Pukall, Fernández-Remolar, Smith, Stedman, Steele, Stepanauskas, Sun, Vago, Voytek, Weiss and Westall2014) composed of scientists and engineers investigating solutions that can effectively contain the returned samples and sterilize uncontained Martian particles. In order to generate consensus on what type of a baseline ‘sterilization’ approach could be adopted for MSR, among a range of scientific and other stakeholders (NASA, public, intra- and intergovernmental agencies, and industry), the NASA Planetary Protection Officer, supported by the Mars Sample Return (MSR) study, convened an informal group of 20–30 sterilization subject matter experts (SMEs) from the biopharmaceutical industry, academia and government to form the Sterilization Working Group (SWG) which met over the course of three workshops held on 30–31 January 2019, 10–11 June 2019 and 19–20 February 2020. The questions that were discussed as part of this collaboration were:
(a) What is the likely nature of organisms (life) on Mars and how might this impact the analysis of inactivation processes?
(b) What are the most likely mechanisms of contamination of the returning spacecraft?
(c) Can sterilization concepts on Earth be applied to BPP?
(d) What modes of passive inactivation exist that will act upon any contamination travelling on or around the OS?
(e) What do we know about the active inactivation inherent in the brazing operation as part of break-the-chain of contact with Mars?
(f) Based on a risk assessment of the current system architecture, are additional active sterilization processes required to ensure BPP?
(g) What future work is needed to make these assessments?
Proposed MSR campaign overview
MSR campaign continues to be a high priority in the planetary science community and an enduring goal of international planetary exploration programmes. From the earliest Mars missions, it was recognized that the cost and complexity of sending instruments to study Mars would always limit the investigation of Mars as a system. The scientific community has long held that, in combination with global and in situ investigations, terrestrial analysis of carefully selected Mars samples would be needed to understand the complex history of Mars. As detailed in the report of the International MSR Objectives and Samples Team (iMOST) scientific community (Beaty et al. Reference Beaty, Grady, Mcsween, Sefton-Nash, Altieri, Amelin, Ammannito, Anand, Benning, Bishop, Borg, Boucher, Brucato, Busemann, Campbell, Czaja, Debaille, Marais, Dixon, Ehlmann, Farmer, Fernandez-Remolar, Filiberto, Fogarty, Glavin, Goreva, Hallis, Harrington, Hausrath, Herd, Horgan, Humayun, Kleine, Kleinhenz, Mackelprang, Mangold, Mayhew, McCoy, McCubbin, Mclennan, Moser, Moynier, Mustard, Niles, Ori, Raulin, Rettberg, Schmitz and Kate2019), the proposed functional objectives for a potential MSR campaign include the following:
• Acquire and return to Earth a scientifically selected set of Mars samples for investigation in terrestrial laboratories.
• Select samples based on their geologic diversity, astrobiological relevance and geochronological significance.
• Establish the field context for each sample using in situ observations.
• Ensure the scientific integrity of the returned samples through contamination control (including round-trip Earth contamination and sample-to-sample cross-contamination) and control of environments experienced by the samples after acquisition.
• Ensure compliance with planetary protection requirements associated with the return of Mars samples to Earth's biosphere.
• Achieve a set of sample-related scientific objectives including evidence of life, geologic environments, geochronology, volatiles, planetary-scale geology, environmental hazards and In Situ Resource Utilization (ISRU).
The architectural design of the proposed MSR campaign consists of a Mars lander including a fetch rover, and Mars ascent vehicle, Earth return orbiter, and associated payload for processing the Orbiting Sample (OS) container. The goal is to return geological samples collected on the Mars 2020 mission from Mars to Earth as early as 2031. In a series of joint missions between NASA and ESA, launching as early as 2026, the notional MSR Architecture (Fig. 1) would launch a Sample Retrieval Lander (SRL) and an Earth Return Orbiter (ERO). Once the sample tubes are loaded into the OS container by the SRL, the Mars Ascent Vehicle System (MAS) would then deliver the samples into low Martian orbit. After the ERO conducts a rendezvous with the OS, the Capture, Containment, and Return System (CCRS) would capture the OS and processes it for Earth return. Following this, the ERO would depart Mars, returns to Earth, and puts the Earth Entry Vehicle (EEV) on its trajectory to landing on Earth. As part of this this process, MSR would ‘break-the-chain’ (BTC) of contact between the Martian environment and the biosphere of Earth. This process would likely include Mars analysis of Mars surface particle transport between spacecraft, control of the amount of material, transfer into defined clean zones on mission elements and highly robust containment assurance hardware and procedures. When coupled with the qualitative assessment of the low risk of Martian material to Earth's biosphere (as discussed later in this paper), this set of operations creates a robust strategy for a reliable sample return campaign.

Fig. 1. Notional Mars Sample Return Campaign Architecture and Roles – arrow colours indicate roles: red is NASA Mars 2020, orange is NASA SRL, blue is ESA ERO and green is NASA and international community (Lock et al. Reference Lock, Nicholas, Vijendran, Woolley, Didion, Laipert and Olikara2019).
Working group discussion topics and conclusions
Mars biology
What are the potential problems with bringing Martian samples to Earth?
NASA and other space-faring agencies and nations are levying requirements for the safe return of samples from extraterrestrial bodies as per the obligations outlined in the Outer Space Treaty and are expending significant effort to mitigate any potential risks to Earth's biosphere from the return of samples from extraterrestrial bodies. Although adverse consequences could conceivably result from the introduction of extraterrestrial material into Earth's biosphere (as will be discussed below), the SWG concluded, as similar working groups sponsored by the National Research Council (NRC) and the European Science Foundation (ESF) have previously done concerning returning samples from Mars (National Research Council 2009 and 1997; Ammann et al. Reference Ammann, Baross, Bennett, Bridges, Fragola, Kerrest, Marshall-Bowman, Raoul, Rettberg, Rummel, Salminen, Stackebrandt and Walter2012), the likelihood of such a risk transpiring is considered to be extremely low. Specifically, these past reports have noted that:
‘…contamination of Earth by putative Mars microorganisms is unlikely to pose a risk of significant ecological impact or other significant harmful effects. The risk is not zero, however…’ (National Research Council 1997)
‘…the potential for large-scale pathogenic effects arising from the release of small quantities of pristine Mars samples is still regarded as being very low.’ …‘extreme environments on Earth have not yet yielded any examples of life forms that are pathogenic to humans’ (National Research Council 2009)
‘…the potential for large-scale effects on the Earth's biosphere by a returned Mars life form appears to be low, but is not demonstrably zero.’ (Ammann et al. Reference Ammann, Baross, Bennett, Bridges, Fragola, Kerrest, Marshall-Bowman, Raoul, Rettberg, Rummel, Salminen, Stackebrandt and Walter2012).
While it is impossible to remove all risk without ceasing space exploration, further analysis of BTC containment and inactivation strategies is essential to provide crucial data on the claim that a MSR mission could achieve a very high probability of not releasing unsterilized particles. There is always some level of risk associated with exploration into the unknown, and it was the goal of the SWG to help manage the risks of possible adverse effects to the Earth's biosphere while maintaining the science integrity of the returned samples.
The Martian environment and the potential for life
Seeking evidence of life from extraterrestrial sources is one of the great scientific goals and challenges of our time. One of the best ways we can address this is by exploring Mars, an object that shared with Earth a similar early geological history, particularly during the time when life appeared on our planet. If life ever arose on the Red Planet, it probably did when Mars was wetter, sometime within the first half billion years after planetary formation (Nisbet and Sleep Reference Nisbet and Sleep2001; Zahnle et al. Reference Zahnle, Arndt, Cockell, Halliday, Nisbet, Selsis and Sleep2006). Conditions then were similar to those when life gained a foothold on the young Earth prior to 3.8 billion years ago. This makes Mars a primary target to search for signs of life in our Solar System.
It is likely that 4.45 billion years ago, early Mars also had developed a global ocean (or large bodies of water) enveloped in a 1 bar, mostly CO2 atmosphere (Elkins-Tanton Reference Elkins-Tanton2011). However, Mars is much further away from the Sun than Earth, and is smaller; therefore, in the absence of other inputs, Mars would have quickly frozen over (Fairén et al. Reference Fairén, Haqq-Misra and McKay2012). But, as on Earth, we can expect that active sub-surface hydrothermal processes driven by internal heat may have helped raise surface temperatures by releasing CO2, CH4 and other gases (Pavlov et al. Reference Pavlov, Kasting, Brown, Rages and Freedman2000; Oze and Sharma Reference Oze and Sharma2005; Schulte et al. Reference Schulte, Richards, Daly, Kurz, McDonald and Holden2006). The extensive subglacial, submerged and emerged volcanic/hydrothermal activity would have resulted in numerous liquid water-rich settings (Warner and Farmer (Reference Warner and Farmer2010); Cousins and Crawford, Reference Cousins and Crawford2011). The right mixture of ingredients, temperature and chemical gradients, organic molecule transport and concentration, and fixation processes could have been found just as well in a plethora of terrestrial submarine vents or in a multitude of vents under top-frozen Martian bodies of water (Westall et al. Reference Westall, Loizeau, Foucher, Bost, Betrand, Vago and Kminek2013; Russell et al. Reference Russell, Barge, Bhartia, Bocanegra, Bracher, Branscomb, Kidd, McGlynn, Meier, Nitschke, Shibuya, Vance, White and Kanik2014).
Although extraterrestrial life could conceivably be different from life on Earth, the SWG concurred that it is most likely to be carbon and water based, or at the very least to share Earth's fundamental chemistries (e.g. covalent and ionic bonds) (Berg et al. Reference Berg, Tymoczko, Gatto and Stryer2019). Additional information on the use of fundamental chemistries is included subsequently in this paper.
Life originated on Earth prior to 3.8 billion years ago; and if life originated on Mars, it probably arose during the same time-period given the similarities in the genesis of the two planets. With regards to life on Mars, there are several scenarios that could have occurred, including:
• Mars has always been a lifeless planet.
• Life originated on Mars independent of life on Earth and went extinct or is extant in diverse refugia in the lithosphere.
• Life originated on Mars and, during interplanetary traffic of rocky material in the early solar system (Bottke and Norman Reference Bottke and Norman2017), and subsequently was transported to Earth.
• Life originated on Earth and, during interplanetary traffic of rocky material in the early solar system, and was transported to Mars.
If life originated on Mars and was not transported to Earth it might bear little resemblance to life on Earth, but it still most likely shares our fundamental chemistries of chemical bonds. The detection of divergent life on Mars would reveal a true second genesis of life in the universe. Thus, any life detected on Mars might not only prove that there is life on other planets but it might also influence our models for the origin of life on Earth. If there is life on Mars, there are three scenarios under which life on Mars and Earth may both use DNA and RNA (or analogous nucleic acids as hereditary materials):
(1) Transfer of life between the two planets.
(2) Transfer of life from a more distant common source to both planets.
(3) Independent development of the same DNA/RNA-based life forms.
Because life does exist on Earth, transfer between the planets would be the most reasonable of these scenarios if indeed life ever existed on Mars. Common ancestry of life on Earth and Mars would be dependent upon impact events (e.g. meteorites, comets, asteroids) resulting in ejection, transit through high radiation interplanetary space, entry and adaptation to another world. Recent studies suggest Martian meteorites were transferred to the Earth at shortened time scales and with higher fluxes than previously believed (Gladman and Burns Reference Gladman and Burns1996; Gladman et al. Reference Gladman, Migliorini, Morbidelli, Zappalà, Michel, Cellino, Froeschlé, Levison, Bailey and Duncan1997; Mileikowsky et al. Reference Mileikowsky, Cucinotta, Wilson, Gladman, Horneck, Lindegren, Melosh, Rickman, Valtonen and Zhengi2000). The final destination of 7% of Martian meteorites is thought to be the Earth, delivering an estimated one billion tons of debris over the history of the solar system (Gladman and Burns Reference Gladman and Burns1996). Several dozen SNC meteorites (i.e. meteorites named after the locations where examples of these meteorites were first found – Shergotty (India), Nakhla (Egypt) and Chassigny (France)) of Martian origin have been discovered here on Earth, and analyses indicate that 20% of Martian meteorites have only experienced mild internal heating during ejection and impact (Weiss et al. Reference Weiss, Kirschvink, Baudenbacher, Vali, Peters, Macdonald and Wikswo2000; Fritz et al. Reference Fritz, Artemieva and Greshake2005; Shuster and Weiss Reference Shuster and Weiss2005). Low internal heating during ejection occurs because of interference between the impact shock wave and the reflected shock wave, leading to lower shock pressures but higher velocities near the target surface. Empirical studies showed that certain types of microbes could survive the requisite shock pressures (Gratz et al. Reference Gratz, Nellis and Hinsey1993; Horneck et al. Reference Horneck, Stöffler, Ott, Hornemann, Cockell, Moeller, Meyer, Vera, Fritz, Schade and Artemieva2008). Atmospheric entry heats the surfaces to unsurvivable temperatures, but because atmospheric transit occurs so quickly, the internal temperatures of meteorites can stay quite moderate below 100°C consistent with the observations of ALH84001 (Fritz et al. Reference Fritz, Artemieva and Greshake2005) where intact amino acids were found. Once life had evolved on one planet, this meteoritic transfer rate makes it plausible that the adjacent planets could ‘catch’ life rather than independently evolve life (Davies Reference Davies2003).
Of the more than 60 000 meteorites that have been catalogued on Earth, 246 have been identified as originating from Mars, including one weighting 18 kg that fell in 1962 (Gladman and Burns Reference Gladman and Burns1996). Thus, the few hundred grams of Martian dust that would be delivered by the MSR would not be the first Martian material to be transferred to Earth. As discussed above, there has been significant, previous meteoritic transfer of Martian materials to Earth, and Earth materials to Mars. Therefore, it is plausible that Earth has been previously exposed to Martian microbiota (if present) and that the Martian material has already had the opportunity to ‘contaminate’ Earth's biosphere yet has caused no known effects.
Two major challenges during transit are desiccation and radiation. Desiccation tolerance may be enhanced through entombment in salt crystals or rock fissures. Certain types of dormant Earth-based microorganisms can show dramatic resistance to drying and heat inactivation when encased on crystals (Doyle and Ernst, Reference Doyle and Ernst1967). UV is easily shielded by tens of microns of material, as evidenced by low survival of single layers of microorganisms but high survival of thicker biofilms of Bacillus subtilis (including dormant spores) exposed to space for 1.5 years on-board the International Space Station (Horneck et al. Reference Horneck, Moeller, Thierry, Mancinelli, Nicholson, Panitz, Rabbow, Rettberg, Spry, Stackebrandt, Vaishampayan and Venkateswaran2012). To avoid radiation damage due to cosmic rays, rapid transit or protection by 1m or more of rock would be critical. Low temperatures during interplanetary transit largely stop water chemistry making it a lesser issue with respect to microbial death (and in fact more of a preservation process), so the limiting factor for survival of nucleic acids may be space radiation that is mitigated through time (short transit time to Earth) and shielding (meteorite composition, thickness, etc.).
Meteoritic exchange in the Solar System was 100–1000 times more intense during the heavy bombardment stage 4 billion years ago than it is now. There are signs of numerous fluid flows (Malin and Edgett Reference Malin and Edgett2000a, Reference Malin and Edgett2000b), a possible ancient ocean (Head et al. Reference Head, Hiesinger, Ivanov, Kreslavsky, Pratt and Thomson1999) and sedimentary formations on Mars that suggest a relatively warmer and wetter Mars 3–4 billion years ago. Thus, during the time of extensive meteoritic exchange 4 billion years ago, Earth and Mars had more similar environments, with Mars being much wetter than it is today (Malin and Edgett Reference Malin and Edgett2000a, Reference Malin and Edgett2000b). By the Archean epoch (2.5–4 billion years ago), microbial evolution on Earth may have already proceeded to the point of modern microbial morphologies and enzymatic carbon metabolism with isotopic fractionation (Mojzsis et al. Reference Mojzsis, Krishnamurthy, Arrhenius, Gesteland, Cech and Atkins1999). All known cell-based organisms on Earth share a core of about 500 genes, some or all of which were inherited from a common ancestor. This includes the most conserved of those genes, the small subunit ribosomal RNA gene 16S rRNA in prokaryotes and 18S rRNA in eukaryotes. This common ancestor has been hypothesized to be an archaeal-like hyperthermophile 3–4 billion years ago whose metabolism exploited oxidation/reduction gradients (Pace Reference Pace1991).
Subsequently, the environments on Mars and Earth have diverged: the appearance of oxygen on the Earth 2.5 billion years ago led to the formation of the ozone layer, which decreased UV radiation, while Mars lost its atmosphere as its magnetic field decayed, causing an increase in UV exposure, cooling of the surface and loss of surface water. Extant life on the Martian surface would need to survive temperatures and pressures below the triple point of water, high UV exposure and the oxidizing surface chemistry presumably induced by UV radiation. Despite these extreme conditions, it has been shown that only a thin layer of soil is needed to protect microbes from UV exposure (Cockell et al., Reference Cockell, Catling, Davis, Snook, Kepner, Lee and McKay2000; Mancinelli and Klovstad Reference Mancinelli and Klovstad2000; Schuerger et al. Reference Schuerger, Mancinelli, Kern, Rothschild and McKay2003). In addition, the redox gradient resulting from UV irradiation might actually power microbial metabolism, just as redox gradients in the Earth's crust drive chemolithotrophic metabolism. While there is no doubt that Mars is currently an extreme environment, the adaptability of microbial life on Earth – for example, extremophiles in the driest deserts or coldest Arctic climates – does not make it unreasonable to propose that Martian microbes could have adapted to the gradual decline in water, temperature and UV protection over the past few billion years; and just as the adapted and diverged microbes in Earth extreme environments still bear the signature of their common ancestry in their conserved genes, Martian microbes may also bear theirs as well.
Even if life did transfer between Mars and Earth 4 billion years ago and thrive in the early Martian environment, it may now only thrive in very particular locations (i.e. refugia), for example, deep in the crust where the temperature rises above that of the frozen surface, or at particular volcanic thermal vents. There may also be regions on Mars where liquid water is not in short supply such as the regions near the ice-rich polar ice caps, especially the north cap with its seasonal variation in ice, and perhaps liquid water underneath (Orosei et al. Reference Orosei, Lauro, Pettinelli, Cicchetti, Coradini, Cosciotti, Di Paolo, Flamini, Mattei, Pajola, Soldovieri, Cartacci, Cassenti, Frigeri, Giuppi, Martufi, Masdea, Mitri, Nenna, Noschese, Restano and Seu2018), where it would be expected to have the highest water levels near the surface. In addition, the D/H ratio (deuterium and hydrogen ratio) of water in Martian meteorites suggests a much larger reservoir of water in the crust that is not in equilibrium with the atmosphere (Donahue Reference Donahue1995). This water is predicted to be liquid a few kilometres into the crust, where temperatures rise above surface temperature. In addition, there is evidence for relatively recent Martian volcanic activity, suggesting sources of temperature gradients and fluid flows just below the Martian surface near these sites Malin et al. Reference Malin, McEwen, Carr, Soderblom, Thomas, Danielson, James, Veverka and Hartmann1999. There may also be local hydrothermal systems near the regions of recent volcanic activity (Malin et al. Reference Malin, McEwen, Carr, Soderblom, Thomas, Danielson, James, Veverka and Hartmann1999). InSight lander's seismometer, SEIS, the Seismic Experiment for Interior Structure, detected 174 ‘marsquakes’ over 207 sols, indicating that Mars is seismically active (Giardini et al. Reference Giardini, Lognonné, Banerdt, Pike, Christensen, Ceylan, Clinton, Driel, Stähler, Boese, Garcia, Khan, Panning, Perrin, Banfield, Beucler, Charalambous, Euchner, Horleston, Jacob, Kawamura, Kedar, Mainsant, Scholz, Smrekar, Spiga, Agard, Antonangeli, Barkaoui, Barrett, Combes, Conejero, Daubar, Drilleau, Ferrier, Gabsi, Gudkova, Hurst, Karakostas, King, Knapmeyer, Knapmeyer-Endrun, Llorca-Cejudo, Lucas, Luno, Margerin, McClean, Mimoun, Murdoch, Nimmo, Nonon, Pardo, Rivoldini, Manfredi, Samuel, Schimmel, Stott, Stutzmann, Teanby, Warren, Weber, Wieczorek and Yana2020), however the buildup of massive volcanic cones do not favour active plate tectonics; a key element for hydrothermal vents and the biology that thrives on their energy and nutrient flows.
Martian biological risk assessment: a discussion
The Mars 2020 sample cache will be collected from roughly the first 6–7 cm of the Martian surface, a very harsh, desiccating environment exposed to solar UV radiation. If Martian life is carbon-based, it is likely to be susceptible to sterilization or inactivation by the same technologies used on Earth, because carbon-based Martian life would likely use similar classes of covalent and hydrogen bonds for structural integrity and information storage and retrieval. If it is not carbon-based (e.g. silicon-based), but does use similar classes of covalent and hydrogen bonds for structural integrity, again, Earth-based sterilization/inactivation processes may be sufficient to disrupt molecular structures. If Martian life is another sort of highly robust, exotic type of life – which was not a focus of this set of SWG workshops – it becomes more difficult to predict what a sterilization/inactivation process would look like. This situation raises a critical point regarding placing focus on fundamental chemistries. The SWG felt it reasonable to assume that fundamental chemistries (e.g. covalent and ionic bonds between known molecules in the periodic table) would be present on Mars as they are on Earth. This concept of fundamental chemistries is discussed in more detail subsequently in this paper.
If it can be considered a reasonable model that life on Earth and Mars use similar biochemistries (i.e. nucleic acids, lipids, proteins), then life on Mars would be expected to have specific environmental requirements and similar susceptibilities to biochemical inactivation. Although, life on Earth is enormously diversified into billions of species, these entire cell-based organisms share the common set of 500 genes previously mentioned that are the signatures of life on Earth. Sterilization technologies are extremely effective at inactivating all known pathogens on Earth, including cellular- and non-cellular-based forms, because they share fundamental chemistry. Their mechanisms of action are also known to directly impact chemical bonds in essential structures for life including nucleic acid, protein and lipid structures (McDonnell Reference McDonnell2017). If we then assume that Earth and Mars life follow the same rules due to similar genetic components, then sterilization protocols used on Earth life are likely to work on Martian life.
However, even if such life on Mars is abundant with compatible biochemistry (such as being nucleic acid-based), the likelihood of it being directly pathogenic to humans (or other hosts) is considered very low. Most microorganisms on Earth are not pathogenic, nor harmful, and their abilities to infect host tissues are due to their coevolution between pathogen and host genomes. Microorganisms are usually highly adapted to specific biological niches or hosts, and even when novel pathogenicity arises, as in zoonosis or opportunistic infections, it does not represent a major evolutionary gulf. Emerging human pathogens are often the result of zoonosis in which an existing pathogen moves between related species being modified during this transfer such as coronaviruses, Ebola or HIV which all emerged from other mammalian hosts, or influenza which can transmit from avian or mammalian hosts. Existing microorganisms that coexist with humans over long periods of time can also cause new diseases when the organism takes on new pathogenicity, such as the Escherichia coli strain 0157:H7 that acquired a gene for Shiga toxin, or opportunistically infect a host with a weakened or compromised immune system such as candidiasis yeast infections or Kaposi's sarcoma, a cancer caused by a virus. Certain vector-borne diseases and parasites that have complex life cycles can transmit between disparate parts of the animal kingdom, such as between mosquitos and humans for malaria or yellow fever, or between snails and humans for the trematodes that cause schistosomiasis, with mosquitos and snails sharing a common ancestor with humans an estimated 600–1200 million years ago (Erwin and Davidson Reference Erwin and Davidson2002). Since any putative Martian microorganism would not have experienced long-term evolutionary contact with humans (or other Earth host), the presence of a direct pathogen on Mars is likely to have a near-zero probability.
Other biological risks beyond self-reproducing microorganisms, such as prions, were also considered in the SWG discussions. For example, prions are self-propagating, misfolded proteins with the ability to refold monomeric, analogous proteins that are benign and soluble into insoluble, abnormal, detergent and protease -resistant aggregations that cause harm to biological systems without being classified as alive.
Although prions lack genetic material such as DNA and RNA, they are capable of Darwinian evolution. Like bacteria and viruses, prions have a similar process of mutation and adaptive change (Li et al. Reference Li, Browning, Mahal, Oelschlegel and Weissmann2010). Prions are capable of interspecies transmission (Fernández-Borges et al. Reference Fernández-Borges, Parra, Vidal, Eraña, Sánchez-Martín, de Castro, Elezgarai, Pumarola, Mayoral and Castilla2017) between mammals, mutation development, and through natural selection, the mutations can result in evolutionary adaptations. Prion mutations are linked to changes in prion folding, a process also referred to as conformational change. Mammalian prions adopt several conformations, with each conformation (or strain) capable of precipitating a particular disease; moreover, each specific strain is thought to exhibit distinctive biochemical properties. Some prion strains maintain their unique biochemical signatures, as well as clinical and neuropathological signs, upon transmission to new hosts (Solforosi et al. Reference Solforosi, Milani, Mancini, Clementi and Burioni2013; Das and Zou Reference Das and Zou2016). It is also important to highlight that prions are completely dependent on their hosts for reproduction.
It is most likely that any hypothetical Martian prion or similar protein assembly (if such a prion exists and is present in the cached samples) would be incapable of propagation owing to the lack of available hosts, unless these protein assemblies were essentially similar to human, animal, plant or other Earth analogues, and due to the presence of differing environmental conditions on Earth. Protein folding and functionality depend on temperature, water availability and an appropriate milieus. The conditions on Mars are arid and would not likely promote interaction or propagation of native hypothetical protein assemblies in Earth hosts. Although there is evidence of water vapour in the atmosphere of Mars and past evidence of water activity found in the environment, the low amount of water would likely be insufficient for sustaining such protein functionality.
However, the danger to Earth is not just pathogens. The mandate to ‘…avoid…adverse changes in the environment of the Earth…’ (United Nations 1967) calls into consideration all parts of the Earth's biosphere. The possibility of Martian material disrupting any aspect of the Earth's ecosystem also must be addressed. For example, could Martian material somehow interfere with the ability of photosynthetic bacteria in Earth's ocean to fix carbon dioxide and produce oxygen? Photosynthetic bacteria such as Prochlorococcus are among the most abundant organisms on Earth and intensely important for the health of oxygen-respiring organisms, such as humans and animals. Damage to the ecosystem could be caused by direct cellular infections (i.e. pathogenesis; unlikely for the reasons outlined above), competition for resources, production of biotoxic metabolites or even displacement of organisms, as has been observed with many invasive species of plants, insects and other organisms between regions on Earth. Planetary protection must consider not just human health directly, but the entire biota of Earth.
Organisms evolve to live in a particular environment, and while some are generalists, others can only survive in very specific conditions. In all cases, organisms do not replicate if critical nutritional or environmental conditions are lacking. Invasive species or pathogens have been successful on Earth because they were adapted to similar environmental niches in their original and dispersed ecosystems (Bleuven and Landry Reference Bleuven and Landry2016). There are many described extremophiles that may survive in environments that are extreme to human or animal life (e.g. extremes of temperature or pressure) but do not survive under conditions in our normal habitat (Merino et al. Reference Merino, Aronson, Bojanova, Feyhl-Buska, Wong, Zhang and Giovannelli2019). Thus, it is plausible that any Martian microbe, after it arrives on Earth, would not be viable on Earth due to a lack of its required Martian nutritional and environmental conditions. Indeed, the Martian environment is inhospitable to Earth life, but conversely, the Earth environment is likely to be just as inhospitable to Martian life. Based on these factors, a very low qualitative probability of biological risk can be assumed.
Conclusions: Hazard Potential of Mars Biology
Based on what is understood about Earth and Mars biology, the SWG discussed what could be specifically said about the likely presence of bacteria, fungi, viruses, prions, toxins, etc., on Mars and whether they could be hazardous to Earth's biosphere. This was discussed in detail at all three SWG workshops with many diverse opinions offered. However, the arguments above for the ‘very low’ likelihood of Mars microbiota to cause harm were agreed to be fundamentally specious arguments (using the nomenclature of formal logical arguments (Gula Reference Gula2002; Engel Reference Engel1982)). As such, all such arguments must be viewed and used with caution as they are hypothetical by their very nature. However, the consensus conclusions of the SWG are as follows:
(1) There are substantial data to support that there is not advanced life (large multi-cellular organisms) that we know of on the surface of Mars. Thus, the focus is on single-celled organisms and biological molecules.
(2) Additional data support that Martian material has already been transferred to Earth by natural mechanisms with no apparent adverse effects.
(3) Earth and Mars life are plausibly similar in biological commonalities, because they are likely to utilize similar fundamental chemistries, and thus, be ‘playing by the same rules’.
(4) Scientific understanding of inactivation is likely applicable to Martian life, especially if the focus is on the fundamental chemistries when determining passive and active inactivation.
(5) Any form of life on Mars is unlikely to be hazardous to Earth's biosphere or humans; however, without data the risk is not zero.
(6) Due to the absence of zero risk, it is important to address containment of the Martian material and inactivation of potential material on the spacecraft surfaces where it may be needed.
Mechanisms of contamination
In order to understand and engineer the efficacy of any process that will be considered for the inactivation of Martian biological material, we must first understand the mechanism(s) through which uncontained Martian material might be present prior to being safely contained in a research facility. Based on the proposed architectural design of the MSR campaign, the most probable source of contamination would be dust particles on or around the OS carried from the surface of the planet that could hypothetically harbour biological material. The quantity of biological material that could theoretically be attached to a dust particle and the number of Martian dust particles adhering to or travelling with the OS are key to assessing the potential for contamination.
Martian dust and its potential transport to Earth
A thorough understanding of Martian dust and its transport physics is essential to understand the potential biology that could be associated with these particles. Martian dust can originate from various sources on the surface of Mars (Fig. 2): Dust particles can be suspended or wind-carried in the atmosphere; these particles have the potential to fall out onto exposed spacecraft surfaces. On the surface of Mars, dust particles can be found in undisturbed or perturbed regolith such as excavated material. This material can be moved onto exposed spacecraft surfaces during sample tube loading.
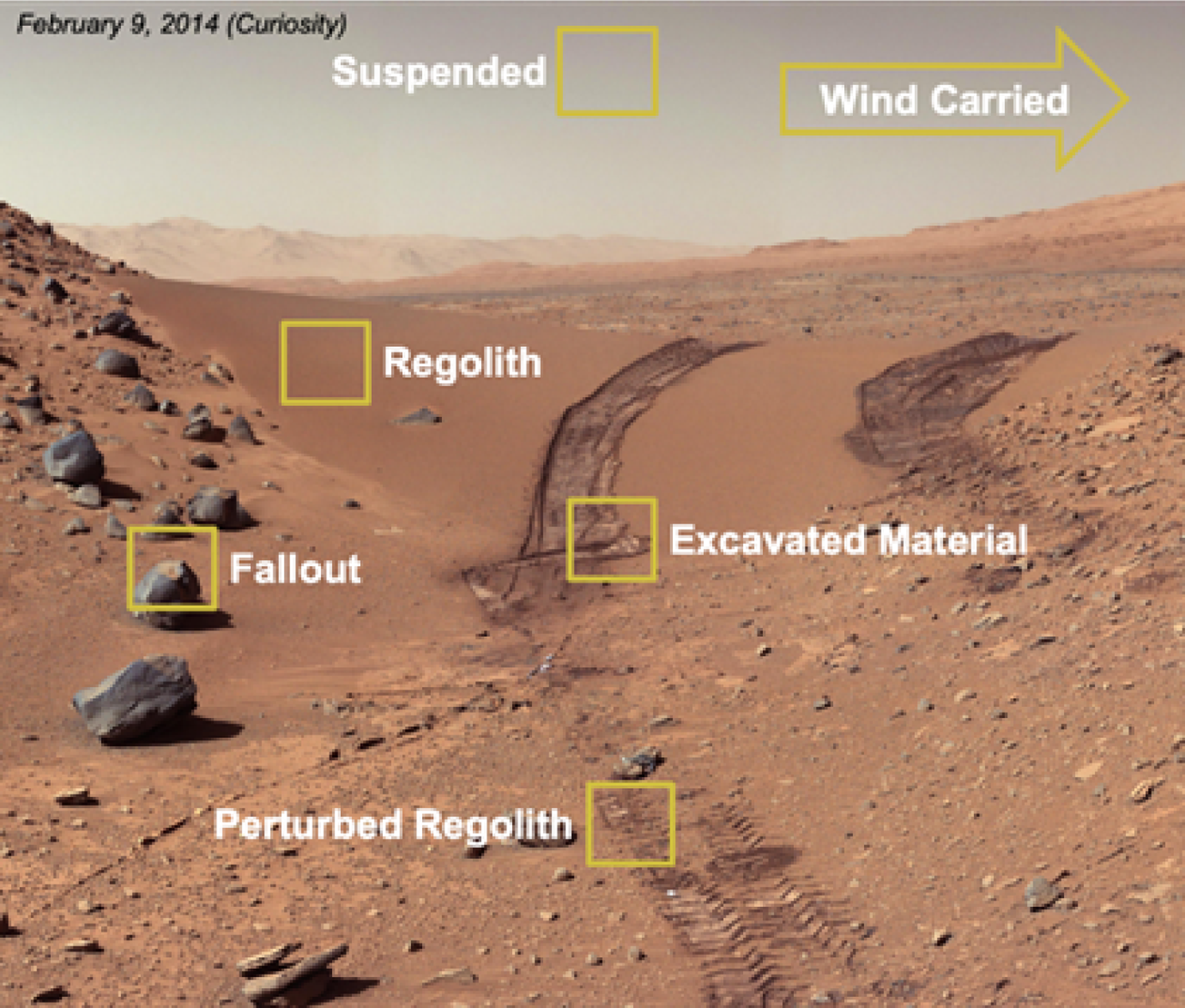
Fig. 2. The Mars material of concern – dust is depicted in each yellow highlighted area.
There are now several models in the literature that describe the size distribution and number density of suspended particles in the Martian atmosphere that depend, among others, on location, season and optical depth. Under nominal conditions, a commonly used Martian dust particle distribution is that described by Clancy and Lee (Reference Clancy and Lee1991), which is based on analyses of data from Mariner 9 and Viking. It shows that approximately 95.95% of all suspended particles have a diameter less than 15 micrometers. Data from the Pathfinder and Mars Exploration Rovers (MER) missions shed light on particle accumulation rates. For example, Mars Pathfinder carried a dedicated instrument (Materials Adherence Experiment – MAE) to measure dust deposition which identified the fractional coverage from dust deposition as 0.3–0.4% per sol (Landis and Jenkins Reference Landis and Jenkins2000).
For example, Mars material can accumulate inside a spacecraft or adhere to external surfaces, all of which enable the Martian dust to potentially hitch a ride back to Earth. Particle transport vectors have been derived to track Martian dust particles of concern from the surface of Mars to the surface of Earth. These vectors describe sources and sinks of dust and the process(es) by which it may be transported throughout the journey from Mars to Earth across different environments such as the Martian surface, launch from the surface, achieving Mars orbit, interplanetary transport to Earth, and Earth-reentry and landing. Each environment will expose Martian dust to different conditions. The launch from the Martian surface can strip and heat particles adhered to the external surface of the launch vehicle, and once in orbit, dust particles can be emitted from external spacecraft surfaces. Mars orbit and the interplanetary transfer to Earth exposes particles on the external surface of the spacecraft to solar UV radiation, solar heating, solar ionizing radiation, galactic cosmic rays, extreme temperature (e.g. high, low and fluxes possible), space vacuum and interactive effects of these factors. All of these processes are being studied by the MSR Campaign
An analog of the Martian environment on Earth: the Atacama Desert
An initial dust bioburden estimate is an essential input parameter in developing a microbial reduction protocol. Such a protocol establishes an appropriate verification and validation programme for a given microbial reduction process and allows the user to incorporate the data into a probabilistic risk assessment or other analyses, that may be part of the design and verification processes used by the MSR Campaign. Unfortunately, a Martian bioburden model does not exist, thus an analogue is necessary to satisfy this input parameter. To establish a working model of the BPP potential risk for contamination, biological performance of a proposed Martian bioburden model was developed based on Earth-based analogue environments. The analogue environments focussed on soil and dust vectors since the plausible potential contamination of the return sample would be either from the top layers of the Martian soil, from sampling or surface exposure, or dust in the Martian aeolian environment.
In developing a bioburden model, physics-based, Earth-based, organic-rich maximum bioloads and Earth-based environmental bounding cases were considered. As a worst-case scenario, a physical model could be employed where every particle would be completely comprised of biological material. In this case, the direct volume of dust could be converted into the maximum packaging of biologicals (e.g. cells, viral or proteinaceous particles) based on morphology and their associated calculated volumes. As an example, a spore model could be utilized as depicted in the following equation (Kesavan et al. Reference Kesavan, Schepers, Bottiger and Edmonds2014):
$\rm {{Total\, \, Spore = \lpar Cluster\, \, Diameter\comma\; }\, {m}\rpar ^3}\times {1.2}$
This is considered a worst-case scenario as it would be very unlikely that 100% of the material in any given environment would be biological. Earth-based environments that were organically rich were discussed, but these cases were deemed even more unrealistic since these conditions are not observed in Mars-based science results (Rummel et al. Reference Rummel, Beaty, Jones, Bakermans, Barlow, Boston, Chevrier, Clark, de Vera, Gough, Hallsworth, Head, Hipkin, Kieft, McEwen, Mellon, Mikucki, Nicholson, Omelon, Peterson, Roden, Lollar, Tanaka, Viola and Wray2014). Alternatively, Mars environmental parameters such as desiccation, UV radiation, salinity and temperature were factors used to establish a relevant Earth-based analogue.
To help frame and define this as a worst-case scenario, a biologically driven environment was evaluated to understand the maximum amount of bio-loading based on biotic processes in an Earth organic-rich environment. Organically rich environments that were considered focussed on Earth-soil or dust from agricultural soils. Note that saturated aqueous environments, such as swamps or lake beds, were not considered due to the desiccated nature of the plausible Mars biological sources. The observed ranges of bioloads observed are 108–109 cells g−1 representing 0.01–0.1% of bioparticle loading, assuming the average bacterial cell is 10−12 g (Frossard et al. Reference Frossard, Hammes and Gessner2016). The >99% of non-biological particles directly demonstrates the worst-case end-member of the physics-based model. While a directed Earth-based biological model defines the possibility of biologicals that can be supported on a substrate, it does not consider all the environmental parameters observed on Mars.
When the Mars environmental parameters were assessed, desiccation, salinity, temperature flux and solar UV radiation were the driving conditions noted that could significantly impact microbial survival on Mars. As such, Earth-based environments that exhibited these extremes include the Antarctic Dry Valleys, the Gobi Desert, Sahara Desert, aeolian dust transport phenomena (e.g. Asian and Saharan) and the Atacama Desert. Of all the sites, the most extreme and largest of astrobiological relevance in terms of study and abundance of peer-reviewed data is the Atacama Desert. Culture and non-culture-based cellular enumeration methods are published that evaluated diverse field sites of the Atacama (Navarro-González et al. Reference Navarro-González, Rainey, Molina, Bagaley, Hollen, de la Rosa, Small, Quinn, Grunthaner, Cáceres, Gomez-Silva and McKay2003; Glavin et al. Reference Glavin, Cleaves, Schubert, Aubrey and Bada2004; Drees et al. Reference Drees, Neilson, Betancourt, Quade, Henderson, Pryor and Maier2006; Connon et al. Reference Connon, Lester, Shafaat, Obenhuber and Ponce2007; Lester et al. Reference Lester, Satomi and Ponce2007; Ewing et al. Reference Ewing, Macalady, Warren-Rhodes, McKay and Amundson2008; Lynch et al. Reference Lynch, King, Farìas, Sowell, Vitry and Schmidt2012; Crits-Christoph et al. Reference Crits-Christoph, Robinson, Barnum, Fricke, Davila, Jedynak, McKay and DiRuggiero2013; Idris et al. Reference Idris, Goodfellow, Sanderson, Asenjo and Bull2017; Schulze-Makuch et al. Reference Schulze-Makuch, Wagner, Kounaves, Mangelsdorf, Devine, Vera, Schmitt-Kopplin, Grossart, Parro, Kaupenjohann, Galy, Schneider, Airo, Frösler, Davila, Arens, Cáceres, Cornejo, Carrizo, Dartnell, DiRuggiero, Flury, Ganzert, Gessner, Grathwohl, Guan, Heinz, Hess, Keppler, Maus, McKay, Meckenstock, Montgomery, Oberlin, Probst, Sáenz, Sattler, Schirmack, Sephton, Schloter, Uhl, Valenzuela, Vestergaard, Wörmer and Zamorano2018; Ruginescu et al. Reference Ruginescu, Purcǎrea, Dorador, Lavin, Cojoc, Neagu, Lucaci and Enache2019) and include culture methods (e.g. tryptic soy agar, R2A, plate count agar) to biochemical (e.g. sublimation, phospholipid-derived fatty acids and adenosine triphosphate), direct microscopy and molecular-based techniques (e.g. quantitative polymerase chain reaction). The values of these aforementioned parameters can be utilized to construct a biological cell per gram of soil model to account for multiple variables in the Atacama that include sample depth, rainfall and total carbon-based extrapolations.
Thus, we propose that the hyper-arid regions of the Atacama Desert (e.g. portions of the Yungay) is the most aligned Earth-based extreme environment compared to Mars environmental parameters that have been extensively studied as an astrobiology field site. Importantly, the environmental perturbations on Mars are harsher for microbial life in terms of larger temperature fluxes, lower water availability, more intense solar UVC radiation and the associated growth on highly oxidized substrates. Although there are large differences in environmental conditions in the Atacama Desert compared to the Martian surface, the Atacama remains the most representative Earth analogue that might help establish an upper bound of possible biological limits. Such an Atacama-based model could then be used as a starting point in the design of the MSR campaign's sterilization programme. While the model could provide a cells per gram of soil distribution model, further development and discussion is required into the applicability and utilization of this model as a contamination vector. In particular, the distribution and association of biological material need to be better understood in order to inform the particle-dust analysis and biological models. Aeolian dust analyses have been conducted to evaluate the particulate versus biological-associated particles that can be used. Alternatively, biological particulate loading experiments can be conducted or data can be utilized from aerobiology experiments in Earth's atmosphere to understand particle formation and the fates and transfer processes on Earth for extrapolation to the Mars environment.
Conclusions: Mechanisms of Contamination
The consensus conclusions of the SWG are as follows:
(1) The dust transport model discussed was a reasonable approach to assessing contamination.
(2) It is important to understand the number and type of particles that could adhere to the OS during loading of the sample tubes and potentially transfer to the MAV system.
(3) It seems reasonable that the Atacama Desert can act as an analogue, although there are several unverified conditions that should be addressed.
(4) Estimating proteins in these desert environments would be difficult, so focussing on amino acids production could be revealing.
(5) Amino acids that have been found in some meteorites do not form complex structures, which leads one to believe that there are still external processes which ultimately lead to the formation of harmful proteins.
Future Work: Mechanisms of Contamination
The consensus of future work suggested by the SWG is as follows:
1. With additional refinement of input parameters and subsequent studies, an improved dust model may be able to adequately predict Martian dust particle quantity, transport and potential sterilization through active and passive ways.
2. The Atacama is distinct in that its resident extremophiles have developed molecular mechanisms for adaptability in these harsh conditions (i.e. low water activity), but we do not fully understand the environmental pressures which can lead to these survival mutations. If the Martian environment is not accommodating for active metabolism, due to a lack of nutrients, additional studies are needed to understand adaptive processes of extremophiles.
3. Prions are biological molecules that do not require metabolic pathways for survival, so additional data are required to determine if the Atacama can be used for predictive analysis.
4. Prions consist of less than 100 amino acids, and there are still knowledge gaps on understanding if de novo synthesis of amino acids can form random β pleated sheets, a hallmark structure for prion pathology.
5. It would be very difficult to model protein formation in the Atacama Desert; however, additional inputs related to biology in these environments will continue to shed light on these knowledge gaps and understand microbial bioburdens in extreme environments like the Atacama.
Sterilization concepts
The term sterilization is generally used to describe the ‘killing’ of viable microorganisms. In the course of the SWG meetings, it was determined that this term would not cover all aspects of potential Martian material to be addressed as part of MSR. Thus, the term ‘inactivation’ is being used in many places throughout this paper to address reduction of both the viable and the non-viable biological materials. This section provides the sterilization concepts related to inactivation of viable microorganisms to provide background for subsequent information in this paper as well as for future work.
It is important to reiterate here that sterilization/inactivation considered by the SWG only needs to be applied to Martian material that has not been suitably contained during the BTC process in-flight. The integrity of the collected encapsulated samples must be kept pristine for astrobiological and geological relevance. Thus, although the information below discusses gathering data and performing validation with very high initial counts of biological material, such as 1 million bacterial spores, the quantity of uncontained Martian material in the robust MSR containment system being planned should be orders of magnitude lower, as described elsewhere in this paper.
The term sterile (or also sterility) is simply defined as being free from viable microorganisms. Sterilization is a process used to render product free from viable microorganisms (and therefore render it sterile). The term sterilization is commonly used in the context of the safety of health care products. Sterilization is a quantifiable process that delivers a defined sterility assurance level (SAL) or the probability of a single viable microorganism occurring on an item after sterilization. The most commonly used SAL is a one in one-million chance of a viable microorganism surviving on a product after sterilization, which is referred to as a SAL of 10−6 (International Organization for Standardization 2017a, 2017b). This is the level that has been accepted globally by the health care industry for products that come into contact with compromised human tissue, for example, items to be injected or implanted inside the body. Although on Earth these processes are usually performed in specialized, dedicated chambers, the critical aspects generally are exposure to the sterilant in the proper environment for a minimum amount of time.
The industry standard approach to estimating the SAL for most sterilization methods is to begin with a known population (typically 1 × 106) of resistant microbes (e.g. spores of Bacillus spp.), apply a sterilizing treatment in which no microbial survivors are detected after a specific time interval, and then doubling this treatment time to achieve a total of 1012 bioburden reduction. The SWG concurred with using this general approach for predicting the inactivation of a putative Mars microbiota during MSR.
There are several forms of sterilization that are routinely used on Earth, and many more that are in various stages of development and commercialization. In order to merit the term ‘sterilization’ in the healthcare industry, substantial data on the process must be gathered to first establish the broad spectrum efficacy against a range of microorganisms, and then to demonstrate a consistent (most commonly, log linear) rate of inactivation (International Organization for Standardization 2009). Thus, when the term sterilization is used for processes on Earth, it is understood to be effective on all forms of life. If a process is only proven effective against certain forms of life, for example, it is effective against vegetative bacteria but not against spores, the process is considered a disinfectant rather than a sterilant. Sterilization methods in the context of microbial inactivation include the following:
Ethylene oxide (EO): EO is a gaseous chemical sterilant. The EO molecule is a simple epoxide ring with strained bond angles which makes it exceptionally reactive. The typical parameters for EO sterilization (including common ranges) are: exposure time (2–8 h), temperature (50–55°C), gas concentration (450–800 mg l−1) and relative humidity (40–80%). EO is considered an alkylating agent, and therefore, the mode of microbial inactivation is the result of alkylation of proteins, DNA and RNA. Alkylation reactions substitute a hydrogen atom from the cellular structure with an alkyl group inhibiting normal microbial structure, function and replication.
Ionizing radiation: Ionizing radiation is a penetrating sterilization process that is designed to expose products or materials to a known source of radiation for a given amount of time. The most common source for sterilizing radiation is γ radiation from cobalt-60. The γ radiation can break chemical bonds, forming free radicals, which can damage DNA or other complex molecules critical for reproduction. Other ionizing radiation sources include electron beams and x-rays. The control parameters for radiation processes are the times of exposure to the radiation fields coupled with its specific dose intensities.
Ultraviolet radiation: Sterilization by ultraviolet (UV) radiation is effective at killing surface microorganisms though it is neither ionizing nor penetrating. Short wavelength UV radiation (UVC, 100–280 nm) is known to kill microoragnisms by disrupting their nucleic acids, by breaking chemical bonds, and creating new ones; but it has also been shown to have an effect on the molecular structure of proteins and lipids (Santos et al. Reference Santos, Moreirinha, Lopes, Esteves, Henriques, Almeida, Domingues, Delgadillo, Correia and Cunha2013).
Vapour hydrogen peroxide (VHP): Vapour hydrogen peroxide has been used to sterilize space flight hardware not compatible with high-temperature sterilization methods. As a vapour, the chemical can penetrate irregular surfaces, and when properly vented, does not lead to condensation of water on surfaces. The hydrogen peroxide inactivates microorganisms through oxidation reactions and is active against a wide range of biomolecules, including proteins, nucleic acids and lipids (Finnegan et al. Reference Finnegan, Linley, Denyer, McDonnell, Simons and Maillard2010).
Moist heat (steam): Moist heat sterilization uses pressure in a chamber to drive steam temperatures up to where inactivation of microorganisms occurs quickly. Temperatures such as 121 or 132°C are common. Once materials in the sterilization chamber reach the desired temperature and pressure, it is held for a period of time, often 15 and 4 min, respectively. The critical parameters for moist heat sterilization are temperature and time in the presence of moisture, most commonly saturated steam. The mode of microbial inactivation is generally understood to be denaturation of essential macromolecules such as lipids, nucleic acids, enzymes and structural proteins through the transfer of energy from the saturated steam molecule to these structures.
Dry heat: The mechanism of spore inactivation by dry heat is likely to be DNA damage (Setlow Reference Setlow2006, Reference Setlow, Driks and Eichenberger2016). Dry heat uses elevated temperatures except that with dry heat, a combination of steam and pressure is not used to heat the products; rather heat is delivered via a number of methods including convection, conduction, infrared radiation, heated forced air, heated inert gases or incineration. Typical temperatures for dry heat sterilization are around 160°C and higher for a number of hours, but lower temperatures such as 125°C are also used with extended exposure times.
Other gaseous sterilization methods (hydrogen peroxide, gas plasmas, nitrogen dioxide, chlorine dioxide, peracetic acid, ozone, etc.): These methods use a microbicidal chemical, usually in a vapourous or gaseous phase, and rely on parameters such as temperature, gas concentration and pressure; and involve a contact time with the surfaces of products. Some of these chemical sterilants do not have an extensive use in industry compared to the other methods, so there is often additional work to be done as part of a regulatory submission to demonstrate that the process is safe and effective for a particular application. More information on the use and compatibility of the more widely used sterilization modalities in the healthcare industry can be found in various ISO and United States Pharmacopeia (USP) standards and AAMI TIR 17 (Association for the Advancement of Medical Instrumentation 2017).
Validation of sterilization processes
Sterilization processes are established by performing validation exercises on the equipment and products to be sterilized. There are two primary sterilization validation approaches that exist in industry: an overkill-based method (typically based on using a biological indicator (BI)) and bioburden-based methods
As briefly discussed above, overkill-based methods often use a microorganism that has previously been demonstrated to be highly resistant to a given sterilization process in order to test the efficacy of that process. This efficacy is demonstrated by starting with a known, high count of the test microorganisms (e.g. 106) and determining the degree of inactivation or number of log reductions that occur during a portion of a proposed sterilization cycle. The microorganisms selected tend to be bacterial endospores (e.g. Bacillus spp.), as these typically have high levels of resistance to inactivation (in comparison to other microorganisms). Endospores (henceforth just spores) are also resistant to desiccation and can therefore be made into BIs by inoculating a carrier (e.g. paper, metal) that is stored inside a package that is permeable to the sterilization process. A difficult to sterilize location on the product is determined and can then be challenged by either placing the BI in that location, or if the product location is too small to fit a BI, directly inoculating that location with a liquid suspension of the spores. The exposure time that inactivates all of the spores is called a fractional cycle, for example, a half-cycle exposure. The successful fractional cycle time is then extrapolated (often doubled) to determine the minimum exposure time that will be applied to the products to ensure sterilization. This method is referred to as an ‘overkill’ method, as it is considered a significant overkill based on the assumed starting population and resistance of microorganisms that may be present. Overkill-based methods are traditionally employed for ethylene oxide (EO), dry heat, moist heat (steam) and other gaseous sterilization methods such as hydrogen peroxide, nitrogen dioxide, chlorine dioxide, ozone and others.
Bioburden-based methods by contrast require a more detailed knowledge of the naturally-occurring bioburden (the population of viable or detectable microorganisms) on the product. The resistance of this bioburden and the extent of the sterilization cycle required are then determined in one of two ways:
Option 1: Using a sterilization table to determine the sterilization cycle based on the product's bioburden count. The table provides a fractional sterilization cycle that is applied and followed by a sterility test. If the sterility test gives acceptable results, the sterilization table provides the complete sterilization cycle to be applied to the product.
Option 2: Determining the sterilization cycle using an incremental series of sterilization exposures followed by sterility tests. The results of the incremental sterilization exposures and sterility tests are used to calculate the complete sterilization cycle to be applied to the product.
Both bioburden-based options provide the ability to quantify the required SAL. Bioburden-based methods are most commonly applied only to radiation sterilization, but may also be applied to other processes. Although bioburden-based methods may not include the same level of overkill as the overkill-based methods, they both are considered equally safe regarding potential impacts to a patient. Combinations of these methods can also be considered as validation approaches.
Overall, both overkill- and bioburden-based methods of sterilization validation require some estimation of the types, levels and resistance of bioburdens that may be found on products. For samples coming from Mars, assumptions based on data will need to be made about potential types of viable Martian material, their quantities and the extent of the inactivation processes in order to assign a safety margin to the collection of sterilization procedures.
Sterilization based on fundamental chemistries of life
It is possible to imagine unique and putative harmful agents on Mars that are both resistant to all forms of inactivation and that are pathogenic or hazardous to life on Earth. However, all available knowledge on the topic, including the previous portions of this paper, point to this potential scenario being qualitatively highly improbable. To date, all available knowledge indicates that the fundamental chemistries of life that occur on Earth (e.g. covalent, ionic and hydrogen bonds between molecules) are also in place on Mars. There is no evidence of indigenous, advanced life on Mars, despite many orbiting and surface missions to the planet, but it is not clear if microbial life or chemical biomarkers are present. As we assess the potential hazards of Martian life (if present) to terrestrial Earth life, it was agreed upon by the SWG that focussing on inactivation of the fundamental chemistries is appropriate.
Earth biological challenge agents can be used to validate the efficacy of sterilization and inactivation modalities. However, emphasis should also be placed on the modes of action of these technologies and particularly their effects on the fundamental chemical properties (e.g. the assembly and function of the macromolecules that make up the various forms of known life). For example, although it might not make sense to focus all sterilization efforts specifically on DNA or RNA inactivation or damage, as those configurations may not be found on the Martian surface; it is sensible to focus on nucleic acids in general and the bonds that hold them together and how they might be disrupted. Thus, the term nucleic acid is used in this paper to represent DNA/RNA-type materials and the inactivation that occurs to those materials. Likewise, it is not reasonable to focus other efforts specifically on the prions that cause transmissible spongiform encephalopathies such as Creutzfeldt-Jakob Disease in humans (as they are entirely based on misfolded human or animal proteins), but it is reasonable to focus on inactivation methods that affect protein assemblies similar to prions and the means of fully denaturing (inactivating) those protein polypeptides. Thus, the term protein assembly is used in this paper to represent prion-type materials and the inactivation that occurs to those materials. For nucleic acids, the primary bonds in place are covalent (between the bases, sugars and phosphate groups of each strand) and hydrogen (between the two strands). For prion-type protein assemblies, the primary bonds in place are peptide, hydrogen and disulfide bonds. Additionally, there are interactions involved with protein folding that include hydrophobic and electrostatic interactions as well as van der Waals forces involved in the polypeptide main chain and amino acid residues to create a given secondary assembly that is associated with the transmissible and disease-causing form of the protein. If inactivation processes engineered into the MSR system can disrupt those types of bonds and interactions, the SWG agreed that it should be sufficient to disrupt those same bonds between other, similar chemistries potentially found in Martian material. Therefore, much of the general focus should be on inactivation of the fundamental chemical bonds of nucleic acid and protein assemblies.
The additional types of biological challenge agents that are intrinsic in the basic requirements for sterilization methods are viruses. Viruses can range in resistance to inactivation, with non-enveloped viruses well established to be the most resistant forms (Eterpi et al. Reference Eterpi, McDonnell and Thomas2009) but less resistant than other forms of microorganisms such as bacterial spores (McDonnell Reference McDonnell2017). It is well understood that with some methods of inactivation, such as chemical or heat, bacteria that form dormant spores, such as Bacillus or Clostridium species, represent a much greater challenge to inactivation than viruses, whether the viruses are enveloped or non-enveloped viruses or bacteriophages (U.S. Food and Drug Administration 2020). For radiation-based inactivation methods including UV (Rockey et al. Reference Rockey, Young, Kohn, Pecson, Wobus, Raskin and Wigginton2020), as the main mechanism of action is known to be DNA or RNA damage, protein structures and prions lack associated DNA and RNA molecules, and therefore prions could present the greatest inactivation challenge.
When considering appropriate Earth analogues for determining inactivation protocols, it must be addressed from two different perspectives:
(1) Nucleic acid based: spores of bacteria that are resistant to chemical- or heat-based inactivation methods can be prioritized as BIs as they represent a more difficult class of organism to inactivate compared to other types of Earth microorganisms and viruses.
(2) Protein based: prion-type proteins are naturally resistant to heat-based inactivation methods and can be prioritized as general-process indicators as they represent a more difficult class of material to inactivate compared to viable microorganisms.
Concept of microbiological safety
The United States Food and Drug Administration (US FDA), and similar regulatory agencies worldwide, require health care manufacturers (e.g. medical device and pharmaceutical manufacturers) to demonstrate that their products are safe and effective. The level of safety with health care products is rarely, if ever, so high that the potential risk to a patient is absolutely zero. Nevertheless, if adequate controls are maintained and monitored for effectiveness, the level of risk to patients can be reduced to acceptable levels.
As discussed earlier, in the application of sterilization processes, the concept of safety is largely addressed by applying a SAL to the diverse processes. In some instances, different levels of sterility assurance other than 10−6 are permissible based on an assessment of risk. For example, a SAL of 10−3, which corresponds to a one in 1000 probability of a viable microorganism being present, is appropriate, such as traditionally in the USA for sterilization of drapes to cover equipment in an operating room during surgery. Essentially, these and other SALs can be shown to be accepted based on a risk assessment and remembering that the definition of ‘sterile’ is being free of living microorganisms and not necessarily a theoretical mathematical extrapolation. Additionally, documents are available (International Organization for Standardization 2017a, 2019) that provide the concepts for justification of alternative SALs based on a thorough risk assessment and the inability of products to withstand typical sterilization processes. Thus, in the healthcare industry, flexibility regarding the extent of sterilization is provided depending on the situation and the output of risk assessment. The MSR SWG has determined that this concept of microbiological ‘safety’ and use of risk assessment is reasonable and appropriate when discussing the level of inactivation required for uncontained material on spacecraft surfaces. As is the case with health care products, the potential risk to humans and planet Earth cannot be described as non-existent or zero. However, with a thorough assessment of what is known and through making reasonable assumptions based on Earth empirical data, an understanding of the potential risk can be determined and quantified.
Biological challenge agents
One of the SWG conclusions from discussions on Mars life was that it is reasonable to employ Earth-based surrogates to challenge potential inactivation processes. This is due, in part, to the possibility that a common ancestry between life of Mars and on Earth may be plausible, or at the very least that the planets use the same fundamental chemistries.
A combination of biological reduction steps including passive inactivation due to environmental conditions during space travel (e.g. solar ionizing radiation and UV, deep space vacuum, solar heating, etc.) and active inactivation spacecraft systems (e.g. chemicals, heat, spacecraft-induced UV radiation) should be considered to understand and reduce the viability of potentially uncontained Martian material through the entire mission architecture from Mars orbit to arrival on Earth. To validate these processes, a programme should be initiated to establish the biological challenges, coupons and test protocols for biological reduction assurance and to establish expected levels of inactivation (i.e. D-values, or the time or dose required to achieve inactivation of 90% of a population of the test microorganisms) and/or the impact of inactivation process variables (e.g. for heat inactivation, Z-values or the change in temperature of a thermal sterilization process that produces a tenfold change in D-values) for both single modalities as well as the additive impacts from multiple modalities. The standardization of a common test programme was considered paramount, as multiple labs could conduct testing and be used for third party verification.
A wide approach to gain expert input for selecting biological challenges was conducted, by surveying the scientific community for the largest spectrum of extreme environmental isolates to include bacterial spores, fungi, archaea, plasmids and prions. The preliminary polling consisted of environmental microbiology, astrobiology, planetary protection and sterilization experts from academia, industry and government labs worldwide. The polling identified 75 candidates, and 13 candidates were down selected to begin initial testing. The candidates that were considered consisted of preferential biosafety level 1 microorganisms that are relevant to spacecraft, the space environment, industrial standards, and that have growth conditions that favour standard microbiology lab resources (e.g. standard doubling times, temperatures, pressures, etc.). After discussing this approach with the SWG, further inputs into accessing the sterilization community was a resulting action. A subset of the 2019 Kilmer Conference attendees was polled. The approach was also presented at the US Environmental Protection Agency's 2019 International Decontamination Research and Development Conference (Benardini and Smith, Reference Benardini and Smith2019). Feedback from both of these communities was utilized. In the end, 39 labs were polled resulting in 101 candidates and 17 candidates were then down-selected (Table 1). Spore-forming bacteria of known high-level resistance to inactivation were selected to be the first candidates for testing due to their hardy nature, ease of growth and viability on test surfaces when desiccated to facilitate ease of shipping and testing.
Table 1. Proposed biological challenge agent list

Defined bacterial spore stocks and a standard set of test surfaces (coupons) were suggested for procurement so that all test laboratories can use the same standardized spore crop, starting population and associated coupon material of a known cleaning level and surface finish. The coupons will be seeded with a target population of approximately 2 × 106 spores and individually packaged for enumeration prior to and after each test procedure to ensure the target populations and viability of the spores on all coupons. Further protocols to prepare test coupons should also be explored for the non-spore-forming microorganisms as identified future work.
It is not clear that typical spore-forming microorganisms would provide sufficient assurance that an inactivation process will be effective without prior knowledge of the nature of life on Mars, if present. Challenge microorganisms or BIs traditionally used at NASA have been identified by past mission requirements that mainly focussed on spore-forming bacterial species. Prions have proved in the past to be highly resistant to traditional sterilization modalities such as EO and radiation that are usually sufficient for inactivating nucleic acid-containing agents (McDonnell and Comoy Reference McDonnell, Comoy and Walker2020; McDonnell Reference McDonnell and Walker2013a, Reference McDonnell, Fraise, Lambert and Maillard2013b). The SWG suggested that tests be performed to evaluate the effectiveness of dry heat on protein assemblies, while concurrent trade-off studies could be performed with other sterilization modalities.
Sup35, a non-pathogenic yeast strain from Saccharomyces cerevisiae, was identified (Table I) to have amyloid-based prions that can be used as an acceptable surrogate for inactivation testing (Chernoff Reference Chernoff2007). Yeast proteins have been successfully employed by researchers to understand fundamental prion properties such as aggregation and self-propagation (Chernova et al. Reference Chernova, Wilkinson and Chernoff2017). At a molecular level, the yeast prion protein selected for testing has been extensively characterized and exhibits aggregation behaviour similar to mammalian prion protein (PrPsc) (Chernova et al. Reference Chernova, Wilkinson and Chernoff2017; Wilson et al. Reference Wilson, Bommarius, Champion, Chernoff, Lynn, Paravastu, Liang, Hsieh and Heemstra2018). Yeast aggregating proteins, despite also being termed ‘prions’, are not known to be dangerous to humans, due to the species barrier, and can be used as a cost-effective and safer alternative to working with mammalian prions (Chernova et al. Reference Chernova, Chernoff and Wilkinson2019).
While there is much literature to suggest how bacterial spores are sterilized, there remains some knowledge gaps in the accurate molecular mechanisms of prion propagation and inactivation. The stabilization of the core assembly, as previously mentioned, is supported by hydrogen bonds and other interactions involving the polypeptide main chain and amino acid residues. Thus, inactivation data should be gathered regarding the prion protein's stability and disruption. Heat will likely increase the kinetic energy, causing molecules to vibrate so rapidly that the peptide bonds and non-polar hydrophobic interactions are disrupted in the process. Testing will require prion proteins to be aggregated and heat exposed, followed by standardized protein tests including sodium docecyl sulfate-polyacrylamide gel electrophoresis (SDS-PAGE) and Western blot analysis, which could be used to visualize the protein conformational changes based on the presence or absence of aggregated and monomeric fractions (Chernoff et al. Reference Chernoff, Uptain and Lindquist2002). Detection of conformational changes do not guarantee the absence of small fractions of the proteins retaining the active prion conformations, and thus, additional assays will likely be required to demonstrate complete degradation of the prion proteins into non-functional forms. A fully degraded/inactivated prion protein is unable to renature or restore pathogenicity by refolding. Thus, prion inactivation assays should be developed to determine if the treated aggregate samples with the proposed inactivation protocols have the ability to seed conformational changes and aggregation with the addition of fresh monomeric subunits. Additional yeast prion tests should be performed to understand the general biochemistry of how prions respond to stressors (i.e. heat, UV, vacuum) relevant to Mars samples, and which specific bonds are being disrupted as a result of the processes.
Test procedures for all biological challenge agents should include sterilization industry best practices such as relevant portions of the international standard series ISO 11138 (American National Standard Institute/Association for the Advancement of Medical Instrumentation 2019, 2017) ‘Sterilization of Health Care Products – Biological Indicators’ and should be performed on representative spacecraft materials. Test procedures should also include previous NASA methodologies to determine D-values using thermal spore exposure vessels (Kempf et al. Reference Kempf, Schubert and Beaudet2008; Schubert and Beaudet Reference Schubert and Beaudet2011) or equivalent. Multiple labs would then test representative BIs to establish inactivation values (D- and/or Z-values) by analysis of sterilization/inactivation curves.
Conclusions: Sterilization Concepts
The consensus conclusions of the SWG are as follows:
(1) The term ‘microbiological safety’ was determined to be the most appropriate because it includes a combination of multiple aspects, including sterilization, inactivation and risk assessments based on published data.
(2) Since the biological risk associated with putative Martian biology is not completely zero, an active inactivation process should be considered for inclusion into the campaign to further mitigate the risk of exposure from dust or other uncontained Martian materials.
(3) The SWG participants concurred on an initial set of potential candidates for selection as biological challenge agents, including bacteria, spore formers, fungi, archaea, viruses, plasmids and prions (Table I).
(4) Bacterial spores are considered as highly resistant challenges to test and optimize sterilization processes for proposed applications. The modes of action of these methods are also useful to understand how targets may also include macromolecules such as proteins and nucleic acids.
(5) Of those identified (Table I), prions seem to represent the hardiest challenge agent to inactivate, so selection of a modality that leads to degradation and denaturation of peptide bonds is necessary. This would result in not just unfolding of the prion structure but breaking the bonds that hold the prion components (amino acids) together.
Future Work: Sterilization Concepts
The consensus of future work suggested by the SWG is as follows:
(1) Since the potential risk for contaminating the Earth's biosphere cannot be predicted to be a zero risk, a thorough assessment of various passive and active inactivation modalities, combined with reasonable assumptions based on empirical data, can determine a level of risk or ‘sterilization assurance’ in the process.
(2) All available knowledge suggests that the fundamental chemistries of life that occur on Earth are most likely to exist on Mars as well. It was recommended to gather data on the ability of inactivation modalities to inactivate specific biological challenge agents. The data will be used to demonstrate the ability of those modalities to act on the fundamental chemistries present in the bonds that form the structures of nucleic acids, amino acids and proteins.
(3) For the spore stocks and prion surrogates, it is critical to have 3rd party vendors produce reagents after we identify a standard set of supplies so that all labs use a standardized stock or surrogate, starting populations, and associated coupon material cleaning and finish.
(4) Test procedures to be developed should include best practices such as relevant portions of ISO 11138 series, ‘Sterilization of Health Care Products – Biological Indicators.’
(5) Test procedures should also include previous NASA methods to determine D-values and/or Z-values using thermal spore exposure vessels and the testing should be performed across multiple labs.
Passive inactivation
Passive inactivation, for the purpose of this report, describes all of the inactivation processes that will act upon potential Martian material; processes that are inherent in the environments that will be encountered on the surface of Mars, to the OS orbit around Mars, the Mars–Earth cruise environment and reentry to Earth. These include solar UV radiation on the surface of Mars and in Mars orbit, solar heating and temperature cycling during ascent and orbit, removal of material during travel, vacuum, ionizing radiation and the synergistic biocidal effect of these factors in space.
UV radiation sterilization on Mars and during Mars sample return
By Earth's standards, Mars is a harsh and inhospitable environment. The Martian atmosphere is dominated by the presence of CO2, but also contains, in much lower amounts, O2, Ar, CO, N2 and H+ (Chevrefils et al. Reference Chevrefils, Caron, Wright, Sakamoto, Payment, Barbeau and Cairns2006). The Martian surface is highly oxidizing, oligotrophic, and is exposed to high levels of solar ultraviolet radiation (UV). The climate of Mars is cold, dry and Martian dust storms occur frequently that are immense in size and can be highly destructive. Although most Earth-borne microorganisms would perish quickly under these conditions, any putative Martian microorganism that exists at the surface would have either evolved the necessary environmental resistance mechanisms to adapt and survive close to the surface or would be surviving in deeper or more sheltered niches. While the entirety of our knowledge of biology is based on life as we know it on Earth, that knowledge can still yield critical insights into how potential extant Martian microbiota may live in such an inhospitable environment, and what challenges and risks it may pose by introducing such an organism into Earth's biosphere. If we can apply any of our knowledge of life as we know it, to life as it could have evolved on another planet such as Mars, then the SWG agreed we might reasonably make the argument that there could be organisms on, or under, the surface of Mars that have evolved adaptations to persist within its harsh, yet not necessarily inhospitable, UV-exposed environment.
Ultraviolet radiation is an effective microbial reduction modality. Few Earth microbes can withstand more than a few minutes of exposure of UVC (200–280 nm); however, some microbial organisms such as Bacillus pumilus SAFR-032 display an ability to withstand much higher doses of UVC (up to 4 kJ/m2) than any other organisms on Earth (Schuerger et al. Reference Schuerger, Richards, Newcombe and Venkateswaran2006; Osman et al. Reference Osman, Peeters, La Duc, Mancinelli, Ehrenfreund and Venkateswaran2008). UV-resistant microbes have developed evolutionary adaptations which allow them to withstand much higher levels of UV. Studies have shown that while initial exposures to UV can be effective in killing a high proportion of B. pumilus SAFR-032 within the exposed populations on spacecraft surfaces (Schuerger et al. Reference Schuerger, Richards, Newcombe and Venkateswaran2006), a small proportion of a population can survive in protected pits, crevices or shaded niches (Moores et al. Reference Moores, Smith, Tanner, Schuerger and Venkateswaran2007). This ability to survive seemingly lethal doses of UV exposures has been attributed to a number of unique physiological mechanisms relating to UV resistance such as those responsible for the repair of UV-damaged DNA (Chatterjee and Walker Reference Chatterjee and Walker2017).
Arguments have been made that a hypothetical Martian microbiota would be no more resistant to UV than an Earth microbe. However, this view does not fully encapsulate the questions surrounding the ability of UV to penetrate into the Martian subsurface, which is dependent on the radiation energy, the surface geochemistry and particle size distribution of the fines.
There is evidence that between 0.5 and 2 mm of contiguous dust is required to completely shield an organism from being inactivated by solar UV on Mars (Link et al. Reference Link, Sawyer, Venkateswaran and Nicholson2004; Schuerger et al. Reference Schuerger, Richards, Newcombe and Venkateswaran2006). At the microbial scale, there are almost an infinite number of niche environments on the surface of Mars which could provide a complete respite from solar UV. This shielding effect may also be applicable on the outside of spacecraft surfaces if an organism finds itself encapsulated or otherwise shielded within a thin layer of Martian dust. In this respect, UV could potentially reduce a bioload on spacecraft surfaces while still in the Martian atmosphere, but ultimately fall short of complete sterilization. The primary focus of the UV sterilization discussions during the SWG workshops was to present the current foundational knowledge relating to UV's potential to sterilize a hypothetical Martian organism.
The solar UV exposures may also affect the assembly and prion activity. Prions are known to be resistant to UVC (200–280 nm) as typically used on Earth for disinfection applications; however, it was highlighted at the workshops that broad-spectrum UV or other radiation exposures analogous to that on Mars have not been tested on protein assemblies such as prions. Since breaking peptide and hydrogen bonds as well as hydrophobic and electrostatic interactions and van der Waal forces is necessary for prion inactivation, further research is needed to determine UV susceptibility at wavelengths more appropriate to those bonds and forces. Understanding environmental conditions on Mars may provide insights to the challenges potential Martian protein assemblies may have to overcome to retain functionality similar to that observed on Earth.
At the first meeting in January 2019, two primary objectives regarding UV sterilization were presented to the members of the working group.
(a) To investigate the sterilization potential of Mars UV on dust that might accumulate on the exterior surfaces of MSR spacecraft.
(b) To investigate the sterilization potential of the Mars-to-Earth cruise environment on dust that might be present on exterior spacecraft surfaces.
The sterilization potential of Mars UV on dust
The absence of ozone in the Mars atmosphere results in a broader UVC spectrum at the Martian surface. Exposure to this solar radiation is from direct, diffuse and reflected UV radiation. There are a variety of factors to consider in order to understand the degree of exposure received by a given surface. Factors which influence UV exposure include:
• Structure and composition of the Martian atmosphere
• Optical properties of Mars Aeolian and surface dust particles
• Solar flux
• Spatial (geographic location) and temporal (day of year, local time, etc.) heterogeneities
• UV particle contact time
• Spacecraft orientation
• Spacecraft geometry
An underlying assumption when considering the UV sterilization potential within the Mars atmosphere is that the damaging effects of UV exposure on hypothetical Mars microorganisms is comparable to that which occurs in Earth microorganisms. This is the basis for understanding Mars UV within the framework of ‘life as we know it’ and is consistent with previous discussions of fundamental chemistries.
In 2017, an exhaustive review of peer-reviewed studies was performed at JPL spanning 50+ years of research on UV inactivation of microbial species (Fig. 3). This work was performed primarily to compile UV lethality data to serve as inputs to models being examined for the Mars 2020 mission.
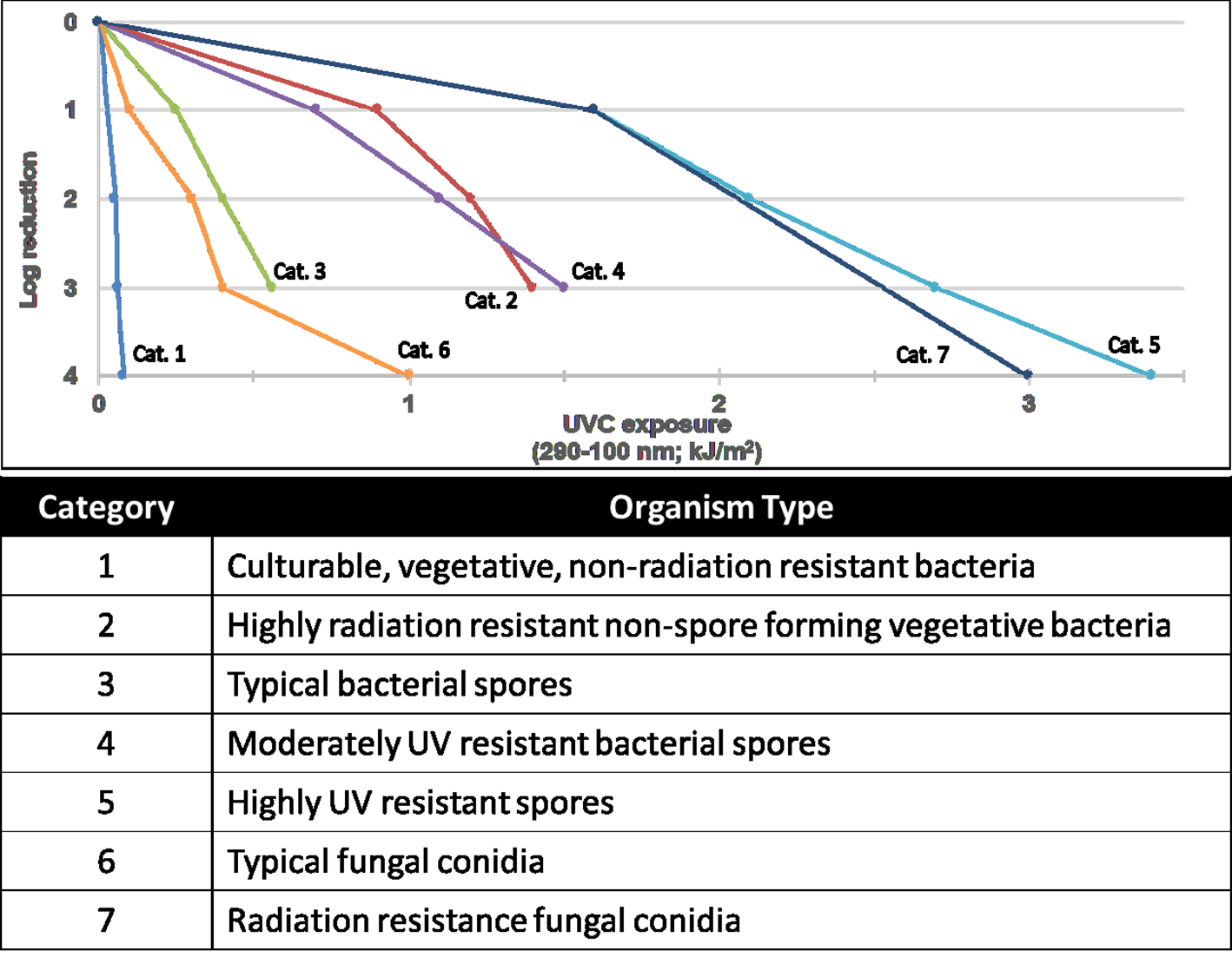
Fig. 3. UV inactivation of microbial species.
Data from UV measurements from NASA's Mars Science Laboratory (MSL)/Rover Environmental Monitoring Station (REMS) and Pathfinder missions indicate that a microbial population exposed to Mars UV would be reduced by 99.999% in only a few minutes after sunrise (Cockell et al., Reference Cockell, Catling, Davis, Snook, Kepner, Lee and McKay2000; Schuerger et al. Reference Schuerger, Mancinelli, Kern, Rothschild and McKay2003; Gómez-Elvira et al., Reference Gómez-Elvira, Armiens, Carrasco, Genzer, Gómez, Haberle, Hamilton, Harri, Kahanpää, Kemppinen, Lepinette, Soler, Martín-Torres, Martínez-Frías, Mischna, Mora, Navarro, Newman, de Pablo, Peinado, Polkko, Rafkin, Ramos, Rennó, Richardson, Rodríguez-Manfredi, Romeral Planelló, Sebastián, Juárez, Torres, Urquí, Vasavada, Verdasca and Zorzano2014). This is the result of a dose of over 12 kJ h−1 of UVC (at local noon on the equator; Cockell et al. Reference Cockell, Catling, Davis, Snook, Kepner, Lee and McKay2000; Patel et al. Reference Patel, Zarnecki and Catling2002; Schuerger et al. Reference Schuerger, Mancinelli, Kern, Rothschild and McKay2003) which is equivalent to the UV required to kill the most UV-resistant microorganism known here on Earth. However, in order to assess the full sterilization potential of Mars UV, the degree of shading and shielding must also be considered. Dust deposition, whether on the surface of Mars or on the spacecraft, can provide spores with protection from UV exposure, and the degree of protection is dependent on the thickness of the dust layer (Mancinelli and Klovstad Reference Mancinelli and Klovstad2000; Schuerger et al. Reference Schuerger, Golden and Ming2012). Several other studies have concluded that both airborne and surface dust loading is an important component in the attenuation and sterilization potential of incoming solar UV in the Martian atmosphere (Schuerger et al. Reference Schuerger, Mancinelli, Kern, Rothschild and McKay2003; Newcombe et al. Reference Newcombe, Schuerger, Benardini, Dickinson, Tanner and Venkateswaran2005; Richards et al. Reference Richards, Newcombe and Venkateswaran2006; Osman et al. Reference Osman, Peeters, La Duc, Mancinelli, Ehrenfreund and Venkateswaran2008; Vaishampayan et al. Reference Vaishampayan, Rabbow, Horneck and Venkateswaran2012). Additionally, life can escape the lethal effects of UV by ‘hiding’ in pits, crevices, etc. (Moores et al. Reference Moores, Smith, Tanner, Schuerger and Venkateswaran2007).
The sterilization potential of Mars–Earth cruise environment on dust
The interplanetary cruise-phase environment between Mars and Earth is composed of several factors that influence microbial survival including:
• High vacuum
• Severe desiccating conditions
• Extreme temperature fluctuations
• Solar UV radiation
• Solar particle events
• Galactic cosmic rays
Of these factors, solar UV radiation and solar heating appear to be the leading contributors limiting microbial survivability on external spacecraft surfaces. However, some studies indicate that some microorganisms may survive these cruise conditions if solar heating and UV are removed (Horneck et al. Reference Horneck, Moeller, Thierry, Mancinelli, Nicholson, Panitz, Rabbow, Rettberg, Spry, Stackebrandt, Vaishampayan and Venkateswaran2012). Additional information needs to be obtained to better understand the effects of the Mars–Earth cruise environment on spacecraft-associated microorganisms.
For the second SWG workshop, the primary focus of the UV presentation centred on whether a consensus could be reached by the SWG on whether Mars UV and Mars-Cruise UV could reasonably provide some degree of sterilization. To frame the discussion, four propositions were presented to the SWG:
(1) UV is highly effective at killing microorganisms.
(2) Any extant Martian life would exhibit a range of UV resistance (from no resistance to high), but none are expected to exhibit absolute immunity to UV. Evidence presented to support this proposition included:
(a) In Mars analogue environments here on Earth, bacteria do not generally relegate themselves to life on the surface where environmental perturbations are at their greatest; microbiota seek refuge in environmental niches which provide protection from the harshest environmental stressors (Dartnell Reference Dartnell2011).
(b) There is no nucleic acid-based life, as we know it, capable of withstanding UV radiation at doses equal to that which occur on the surface of Mars, for more than a few hours. Following are quotes that lend credence to this statement:
‘Although the various Bacillus spp. exhibited diverse levels of UV resistance, none were immune to UV irradiation, and, thus, all species would be expected to be inactivated on Sun-exposed spacecraft surfaces within a few tens-of-minutes to a few hours on sol 1 under clear-sky conditions on equatorial Mars. We have not observed any phenomena in these experiments nor have we found any literature that would support the conclusion that common terrestrial microorganisms typically found on spacecraft are “immune” to the UV environment on Mars.’ (Schuerger et al. Reference Schuerger, Richards, Newcombe and Venkateswaran2006)
‘UV radiation was the key parameter that determined survivability of spores under simulated Martian conditions; direct exposure to UV radiation resulted in rapid and nearly complete inactivation of microbial cultures.’ (Nicholson et al. Reference Nicholson, Schuerger and Setlow2005)
‘UV reaching the surface of Mars has a 1000-fold greater biocidal effect than on Earth.’ (Rummel et al. Reference Rummel, Beaty, Jones, Bakermans, Barlow, Boston, Chevrier, Clark, de Vera, Gough, Hallsworth, Head, Hipkin, Kieft, McEwen, Mellon, Mikucki, Nicholson, Omelon, Peterson, Roden, Lollar, Tanaka, Viola and Wray2014)
(3) In addition to the bioburden reductions resulting from naturally occurring solar UV exposures, artificial (i.e. engineered) UV sources could potentially be developed and deployed to result in improved cumulative inactivation kinetics.
(4) Knowledge and data gaps exist which limit our ability to calculate the degree of bioburden reduction resulting from UV exposure. Needed items to fill these gaps include:
(a) Laboratory testing and experimentation for solar and artificial UV exposures to a wider microbial species diversity than is heretofore been available in the literature.
(b) UV modelling specifically relevant to the future MSR architecture missions.
(c) Dust modelling on Mars.
Synergism among biocidal space factors
A number of papers on the habitability of Mars have discussed biocidal and inhibitory factors (average 20 factors per study) that will impact both the survival and the growth of Earth microorganisms on the surface (Stoker et al. Reference Stoker, Zent, Catling, Douglas, Marshall, Archer, Clark, Kounaves, Lemmon, Quinn, Renno, Smith and Young2010; Schuerger et al., Reference Schuerger, Mancinelli, Kern, Rothschild and McKay2003; Rummel et al. Reference Rummel, Beaty, Jones, Bakermans, Barlow, Boston, Chevrier, Clark, de Vera, Gough, Hallsworth, Head, Hipkin, Kieft, McEwen, Mellon, Mikucki, Nicholson, Omelon, Peterson, Roden, Lollar, Tanaka, Viola and Wray2014; Cockell et al. Reference Cockell, Bush, Bryce, Direito, Fox-Powell, Harrison, Lammer, Landenmark, Martin-Torres, Nicholson, Noack, O'Malley-James, Payler, Rushby, Samuels, Schwendner, Wadsworth and Zorzano2016). The biocidal and inhibitory factors include (but are not limited to): UV irradiation, extreme desiccation, low pressures, low temperatures, CO2-enriched anoxic atmospheres, oligotrophic conditions, volatile oxidants and high salts. When conducting simulation experiments relevant to microbial survival on Mars, it is often difficult to design precise treatments and controls due to interactive effects among these factors. From a biocidal perspective, many of these factors can impact microbial survival through either additive (i.e. the sequential sum of the interactions) or synergistic (i.e. interactions that are greater than the sum of all factors) effects.
The literature on interactive effects among space or Martian biocidal factors suggests that most interactions are synergistic and/or cumulative in nature in which multiple factors typically yield linear models in which the rates of microbial inactivation vary (Bucker et al. Reference Bucker, Horneck, Wollenhaupt, Schwager and Taylor1974; Weber and Greenberg Reference Weber and Greenberg1985). In contrast, additive interactions are typically not generally encountered (Foster et al. Reference Foster, Winans, Casey and Kirschner1978; Horneckücker et al. Reference Horneck, Bücker, Dose, Martens, Bieger, Menningmann, Reitz, Requardt and Weber1984a, Reference Horneck, Bucker, Reitz, Requardt, Dose, Martens, Menningmann and Weber1984b), but when observed, the rates of microbial inactivation are the same and only the starting/ending microbial counts differ. The following discussion will focus on synergistic effects among four biocidal conditions found in both interplanetary space and on the Martian surface (i.e. solar UV irradiation, solar heating, vacuum and ionizing radiation). In most cases, the assays examined only the interactions of two simultaneously-applied factors. Research into multi-factorial interactions for space and Martian biocidal conditions are, in general, lacking.
Vacuum alone
Vacuum imparts two concomitant conditions on bacterial endospores or vegetative cells including reduced atmospheric pressure itself and extreme desiccation effects achieved by lowering the vapour pressure of water around the spores or vegetative cells to extremely low levels. The longest term microbial survival study in space was the 69-month experiment on-board the Long Duration Exposure Facility (LDEF) that examined the survival of Bacillus subtilis spores in evacuated sample holders. Horneck et al. (Reference Horneck, Bucker and Reitz1994) reported that spore survival was 0.3–2% after 69 months compared to the T = 0 controls (regardless if the spores were in monolayers or multilayers) when exposed to the vacuum effects alone in low-Earth orbit (LEO). Similar results have been observed for a number of spore-forming and non-spore-forming bacterial, archaeal and fungal species in space (reviewed by Nicholson et al. Reference Nicholson, Munakata, Horneck, Melosh and Setlow2000; Horneck et al. Reference Horneck, Klaus and Mancinelli2010).
However, Brueschke et al. (Reference Brueschke, Suess and Willard1961) reported that exposures to high vacuum in the lab down to 10−7 Pa for only 48 h yielded no surviving vegetative cells of B. subtilis, Aspergillus niger, A. terreus and Penicillium citrinum. The authors proposed that the results ‘…imply that inadvertent surface contamination of the space vehicles during prelaunch periods may not be a serious problem with space flights of long duration since the surface may be vacuum-sterilized [emphasis added] during flight …’ (Brueschke et al. Reference Brueschke, Suess and Willard1961). Lastly, it is generally accepted that vacuum-only effects will impact non-spore-forming species to a much greater extent (up to 2–6 logs of greater lethality) than spore-forming species in space (Nicholson et al. Reference Nicholson, Munakata, Horneck, Melosh and Setlow2000; Horneck et al. Reference Horneck, Klaus and Mancinelli2010).
Few studies exist that examine the interactive effects of desiccation and vacuum on spore or cell survival under Martian or space conditions (reviewed by Horneck et al. Reference Horneck, Klaus and Mancinelli2010). Saffary et al. (Reference Saffary, Nandakumar, Spencer, Robb, Davila, Swartz, Ofman, Thomas and DiRuggiero2002) showed clear evidence that space vacuum exhibited 2 logs of increased lethality over desiccation alone for both B. subtilis and Deinococcus radiodurans during lab simulations and rocket flights. If confirmed for other bacterial, fungal and archaeal species, it may be possible to place the synergistic biocidal effects of vacuum as separate, and more severe, than desiccation alone.
Recently, Schuerger et al. (Reference Schuerger, Moores, Smith and Reitz2019) summarized and plotted vacuum-only effects on spore viability for B. subtilis against time and developed a biphasic kill-curve (i.e. tailing effects) for vacuum-only. Based on published data, the biphasic plot by Schuerger et al. (Reference Schuerger, Moores, Smith and Reitz2019) suggests that between a few days and 100 days, approximately 50% of a given population of spores in monolayers on aluminium or glass coupons can be inactivated by VAC-only effects (1st phase), but that it requires much longer time periods to approach the 1% survival rate reported by Horneck et al. (Reference Horneck, Bucker and Reitz1994) for the LDEF mission. The biphasic model for vacuum-only effects can then be extrapolated to intermediate or longer periods of time based on a bioburden reduction of approximately 0.02% of the initial population per month (30 days) for conditions similar to the Moon's surface (Schuerger et al. Reference Schuerger, Moores, Smith and Reitz2019).
Vacuum + UV interactions
However, when other conditions are applied concomitantly with vacuum, the lethality of space increases, and often dramatically so. For example, B. subtilis 168 spores were reported to lose viability by only 1–2 logs when UV-irradiated at 101.3 kPa (1 bar) at 20°C, but >3-logs when UV-irradiated at the same flux (0.2–0.4 kJ m −2) under vacuum (Weber and Greenberg Reference Weber and Greenberg1985). In an even earlier study (Horneck et al. Reference Horneck, Bucker and Wollenhaupt1971), UV irradiation in vacuum was found to increase the lethality of vacuum by >2 logs by UV exposures as small as 50 J/m2.
Furthermore, the synergistic interaction of vacuum + UV can be enhanced if the exposures are conducted at higher temperatures. For example, in the same study by Weber and Greenberg (Reference Weber and Greenberg1985), UV exposures under vacuum at 21°C were significantly more lethal (>4 logs inactivation) when compared to similar exposures at − 263°C (10 K) ( 1 log of inactivation), thus the synergistic effects are lessened as space temperatures are lowered. Generally, the effects of vacuum + UV (Nicholson et al. Reference Nicholson, Munakata, Horneck, Melosh and Setlow2000; Horneck et al. Reference Horneck, Klaus and Mancinelli2010; Schuerger et al. Reference Schuerger, Moores, Smith and Reitz2019) seem to indicate that 2 logs of increased lethality can be predicted for Bacillus spores under most conditions of either low-pressure (0.7 kPa) or vacuum (< 10−4 Pa). The biocidal kinetics of UV irradiation (± vacuum) appear to follow a biphasic response in which the first phase generally ends after 4-logs of spore reduction, and the second phase translates to much slower inactivation rates suggesting that a small proportion of spores are either shielded from, or more refractory to, continued UV irradiation (Schuerger et al. Reference Schuerger, Richards, Newcombe and Venkateswaran2006, Reference Schuerger, Moores, Smith and Reitz2019). However, D-values are often calculated only for the first phase of the kill-curves (i.e. using only data for the first 4-logs of reduction) which might yield faster inactivation rates overall than calculating D-values for both phases. Thus, the D-value calculated from the first phase should not be used for further extrapolation of inactivation.
It is often believed that UVC (200–280 nm) alone is responsible for the inactivation of both spores and vegetative cells under space or Martian conditions. But other UV bands can act to increase inactivation of spores; albeit at longer time intervals or higher doses. In a recent study, Moeller et al. (Reference Moeller, Setlow, Reitz and Nicholson2009) tested a number of B. subtilis spore-protein and core-water mutants against individual UV bands from 200 to 400 nm, and reported that all individual bands can impact the viability of spores. For example, UVC irradiation could reduce wild-type (WT) B. subtilis 168 by 2.5 logs following only 1.0 kJ m−2 exposure, but that UVB (280–320 nm) + UVA (320–400 nm) required up to 30 kJ m−2 of radiation for a similar inactivation level. Extending this further, B. subtilis 168 required up to 1 × 104 kJ m−2 of UVA alone to achieve the same level of inactivation. On the surface of Mars, UV irradiation is attenuated at 190 nm due to CO2 absorption in the atmosphere (Kuhn and Atreya Reference Kuhn and Atreya1979); but in space, the photonic energy below 200 nm will contribute to the biocidal nature of solar-UV irradiation. However, only a few studies have explored the effects of interplanetary UV <200 nm, and the topic requires attention.
Simultaneous space conditions
Studies on the interaction between all factors in a space environment have been attempted in low earth orbit on free-flying satellites, the Shuttle, and the International Space Station (ISS). One such mission from the ISS was EXPOSE-E. In this mission (Horneck et al. Reference Horneck, Moeller, Thierry, Mancinelli, Nicholson, Panitz, Rabbow, Rettberg, Spry, Stackebrandt, Vaishampayan and Venkateswaran2012), spores of B. subtilis 168 and B. pumilus SAFR-032 were exposed to the totality of space conditions (vacuum+UV+heat+ionizing radiation) in which the solar UV was either not, partially or completely shielded; and the solar spectrum was shielded so that only photons >110 nm (outer space simulations) or >200 nm (Mars simulations) passed through protective windows. The UV fluence rates varied between zero (fully shielded) and $882\, {\rm MJ\, m}^{-2}$ (interplanetary space). Temperature fluctuated between −20 and 59°C. Cosmic radiation averaged 155 mGy over the course of the 1.5 years of the mission. For both species, vacuum+ionizing radiation effects appeared to increase the lethality of space by 1 log. In contrast, B. pumilus SAFR-032 (a strongly UV-resistant strain) proved to be much more sensitive to the interplanetary space conditions than B. subtilis 168 in which all factors induced a notable reduction in viable spores by SAFR-032 by >7 logs, while the 168 spores appeared to decrease by only 4 logs. Furthermore, the SAFR-032 spores decreased their viability by 7 logs compared to only 2 logs for 168 spores exposed to Mars simulated conditions. Thus, even though B. pumilus SAFR-032 is considered to be one of the strongest UV-resistant Bacillus species recovered from spacecraft or spacecraft assembly facilities (Link et al. Reference Link, Sawyer, Venkateswaran and Nicholson2004), it appears when other concomitant space biocidal effects are present, their combined effects impact spore survival much more for SAFR-032 than the lab-standard B. subtilis 168 strain.
Although other LEO experiments (Vaishampayan et al. Reference Vaishampayan, Rabbow, Horneck and Venkateswaran2012; Panitz et al. Reference Panitz, Horneck, Rabbow, Rettberg, Moeller, Cadet, Douki and Reitz2015) generally agree with the biocidal effects of the space conditions discussed above for B. subtilis 168 and B. pumilus SAFR-032 (see Horneck et al. Reference Horneck, Moeller, Thierry, Mancinelli, Nicholson, Panitz, Rabbow, Rettberg, Spry, Stackebrandt, Vaishampayan and Venkateswaran2012), a few studies tested similar species but reported more modest effects of the space conditions on survival. For example, Moeller et al. (Reference Moeller, Reitz, Li and Nicholson2012) tested B. subtilis 168 during the same EXPOSE-E mission and observed a modest 3 log decrease in surviving spores over the course of the 1.5-year mission. Although unknown, the most likely explanation for the divergent results between Horneck et al. (Reference Horneck, Moeller, Thierry, Mancinelli, Nicholson, Panitz, Rabbow, Rettberg, Spry, Stackebrandt, Vaishampayan and Venkateswaran2012) and Moeller et al. (Reference Moeller, Reitz, Li and Nicholson2012) were procedural methodologies in the preparation of spore layers, and experimental error in processing the samples, rather than the biocidal conditions present in space.
Although early research has suggested that the space environment will ‘...vacuum-sterilize...’ microorganisms inadvertently emplaced on spacecraft surfaces prior to launch (Brueschke et al. Reference Brueschke, Suess and Willard1961), most of the subsequent studies have not verified this conclusion. Most ground-based experiments with vacuum, UV, cosmic radiation and/or heat have indicated that all four of these factors can increase the lethality of space, and that synergistic interactions occur that increase the lethality of space by 1–7 logs depending on the number of interactive factors present, the duration of the assays and the types of spores/cells tested.
Recently, Moores and Schuerger (Reference Moores and Schuerger2020) reported a new Cruise-Phase Microbial Survival (CPMS) model for long interplanetary missions to the outer planets. The CPMS predicts that bacterial spore-forming species (e.g. Bacillus spp.) are likely to be fully inactivated if mission lengths are >6 years. Furthermore, Dillon et al. (Reference Dillon, Holdridge, Puleo and Oxborrow1971) examined the thermal inactivation rates of most microorganisms present on Lunar spacecraft and determined that most species should be inactivated within 9–12 months on the Lunar surface. Recently, Schuerger et al. (Reference Schuerger, Moores, Smith and Reitz2019) updated and extended the models of Dillon et al. (Reference Dillon, Holdridge, Puleo and Oxborrow1971) and showed that it is plausible to achieve up to 2479 log reductions on external spacecraft surfaces per lunation, but only approximately 0.02 log reductions per lunation on deeply embedded internal surfaces that are thermally isolated from solar heating.
In addition, a significantly larger list of biocidal or inhibitory factors is present on the surface of Mars (e.g. UV irradiation, extreme desiccation, low-pressures, low-temperatures, CO2-enriched anoxic atmospheres, oligotrophic conditions, volatile oxidants and high salts) (Stoker et al. Reference Stoker, Zent, Catling, Douglas, Marshall, Archer, Clark, Kounaves, Lemmon, Quinn, Renno, Smith and Young2010; Schuerger et al., 2013; Rummel et al. Reference Rummel, Beaty, Jones, Bakermans, Barlow, Boston, Chevrier, Clark, de Vera, Gough, Hallsworth, Head, Hipkin, Kieft, McEwen, Mellon, Mikucki, Nicholson, Omelon, Peterson, Roden, Lollar, Tanaka, Viola and Wray2014; Cockell et al. Reference Cockell, Bush, Bryce, Direito, Fox-Powell, Harrison, Lammer, Landenmark, Martin-Torres, Nicholson, Noack, O'Malley-James, Payler, Rushby, Samuels, Schwendner, Wadsworth and Zorzano2016). While the interplanetary space environment might achieve substantial lethal doses for spacecraft microorganisms (Schuerger et al. Reference Schuerger, Moores, Smith and Reitz2019; Moores and Schuerger Reference Moores and Schuerger2020), adding in to the assays the numerous additional biocidal factors on the surface of Mars might greatly increase the lethality of the space environment on the MSR spacecraft by many orders of magnitude. On the other hand, it is possible that Martian microbes carry some resistance to the Martian biocidal factors on the surface, and that only when removed and taken into space, do the factors variant from the surface begin to increase microbial inactivation. As this analysis continues, it is necessary to consider both options as possible until empirical data are available to demonstrate one or the other, or neither options as accurate.
Conclusions: Passive Inactivation
Understanding passive inactivation mechanisms and their biocidal effects are important inputs to the determination of the potential quantity of active Martian material prior to any containment or additional inactivation processes. The consensus conclusions of the SWG are as follows:
(1) Current data and understanding support the hypothesis that the UV radiation present on Mars, in orbit, and during the Mars–Earth cruise journey phase will likely result in a reduction of extant Martian life (if present).
(2) The significantly stronger UV source when outside of Mars’ atmosphere should increase the inactivation of Martian microbiota.
(3) There is potential for engineered (active) sources of UV, such as UV LEDs, to provide additional UV exposure to aid in the inactivation of Martian material. However, it was felt that anything that had survived the Martian and space UV environments would either be shielded within a dust particle or otherwise adapted to withstand UV. Therefore, UV should not be considered as the sole method for active inactivation.
(4) The working group had some discussion about whether we can leverage additional passive inactivation by leaving the OS in orbit as long as possible around Mars before sample retrieval. This also ties into the quantity of dust particles that may be in orbit on or around the OS, the inactivation of these particles and their proximity to the OS during retrieval.
Future Work: Passive Inactivation
The consensus of future work suggested by the SWG is as follows:
(1) Additional laboratory experiments will be required to generate further empirical data needed to fully elucidate the efficacy of UV sterilization in the various potential conditions where microorganisms could be expected to reside.
(2) Empirical data generated from additional laboratory studies can be used as inputs into newly developed MSR-relevant models to further address questions surrounding MSR-BTC that are not easily replicated in the laboratory. To support these studies, detailed procedures and validation and verification protocols should be included for inactivation challenge testing.
(3) Additional research is required to quantitatively constrain the synergistic interactions among the various space and Martian biocidal conditions.
Break the chain: containment and active inactivation
The current concept for MSR campaign architecture necessitates a process to BTC of contact between Earth and Mars. Current architecture scenarios feature a containment and encapsulation strategy for the sample tubes, and any additional Earth-return hardware exposed to the Mars environment but not subsequently sterilized. Through progressive encapsulation, the amount of active sterilization required to successfully break the chain is reduced (but not eliminated). While the MSR campaign is anticipating that two robust, redundant containment vessels are all that would be required to meet the containment assurance needs during an agreed upon set of off-nominal landing conditions, the additional margin provided by the following hardware elements increases the robustness of the containment assurance strategy:
• The OS would contain the sample tubes by means of a dust tight seal. The exterior of the OS would not have direct contact with the Martian surface, but it would likely have limited contact with the Martian atmosphere, including dust blown in the wind.
• The OS would be contained for return to Earth in the Primary Containment Vessel (PCV), the exterior of which would have no direct or indirect contact with Mars or the Martian atmosphere.
• During nominal operation, the only surface of the PCV that could potentially contact Martian material would be the seam line, as the OS could potentially transfer material during assembly. The seam line may require sterilization.
• The PCV would be placed into the Secondary Containment Vessel (SCV), which provides redundancy during the EEV landing event.
• The SCV is integral to the Earth Entry System (EES). The EES is designed to provide a regulated load environment to the PCV and SCV on landing, possibly destructively, during off-nominal impacts.
Active inactivation can be an additional step(s) engineered into the campaign architecture, to provide assurance that any Martian material is rendered safe for return to Earth. The quantity of active inactivation to be designed into the system is directly proportional to the difficulty of the engineering process. Although inactivation, often called sterilization in health care settings, is ubiquitous in health care on a daily basis, it has not yet been proven in space environments. Thus, there is a desire to implement only the quantity of active inactivation modalities deemed necessary.
BTC concepts
MSR would be classified as a Category V Restricted Earth return mission (National Aeronautics and Space Administration 2011). BTC is defined as an active, surface-to-surface (Mars to Earth) process to satisfy BPP goals by prohibiting the uncontrolled transmission and release of Mars material of concern into Earth's biosphere. It is important to note three key phrases within this definition:
(1) Active inactivation: In order to create a robust and defendable BTC process, active measures are necessary to combat the significant (and perhaps unknowable) uncertainties present in some aspects of the MSR campaign.
(2) Surface-to-surface (Mars to Earth): BTC is not accomplished in a single step. It is the aggregation of all active and passive processes from the target body back to the Earth's biosphere. For MSR, factors influencing BPP begin when Mars 2020 accumulates dust on the surface of Mars after landing in 2021. The process ends once the EEV has been robustly contained on the surface of Earth and its landing site is remediated, approximately in 2031.
(3) Material of concern: While a robust BTC process recognizes that a conservative and margined estimate of Martian material should be utilized for the design, analysis and testing of the engineered systems, it may take into account the ability to inactivate percentages of the bulk materials returned through active or passive processes. Note that while inactivated materials may not be ‘of concern’ for return to Earth, it may still act to impair further inactivation measures applied to the bulk materials (shielding, blocking, etc.).
There are three primary tools which can be utilized to BTC with Mars:
(1) Particle transport (adhesion, emission and transmission)
(2) Containment (including sealing, encapsulation, isolation and blocking)
(3) Inactivation (including both passive and active means).
While all three tools can heavily impact the amount of material returned, note that only containment and inactivation can be directly controlled and verified.
Particle transport refers to the processes in which Martian material moves through the campaign's engineered systems during surface operations and the return trip to Earth. This material can change locations through natural or artificial forcing functions (e.g. wind, orbital dynamics, drilling, driving, etc.). Once in motion, it can both adhere to new surfaces and dislodge from them.
Containment measures either enclose Martian material within a defined volume or prohibit the transmission of material from one side of a boundary to another. The efficacy of these boundaries can be adjusted to meet system-level requirements (i.e. native particle loading and morphology, required particle transmission levels, mass, etc.), and boundaries can be either permanent or temporary depending on the use case. It is important to note that while containment boundaries can be highly effective once created, one cannot control where material of concern lands on a seam line during closure. While many joint designs would prohibit particle flow to some degree, three seals are being considered on MSR to regulate transmission of material:
(1) Compression seals (e.g. o-rings, engineered lip seals, etc.) are readily available throughout industry in a variety of forms. They can be rated to be gas tight when implemented properly.
(2) Melt seals (e.g. solder, braze, etc.) utilize material flowed into a seam line to prohibit transmission. Note that the seal material is also the retention system for the joint. These can be rated to gas tight and have the added advantage of being capable of inactivating and encapsulating Mars material of concern during formation (given design to a time-temperature lethality curve).
(3) Labyrinth seals prohibit particle motion through a seam line by means of a tortuous path. These are not gas or liquid tight, and may require the development of nanometre particle detection to verify for use in the MSR BTC strategy.
These seals would likely be used throughout the mission to block accumulation of Martian material while on the planet's surface, prohibit its transmission to designated ‘clean zones’, and to close out returning containment vessels.
Sterilization and inactivation, applied through engineered systems, would be utilized strategically to facilitate the control of Mars material. Complete inactivation of surfaces was considered, although the use of geometry may relieve the campaign's need to do this. Partial inactivation may be utilized on certain surfaces and volumes to reduce the overall quantity of material of concern as required to close the end-to-end BTC architecture (e.g. before launch of the Mars Ascent Vehicle (MAV), after OS capture, etc.).
Within MSR, aseptic transfer is defined as the combination of containment and sterilization to move an object from an environment with material of concern to one that has been isolated from material of concern (a ‘clean zone’). Although containment boundaries can be highly effective once created, one cannot control where material of concern lands on a seam line during the container loading or closure process. By utilizing an active sterilization process during or after the closure process, one can render the remaining particles of concern that may exist on the seam line inert and safe.
Presently, a brazing operation with a double walled lid is envisioned to accomplish aseptic transfer to a ‘clean zone’ on the CCRS payload after the OS is captured in Mars orbit (Gershman et al. Reference Gershman, Bar-Cohen, Hendry, Stricker, Dobrynin and Morrese2018). Aside from aseptic transfer, this process creates the PCV; one of two containment vessels used in the present MSR architecture. Brazing provides for a number of benefits, including late-game design flexibility in allowing for adjusting time and temperature for sterilization, encapsulation of trapped Mars material and a self-securing sealed interface (Fig. 4).
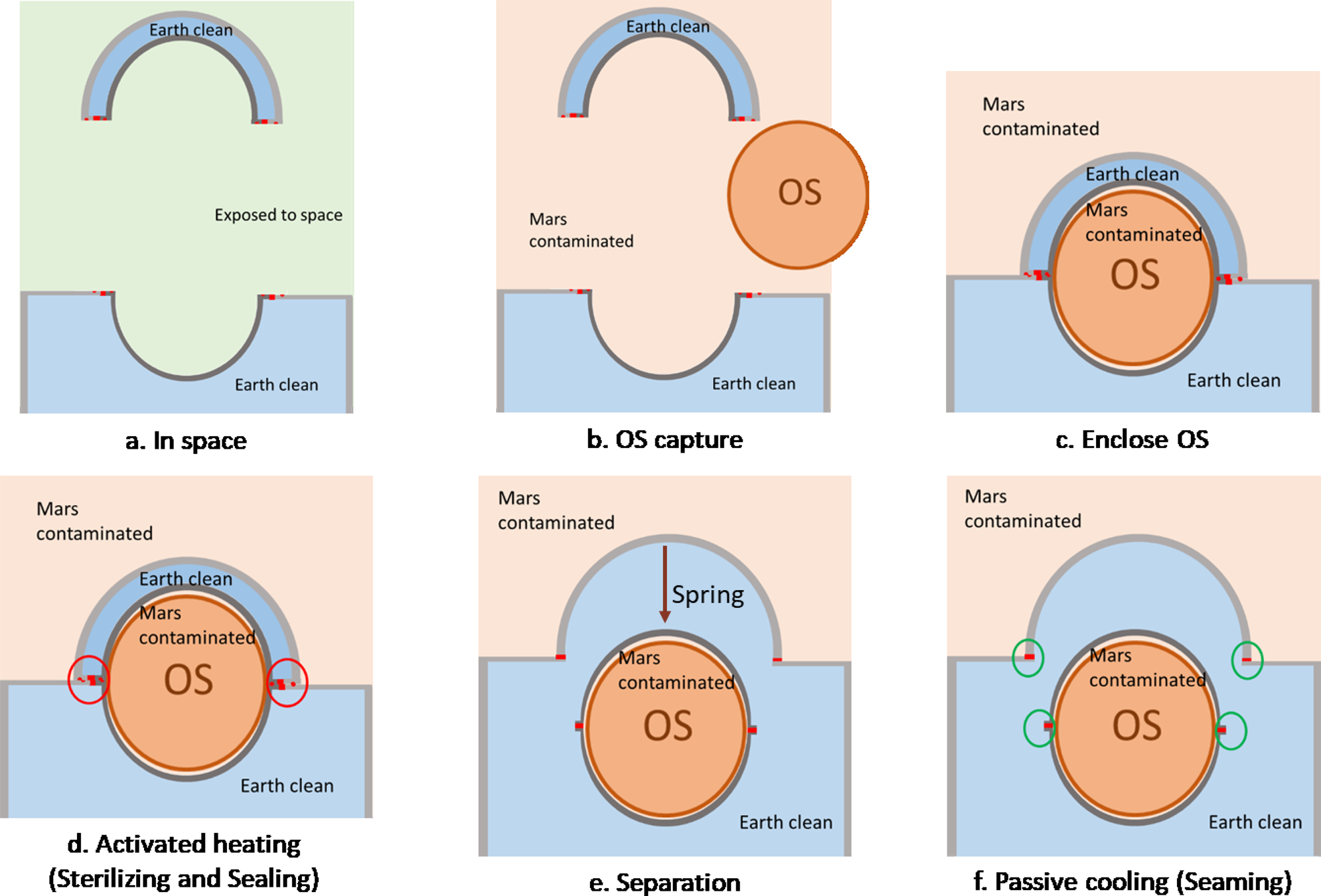
Fig. 4. Double-lid break-the-chain concept – utilizing a double-walled lid and heat to aseptically transfer from a Mars contaminated zone to an isolated (‘Earth clean’) zone within a spacecraft. Note that the container base forms a segment of the wall of the spacecraft. Presently, soldering or brazing is envisioned for this process. (Gershman et al. Reference Gershman, Bar-Cohen, Hendry, Stricker, Dobrynin and Morrese2018)
While work at JPL continues to solidify the BTC architecture for MSR, three critical pinch points have been established. Pinch points are defined as times and locations in the return process where a small action can have a far reaching benefit for the overall BTC process.
• Within the MAV thermal enclosure and MAV Payload Assembly (MPA) before launch from the Sample Return Lander (SRL).
• Within the CCRS on the ERO in Mars orbit, before departure.
• At the EEV recovery site, immediately following landing.
These locations and times represent the most effective places to insert active measures to address material of concern through containment and sterilization.
Criteria for active inactivation processes
In order for an active inactivation process to be considered for the MSR programme, it must satisfy certain criteria:
• It must be capable of providing the required inactivation of biological challenge agents or molecules;
• It must be able to be validated in the environment in which it is flown;
• It must have a Technological Readiness Level (TRL) appropriate for deployment in space;
• It must be able to act on particles on or around the OS without affecting the samples inside.
The most commonly used sterilization modalities in the medical device industry are ethylene oxide gas, radiation and moist and dry heat. During the 2nd SWG workshop, approximately 30 chemical options were partitioned into three groups for BTC. Each option represented a different application on various surfaces of the spacecraft. There was consensus in understanding the pros and cons for all chemicals. System constraints eliminated several chemicals due to the ability to empirically prove effective sterilization in flight, shelf-life considerations, the ability to safely handle during Assembly, Test, Launch, Operations (ATLO), and having low probability of sample contamination. While it was determined that NASA had success using EO on Earth in the past early Ranger missions, the SMEs agreed that there were still significant safety issues using EO in orbit and that other chemicals needed to be tested in parallel to fill data gaps using current biological challenge agents. By far the simplest of these modalities from a technological standpoint is dry heat. The validation pathway for dry heat is well understood, and has been used to satisfy sterilization practices within industry and for forward planetary protection goals for decades in the planetary exploration world, and can be implemented in a space environment. Depending on the design of the process and the containment structure, heat can penetrate to areas of interest without negatively affecting the sample tubes inside the OS.
Whichever active process is chosen, it must also meet requirements for flight in space. In aerospace, mass is often the main driver for design choices. These choices affect the launch vehicle selection, specification of inertial loads on hardware, orbital transfer times, and entry, descent and landing (EDL) analysis. This driver is not just considered with respect to the payload one sends, but also the required support systems (power, thermal, propulsion, structures, etc.). Power requirements must also be considered. Landed missions, including the SRL, typically have limited energy available, as they are often solar powered and have restricted battery storage. Large orbital busses, such as the ERO, do not typically face these constraints, as they may have larger solar arrays. Specifically, the ERO is optimized for the high-energy requirements of electric propulsion. Thus, the right sterilization modality should be selected holistically for each mission.
Dry heat sterilization in the context of Mars sample return
Of all of the established sterilization modalities, dry heat is the most widely accepted process for sterilization in the aerospace community for forward planetary protection efforts, and as such, it was readily adopted by the SWG as a front runner for BPP. As previously described, the aseptic transfer system utilizes heat to form a brazed seal of the Primary Containment Vessel (PCV). Brazing (and its lower temperature variant, soldering) can use a variety of materials with a range of melting temperatures, which, along with joint geometry, can be tuned to meet desired performance requirements. Per standard spacecraft design principles, the lowest temperature braze that meets mission requirements (including impact performance and sterilization) with an appropriate margin would be used to preserve mass, power and thermal resources at the ERO system level. The application of heat to seal the PCV would also be sufficient to sterilize the closure interface.
Dry heat is the preferred sterilization technique for the following reasons:
• Dry heat is a NASA-approved technique for microbial reduction in forward planetary protection, and there exists successful extensive experience with outward-bound spacecraft hardware.
• It is more compatible with spacecraft parts and components than several other techniques.
• Dry heat can be applied in a vacuum, which is preferred.
• Heat transfer and thermal regulation on spacecraft is a refined and well-established engineering practice that has been demonstrated on every successful satellite mission flown.
• Precise control of the relevant factors (heat and time) is possible.
• The heat penetrates below surfaces into crevices where gas cannot diffuse.
• Heat transfer is not affected by the lack of gravity, or the absence of convection, as chemical processes would be.
• Even in the case of extremely unlikely speculative biology, there appears to be no microorganisms (or structured materials) that are immune to heat inactivation or destruction, presuming the appropriate level of heat is applied for the appropriate time.
Bacterial spore inactivation studies in the literature have very little information on temperatures at or above 200°C and under conditions analogous to those expected to be encountered in the MSR scenario. Assessment of the time and temperatures needed to achieve sterilization have been initially assessed using a rapid heating device whereby dried bacterial spores are inoculated on silicon coupons and rapidly heated by halogen bulbs with control circuitry that modulates the temperature (Schubert et al. Reference Schubert, Ronney, Camasmie, Siu Hay, Koldos and Ronney2018). Preliminary results were presented during the workshops which demonstrated that the standard BI spore Bacillus atrophaeus ATCC 9372 is quickly inactivated at 200°C and at higher temperatures (Fig. 5). Data were also presented that the heat-resistant Bacillus sp. ATCC 29669 spores were inactivated at 225°C and above (Fig. 6).A more traditional approach that permits the heating of dry spores in a stainless-steel tube is also a method that would be employed. While the heating ramp is not as rapid as the device mentioned above, the stainless-steel tube exposure technique fits the time and temperature profiles expected to be encountered in the sample return hardware brazing method. From Fig. 7, it would be expected that a 6-log reduction of bacterial endospores would be achieved in 3 min at 200°C. However, experiments undertaken to demonstrate complete spore inactivation at temperatures above 200°C and up to 300°C for 1 to 10 min, survivors were occasionally observed (Schubert, unpublished). At this point, it was unclear if the survivors were a real tailing observation or were an experimental artefact. The survivors were sub-cultured and identified as a Bacillus sp. ATCC 29669 by Bruker Biotyper MALDI-TOF analysis.

Fig. 5. Bacillus atrophaeus (ATCC 9372) spore inactivation heating profile – the industrial biological indicator spore for dry heat B. atrophaeus ATCC 9372 was exposed to rapid spike profiles. The exposures consisted of beginning at ambient temperature and rising to the designated temperatures in 60 s. Once the target temperature was reached, the heat input was stopped and the test silicon coupons began to cool. In these experiments, the time spent at temperatures where significant inactivation occurred was relatively short. For example, during the 200°C ramp, the time above 110°C was 40 s. Minor rates of spore inactivation are initially observed as low as 135°C with a 1 log population drop at 170°C. All spores were inactivated reaching target temperatures of 200°C or higher. No colony forming units were observed at 200°C or higher, but for purposes of this graph, the zero values were plotted as 1 (Schubert et al. Reference Schubert, Ronney, Camasmie, Siu Hay, Koldos and Ronney2018).
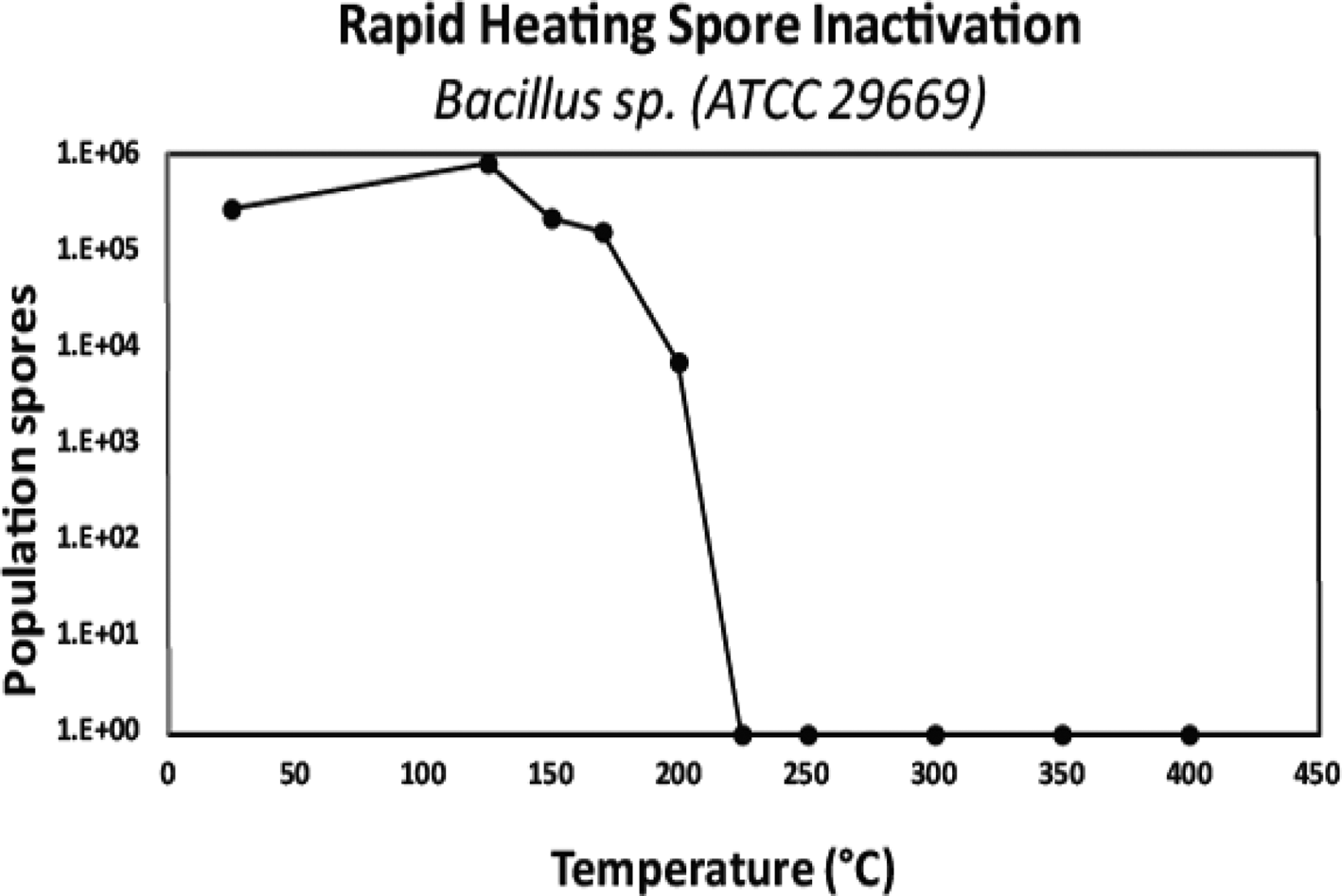
Fig. 6. Bacillus spp. (ATCC 29669) spore inactivation heating profile – the heat-resistant spore ATCC 29669 was exposed to rapid spike profiles. The exposures consisted of beginning at ambient temperature and rising to the designated temperatures in 60 s. Once the target temperature was reached, the heat input was stopped and the test silicon coupons began to cool. Minor rates of spore inactivation were initially observed as low as 150°C with a 1 log population drop at 200°C. All spores were inactivated reaching target temperatures of 225°C or higher. No colony forming units were observed at 225°C or higher, but for purposes of this graph, the zero values were plotted as 1 (Schubert et al. Reference Schubert, Ronney, Camasmie, Siu Hay, Koldos and Ronney2018).

Fig. 7. Bacillus atrophaeus (ATCC 9372) and Bacillus spp. (ATCC 29669) D-values – D-values are defined as the time at a given temperature that will inactivate 90% (one decimal reduction) of a population of microorganisms. For B. atrophaeus spores, each D-value point was determined from the slope of the line for 5 time points at each temperature, done in triplicate, for a sum of 75 independent determinations. The Bacillus sp. ATCC29669 D-value plot was fitted from 120 independent determinations. Errors are given at the 95% confidence limit. R2 is the coefficient of determination for a regression analysis. Error bars are smaller than most symbols in this chart (replotted from Kempf et al. (Reference Kempf, Schubert and Beaudet2008), and Schubert and Beaudet (Reference Schubert and Beaudet2011).
Exposures of 106 spores dried at the bottom of Thermal Spore Exposure Vessels (TSEVs) which were then recovered, diluted and plated indicated that sterilization was possible at 250, 270 and 300°C for 2 min (Schubert, 2020 unpublished), but the number of tests were few and some survivors were noted. The phenomenon of tailing (small numbers of survivors) was discussed.
Data were presented from Mars 2020 experiments where heat-hardy ATCC 29669 spores deposited onto titanium coupons were uniformly inactivated at temperatures of 200, 250 and 300°C at times as short as 5 min (total exposure time) (Schubert, 2020 unpublished). The workshop consensus was that 250–300°C is a good minimum sterilization target temperature range for the brazing process to seal the OS, but further tests to confirm this are needed.
Future work should include extensive testing to validate a minimum time and temperature for complete inactivation for the chosen resistant spore population. Also important will be determination of Z-values to enable extrapolation to higher temperatures where the exposure time is extremely short (e.g. <1 s). Special precautions for ensuring the test fixtures, tubes and solutions are planned. Binary tests (also called fractional positive tests), in which spores deposited onto coupons, exposed at specified times and temperatures, and placed into sterile broth for detection of growth will be the primary focus. These tests have the feature indicating that any spore capable of growth can be detected. It also simplifies the testing protocol to a single transfer of a coupon, in contrast to the much more laborious dilution series procedures which require up to 48 pipetting steps per analysis. Another unknown to be evaluated will be the influence of a Martian dust simulant on the time and temperature of inactivation. It is known that sand and soil can contribute to the heat resistance of spores.
Current literature for dry heat inactivation in regards to prions is focussed on sterilizing clinical materials and instruments. The majority of tests were performed with either brain tissue homogenates (e.g. Weinstein et al. Reference Weinstein, Rutala and Weber2001) or whole brain materials, thus the hydrophobic brain tissues with high contents of lipid may contribute to the ‘protective effect’ where the tissue shields the embedded prions from the inactivating agents. This may partly explain why prions seem to be difficult to inactivate with dry heat. Another possible explanation for prion survival at high temperatures is due to uneven heat distribution in brain materials (Rohwer Reference Rohwer1984). Regarding the MSR architecture, we discussed inactivation of self-perpetuating protein assemblies on spacecraft-associated surfaces, potentially associated with Martian dust. It was concluded that new research is required to evaluate the effectiveness of dry heat with purified prions to avoid the shielding effects of biological materials. A study performed by Bélondrade et al. (Reference Bélondrade, Jas-Duval, Nicot, Bruyére-Ostells, Mayran, Herzog, Reine, Torres, Fournier-Wirth, Béringue, Lehmann and Bougard2020) demonstrated that the inactivation of purified human prions can be achieved after moist heat exposure at 134°C for 20 min. Heat has been shown to inactivate proteins by increasing kinetic energy of molecules causing the materials to dissociate. For example, prion-interlocking side chains (Makin et al. Reference Makin, Atkins, Sikorski, Johansson and Serpell2005) held together by hydrogen bonds, van der Waals forces (Kupfer et al. Reference Kupfer, Hinrichs and Groschup2009), and hydrophobic and electrostatic interactions are disrupted as a result of heating. The workshop consensus was that dry heating at the temperature range of 250–300°C used to inactivate spores would also be a good approach for targeting protein assemblies; however, additional work is needed to better understand the kinetics of the inactivation or self-perpetuating protein assemblies in these conditions.
Conclusions: Containment and Active Inactivation to BTC
Discussion during the three workshops centred on understanding the various methods of active inactivation modalities currently available and being implemented by industry, academia and at NASA Centers. The consensus conclusions of the SWG are as follows:
(1) In the review of multiple inactivation methods, dry heat and chemical sterilization appeared to be the most suitable modalities for the MSR.
(2) It would be best to choose modalities with which the scientific community and public were already comfortable for ease of acceptance and public communication.
(3) Dry heat sterilization represents the best modality used to date across all sterility assurance laboratories and had the appropriate data history using a variety of BIs, which meets the necessary TRL, and integration feasibility given the limitations on spacecraft flight requirements.
(4) A soldering or brazing process (at temperatures > 250°C) was proposed to establish the structural bond of the primary containment vessel of the OS and might be part of the aseptic transfer process. At these temperatures, the brazing process could also provide active inactivation of Martian material potentially present in the seam of the Primary Containment Vessel. At the brazed junction, any foreign material including dust should be inactivated, encapsulated and protected from release.
(5) The initial dry heat data are encouraging, which demonstrated effectiveness at 300°C for 10 min against the hardiest available spore species; however, the data showed variable results at the lower temperatures. These confounding data points could be a result of the assay technique or true survival of the hardy spores.
(6) Overall, the workshop consensus was that 250–300°C is a good sterilization target range, and further tests to confirm this are needed.
(7) Chemical sterilization was also an attractive modality for BTC that was discussed during all three workshops. Sterilizing gases are typically used when exposure to other methods (heat or radiation) could damage the materials or equipment. The most common gas used for sterilization is ethylene oxide (EO), with other gasses such as hydrogen peroxide, ozone, mixed oxides of nitrogen and chlorine dioxide also available.
Future Work: Containment and Active Inactivation to BTC
The consensus of future work suggested by the SWG are as follows:
(1) More data are required to assess dry heat for MSR in-flight applications.
(2) Future work will include extensive testing at JPL and industry collaborators to validate a minimum time and temperature for complete inactivation for the resistant bacterial spore population and proteinaceous molecules.
(3) Another unknown to be evaluated will be the influence of Martian dust simulant on the time and temperature of inactivation.
(4) After receiving presentations from three laboratories which specialized in gaseous chemical decontamination and sterilization, it was determined that future collaborations with these laboratories and JPL will be developed to answer the outstanding questions regarding the feasibility for chemical sterilization to be implemented as part of the BTC strategy.
Risk communication
An endeavour to bring Mars samples back to Earth would be the boldest of its kind since Apollo. Thus, any such plan to return samples should be expected to draw unprecedented interest from multiple audiences. These audiences include decision-makers in government, the public and interested parties from around the world; especially since MSR is currently envisioned as a collaborative international effort. The potential risks associated with returning samples from Mars are likely to be low-probability, yet high-consequence risks that can be effectively addressed using the principles of risk communication.
The SWG recognized that addressing the concerns of stakeholders as well as decision-makers in government and international bodies via a sound Risk Communication Plan is key, and if done effectively, should help with both initial public discussions and ultimate prospects for the success of MSR. The risk communication plan and related processes should be coordinated between participating local government bodies, federal and international agencies, and take advantage of the knowledge and experience in academia, government and industry. It should allow for flexibility so that products and processes can be responsive to the possibility of revised estimates of technical risks, changing political and regulatory environments, and the evolving needs of various audiences.
Risk communication efforts should accurately and effectively communicate possible risks, be prepared to acknowledge and respond to public concerns and comments, and include clear communication about agencies’ readiness to respond to contingencies. The strategy should allow the use of new and existing platforms to address the ever-changing media environment.
Summary
The SWG proved to be a success in engaging multidisciplinary SMEs and stakeholders to review and address sterilization/inactivation concepts and data for MSR. Discussions resulted in important and useful understandings of the importance of the various inactivation processes and their applicability for spaceflight.
In answering the initial questions from this collaboration as provided in the Introduction section, the SWG reached a general consensus that:
(a) Sterilization/inactivation decisions for BPP can be based on biology as we know it, focussing on fundamental chemistries.
(b) The most probable source of contamination is likely to be dust particles on or around the OS.
(c) Sterilization modalities that are effective on Earth should be part of the MSR planetary protection toolkit.
(d) Passive processes including UV exposure, heat, vacuum and combinations of these factors will likely contribute to the inactivation or Martian material.
(e) The brazing of the PCV seam can provide an active inactivation step in addition to the containment of Martian material in the form of dust particles.
(f) Additional active processes including those based on dry heat and chemical sterilization could be engineered into the campaign architecture.
Moreover, discussions validated current sterilization/inactivation parameters, defined additional parameters and identified future research investments in planetary protection that would help better quantify and qualify outstanding data. There are several areas of inactivation that require further analysis or additional data points for all stakeholders to conclude with certainty which modality would be effective at meeting the stringent requirement for BPP for MSR.
As highlighted in earlier sections, typically both overkill- and bioburden-based methods of industry sterilization validation require some estimation of the types, levels and resistance of bioburden that may be found on products. For spacecraft coming from Mars, assumptions based on data will need to be made about potential types of viable putative Martian biology, their quantities and the extent of the inactivation processes in order to assign a safety margin to the collection of sterilization procedures. Knowledge and data gaps exist which limit our ability to calculate the degree of bioburden reduction resulting from UV exposure on Mars, in Martian orbit, and during the cruise to Earth; therefore, additional testing is needed to generate empirical data for solar and artificial UV exposures in the context of Martian dust among the various space and Martian synergistic biocidal conditions.
Once an active inactivation process or processes have been identified along with surrogate Earth biological challenge organisms and proteinaceous molecules for inactivation challenge, future studies will need to be validated to fully understand the inactivation capability in context of the space environment. Based on feedback from industry partners, those experimental studies should mirror the approaches used in sterilization of health care products, including use of internationally recognized standards (e.g. the International Organization for Standardization, ISO, the Association for the Advancement of Medical Instrumentation, AAMI, etc.). If similar essential genetic components and chemistries are shared between Earth and Mars, it can be considered a reasonable expectation that they would obey similar rules for inactivation/sterilization. The results from these experiments will be the final input in the overall quantitative evaluation of the potential for harmful biological contamination and associated risk assessment for MSR to help meet anticipated regulatory challenges and provide a foundation for effective risk communication.
The continuation of this work is planned through the establishment of a formal advisory group, chartered under the NASA Planetary Protection Office (PPO). This group is expected to provide formal recommendations to the PPO to aid in meeting BPP requirements while achieving the objectives for the MSR campaign.
Acknowledgements
The Workshop organizers acknowledge the support of Dr. Lisa Pratt (NASA Planetary Protection Officer), Dr. Gerhard Kminek (ESA Planetary Protection Officer) and the Mars Exploration Program Office for their sponsorship of this working group. Collectively, the invited experts, NASA and JPL experts contributed in many ways to the deliberations and conclusions at the working group. Finally, we would like to thank Carla De La Paz and Barbara Saltzberg for their Executive Administrative support, logistics coordination and travel arrangements for invited experts.
Note: The decision to implement Mars Sample Return will not be finalized until NASA's completion of the National Environmental Policy Act (NEPA) process. This document is being made available for information purposes only.
Appendix A: Working Group Participants
External Subject Matter Experts
• John Logar, Johnson & Johnson
• Gerald McDonnell, Ph.D., Johnson & Johnson
• Rohit Chitale, MPH, Ph.D., Defense Advanced Research Projects Agency (DARPA)
• Thomas Coohill, Ph.D., Department of Physics and Astronomy; Siena College Loudonville, NY
• Emily Craven, P. Eng, Mevex
• Paul Littley, Nelson Labs
• Rocco Mancinelli, Ph.D., NASA-Ames; Bay Area Environmental Research Institute
• Gary R. Mitchel, South Coast Sterilization Consulting
• Kevin O'Hara, Sterigenics
• Gary Ruvkun, Ph.D., Harvard Medical School and Department of Molecular Biology, Massachusetts General Hospital
• Martell Winters, Nelson Labs
• Kris Zacny, Ph.D., Honeybee Robotics Spacecraft Mechanisms Corporation
• Peter Emanuel, Ph.D., U.S. Army Edgewood Chemical and Biological Center
• Andrew C. Schuerger, Ph.D., University of Florida Space Life Sciences Lab
• Yury Chernoff, Ph.D., Georgia Institute of Technology
• Brian Bennett, U.S. Army CCDC Chemical Biological Center (CCDC-CBC) The Biotesting Division
• Christine Pearson, Assoc. Director for Communication, Division of High Consequence Pathogens and Pathology, CDC
• Kim Hummel. Ph.D., Acting Director Division of High Consequence Pathogens and Pathology, CDC
• Joseph Wood, US EPA Office of Research and Development; Center for Environmental Solutions and Emergency Response
• Douwe Mason, Research & Technology Development; The Sabre Companies
NASA and JPL-Caltech Participants
• Lisa Pratt, Ph.D., NASA Headquarters
• Andy J. Spry, Ph.D., NASA Headquarters
• Chris Salvo, JPL-Caltech
• Brian Muirhead, JPL-Caltech
• Bob Gershman, JPL-Caltech
• Alvin L. Smith, Ph.D., PMP, JPL-Caltech
• Douglas M. Isbell, JPL-Caltech
• Wayne Schubert, JPL-Caltech
• Morgan Hendry, JPL-Caltech
• Mariko Burgin, JPL-Caltech
• Elizabeth Luthman, JPL-Caltech
• Victoria Ryan, JPL-Caltech
• J. Nick Benardini, Ph.D., JPL-Caltech
• Emily P. Seto, JPL-Caltech
• Richard Mattingly, JPL-Caltech
• Kelli McCoy, JPL-Caltech
• Michael DiNicola, JPL-Caltech
• Robert Lock, JPL-Caltech
• Amruta Mehta, JPL-Caltech
• Erin Lalime, Ph.D., NASA-GSFC
• Betsy Pugel, Ph.D., NASA-GSFC
• Thomas Randolph, JPL-Caltech
• Moogega C. Stricker, Ph.D., JPL-Caltech
• Brian Shirey, Ph.D., JPL-Caltech
• Jeff Umland, Ph.D., JPL-Caltech
• Charles Budney, JPL-Caltech
• Aaron B. Regberg, Ph.D., NASA-JSC
• Arturo Avila, JPL-Caltech
• Melissa Jones, Ph.D., JPL-Caltech
• Dan Glavin, Ph.D., NASA-GSFC
• Jason Dworkin, Ph.D., NASA-GSFC
ESA Participants
• Gerhard Kminek, Ph.D., ESA