Introduction
Brain stimulation has become an exciting development in neurosciences to understand the concepts of plasticity and to treat various brain disorders. Brain stimulation can be broadly classified as invasive (e.g., deep brain stimulation [DBS]) and noninvasive brain stimulation (NIBS) techniques. Although DBS plays a significant role in the treatment of several neurological and psychiatric disorders, it requires surgery and cannot be used in healthy subjects to understand brain physiology. Hence, in this narrative review, we discuss several NIBS techniques and their potential role in inducing as well as measuring brain plasticity. NIBS can broadly be classified as electrical, magnetic, or sonographic Reference Fomenko, Chen and Nankoo1 modes of stimulation. A representation of various modes of stimulation under these three subtypes is as depicted in Figure 1 and summarized in Table 1.
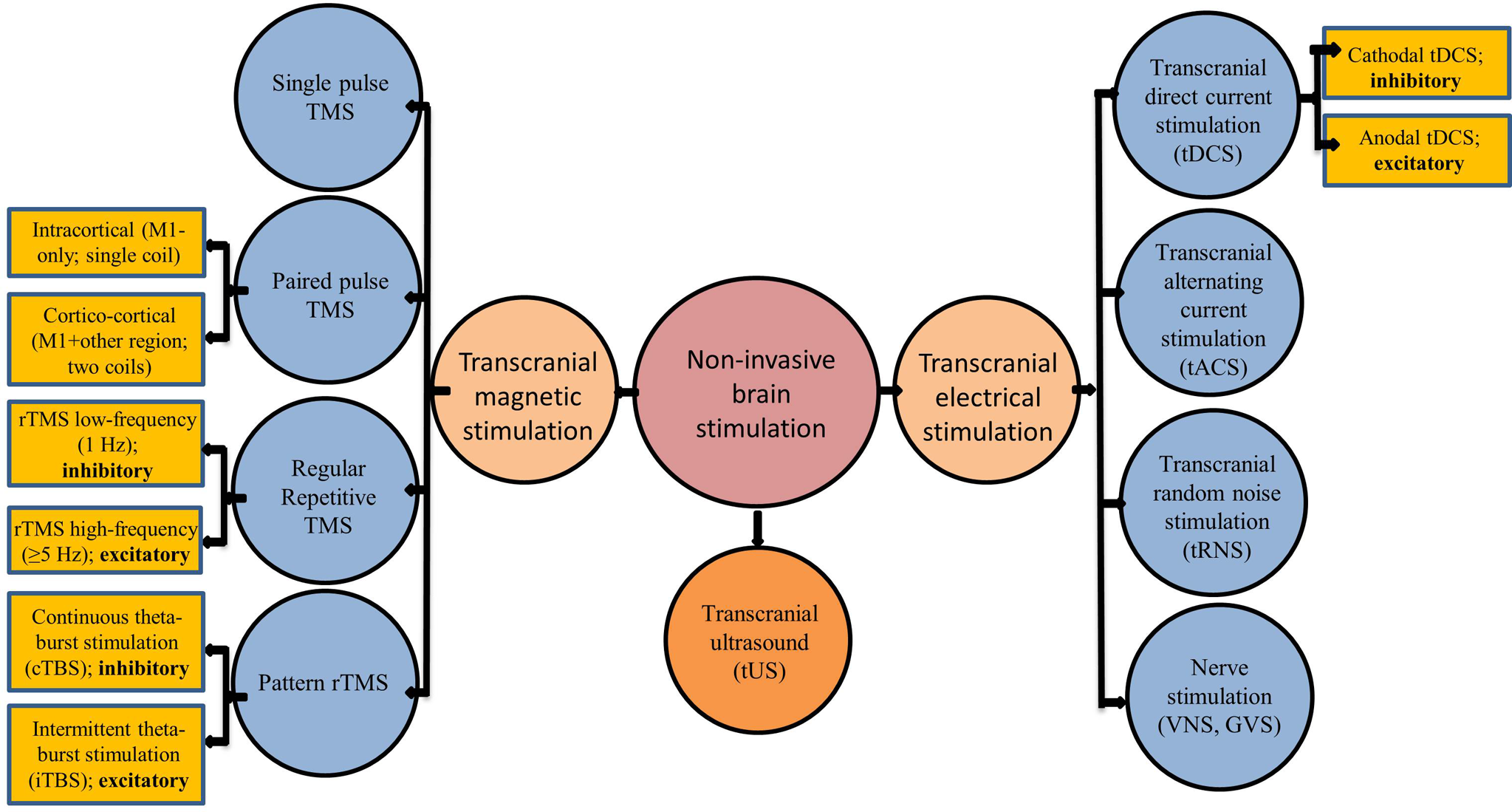
Figure 1: Different modes of noninvasive brain stimulation: GVS: Galvanic vestibular stimulation; tACS: transcranial alternating current stimulation; TBS: theta-burst stimulation; tDCS: transcranial direct current stimulation; TMS: transcranial magnetic stimulation; tRNS: transcranial random noise stimulation, TUS: transcranial ultrasound stimulation; VNS: vagal nerve stimulation.
Table 1: Key features of different modes of NIBS

GVS: galvanic vestibular stimulation; NIBS: noninvasive brain stimulation; TMS: transcranial magnetic stimulation; tACS: transcranial alternating current stimulation; tRNS: transcranial random noise stimulation; tDCS: transcranial direct current stimulation; taVNS: transcutaneous auricular vagal nerve stimulation.
Transcranial Magnetic Stimulation (TMS)
Basics of TMS
TMS is a noninvasive neurophysiological technique of stimulating the brain through the intact skull. Reference Barker, Jalinous and Freeston2 It has been widely used to access changes in cortical excitability associated with neurological and psychiatric disorders due to its ability to identify subtle deficits in brain inhibition and excitation. Reference Rossini, Burke and Chen3
TMS and Cortical Excitability
Single-pulse TMS can assess cortical excitability using parameters such as the amplitude of motor evoked potentials (MEPs), resting or active motor thresholds (RMT/AMT: minimal intensity to generate small MEP of specific amplitude when the target muscle is at rest or active, respectively), the silent periods (silence in the electromyography activity following magnetic stimulation generated MEP during the active contraction of the muscle of interest), recruitment curves (input–output curve, greater cortical activation with increasing stimulation intensity), and mapping of muscle representation in motor homunculus in the motor cortex (Figure 2). Using paired-pulse TMS, several inhibitory and facilitatory intracortical circuits such as short-interval cortical inhibitions (SICIs) and intracortical facilitations (ICFs) can be measured. Reference Chen, Cros and Curra4,Reference Agrawal, Bhattacharya, Kamble, Yadav and Pal5
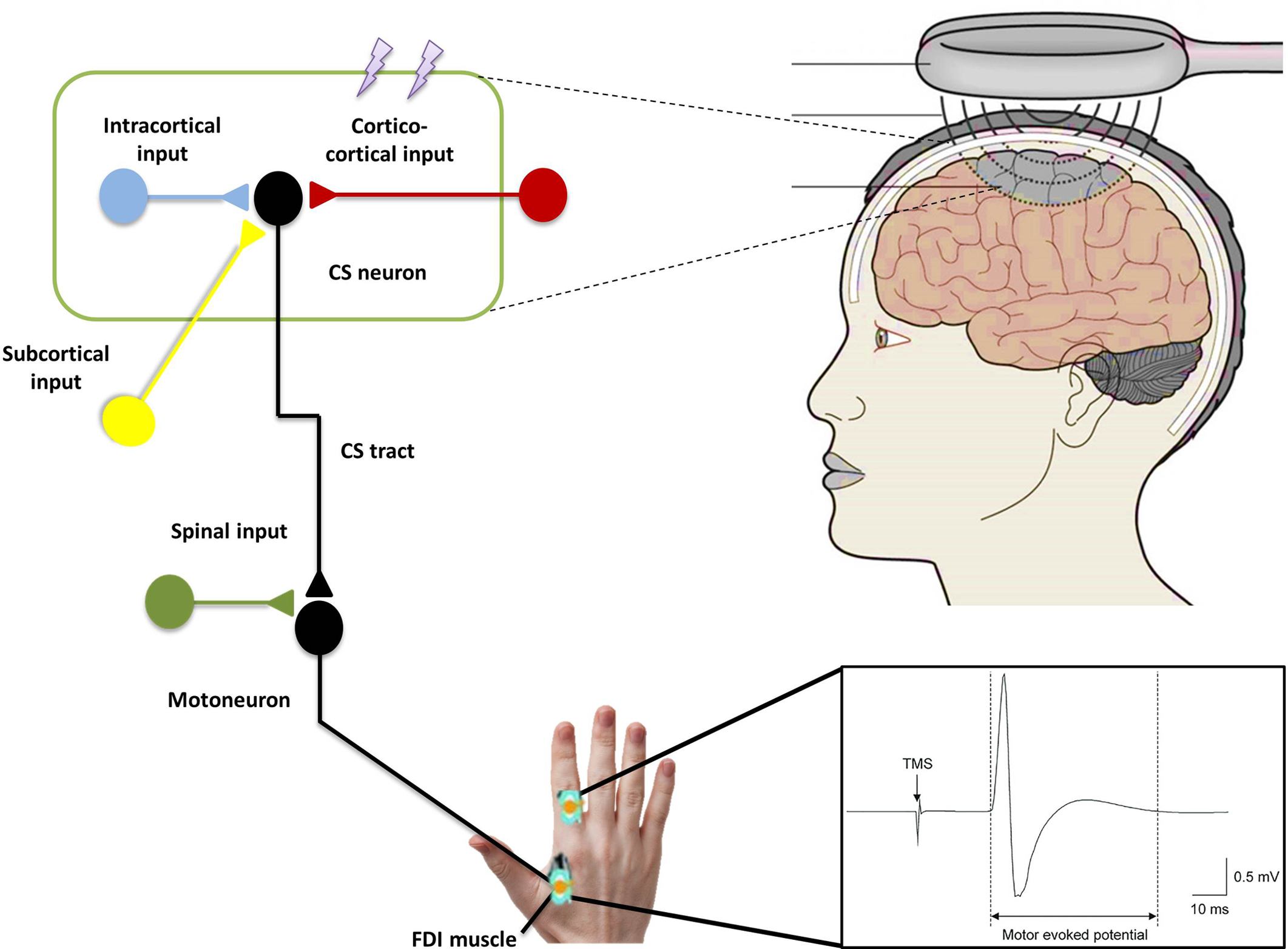
Figure 2: Schematic representation of the transcranial magnetic stimulation demonstrating the magnetic field generated with the magnetic coil placed over the hand area of the primary motor cortex. This, in turn, induces electrical current to activate cortical circuits (lightning bolts indicating the electromagnetic pulses) leading to activation of corticospinal neurons and subsequently and alpha motor neurons in the spinal cord that innervate the muscle of interest (first dorsal interosseous muscle). This leads to motor evoked potential recorded with surface electromyography.
Mechanistic Basis of TMS
A TMS device consists of a series of capacitors connected to a wire coil which has inductance as well as resistance. When a rapidly changing electrical current passes through a wire coil placed on the scalp, a changing magnetic field is generated which penetrates the cranium. This generates eddy currents which lead to action potentials in the neurons in the brain. Reference Rothwell6,Reference Mills7 The commonly used figure-of-eight coil is usually composed of two wires coiled side by side on the same plane passing currents in opposite directions. The figure-of-eight coil has better focality than other coils such as the circular coil because the point of maximum stimulation is at the junction of the two loops. Reference Ueno, Tashiro and Harada8 TMS administered over the motor cortex elicits a series of waves over corticospinal axons that can be recorded over the spinal cord, with the direct (D) wave followed by a series of indirect (I) waves. TMS at low intensities preferentially activates I waves which arises from the transsynaptic activation of corticospinal neurons and at higher intensities also evoke the D wave which represents direct stimulation of the corticospinal axon. Reference Terao and Ugawa9
Clinical Applications of Single and Paired TMS
TMS has been very helpful in diagnosing spinal cord compressions in patients with myelopathy. Central motor conduction time of the upper and lower limb correlated with the severity of cord compression with TMS having 100% sensitivity and 84.8% specificity. Reference Lo, Chan and Lim10 Patients with cerebellar impairment have increased RMT of the contralateral motor cortex. Reference Cruz-Martinez and Arpa11 In patients with Parkinson’s disease (PD), RMT was found to be decreased in very rigid patients and AMT was increased in chronic bradykinetic patients. Reference Cantello, Gianelli, Bettucci, Civardi, De Angelis and Mutani12,Reference Ellaway, Davey, Maskill and Dick13 MT is reduced in patients with tics and obsessive compulsive disorder. Reference Greenberg, Ziemann and Corá-Locatelli14 One study showed that SICI was reduced in PD patients and levodopa partly normalized this impaired inhibition. Reference Ridding, Inzelberg and Rothwell15,Reference Ammann, Dileone and Pagge16 Interestingly, a study showed that short-interval ICF, which is caused by summation of activation of different facilitatory interneurons in the M1, is increased in PD patients. Reference Ni, Bahl, Gunraj, Mazzella and Chen17 Studies have demonstrated that patients with upper limb dystonia, Reference Ridding, Sheean, Rothwell, Inzelberg and Kujirai18 cervical dystonia, Reference Kaňovský, Bareš, Streitová, Klajblová, Daniel and Rektor19 and blepharospasm have reduced SICI. Reference Sommer, Ruge, Tergau, Beuche, Altenmüller and Paulus20 Also, TMS can be combined with a wide range of brain mapping modalities such as magnetic resonance imaging (MRI) or magnetic resonance spectroscopy and has great scientific and clinical potential. Reference Siebner, Bergmann and Bestmann21–Reference Rossi, Antal and Bestmann24
Limitations of TMS
The extent of the induced current in the brain can be variable. It is not known which type of neurons are stimulated by TMS and whether the effects of TMS on the neurons are excitatory, inhibitory, or state dependent. Reference Ziemann25 Another limitation is that the TMS most affects the cortical structures. It is difficult to specifically target subcortical regions, and TMS pulse cannot stimulate subcortical areas without affecting cortical ones. Reference Bolognini and Ro26,Reference Sliwinska, Vitello and Devlin27
Summary of Single and Paired TMS
An important feature of magnetic stimulation that differentiates it from electrical stimulation is that the current induced flows parallel to the surface of coil in the brain. Different TMS techniques and parameters have demonstrated potential diagnostic utility with promising results. However, further studies with large sample sizes are needed to establish the sensitivity and specificity of the techniques in each condition.
Repetitive TMS (rTMS)
Basics of rTMS
The use of rTMS as a treatment was first described in 1993 as a treatment for drug-resistant major depression. Reference Höflich, Kasper, Hufnagel, Ruhrmann and Möller28 Since TMS indirectly activates pyramidal neurons of layer V of the motor cortex (M1) through interneurons in layers II and III, it is believed that rTMS triggers the same set of synaptic connections multiple times.
rTMS and Cortical Excitability
rTMS over the motor cortex leads to lasting changes in MEP amplitudes that persist post stimulation. Previous studies showed that high-frequency (>5 Hz) rTMS increases cortical excitability, whereas low frequency (1 Hz or lower) rTMS decreases cortical excitability. Reference Pascual-Leone, Gomez-Tortosa, Grafman, Alway, Nichelli and Hallett29,Reference Chen, Classen and Gerloff30 These changes are likely caused by synaptic long-term potentiation and depression (LTP/LTD) since effects of rTMS are blocked by administration of drugs that interfere with N-methyl-D-aspartate (NMDA) receptors, which are known to be involved in LTP/LTD processes. Reference Ziemann, Paulus and Nitsche31,Reference Hoogendam, Ramakers and Di Lazzaro32 The physiological effects of a single session of rTMS lasts around 30–60 min based on the protocol administered. Reference Hoogendam, Ramakers and Di Lazzaro32 Repeated rTMS administration leads to cumulative effects, depending on the number of sessions. Reference Bäumer, Demiralay and Hidding33,Reference Schulze, Feffer and Lozano34
Clinical Applications
For the treatment of psychiatric disorders such as depression, the remission rates were higher with longer duration of rTMS treatment (daily up to 6 weeks), and previous studies indicate that at least 20 to 30 sessions are needed to have optimal effects. Reference O’Reardon, Solvason and Janicak35,Reference Milev, Giacobbe and Kennedy36 rTMS also induced changes in the functional and structural connectivity of the associated networks. Reference Anderson, Hoy, Daskalakis and Fitzgerald37 In addition to the frequency of rTMS, the number of pulses is also important. In a study in patients with tinnitus, patients who received 6000 pulses did not improve but patients who received 12,000 pulses reported a beneficial effect on tinnitus. Reference Park, Noh and Lee38 Theta-burst stimulation (TBS) protocol involves magnetic pulses dispensed in bursts of three pulse at high frequency (50 Hz) with an interburst interval of 200 ms or 5 Hz (i.e., at the theta frequency). Reference Huang, Edwards, Rounis, Bhatia and Rothwell39 A meta-analysis suggested TBS may be an effective treatment for depression. Reference Berlim, McGirr, Rodrigues dos Santos, Tremblay and Martins40 A non-inferiority study showed similar efficacy of intermittent TBS (iTBS) and 10 Hz rTMS in the treatment of depression, but TBS has the advantage that it can be administered in a much shorter time. Reference Blumberger, Vila-Rodriguez and Thorpe41 Both high-frequency rTMS and the theta-burst TMS of the left dorsolateral prefrontal cortex (DLPFC) have been approved by the United States Federal Drug Administration and Health Canada for treatment of medication-resistant depression. Reference Blumberger, Vila-Rodriguez and Thorpe41,Reference Lefaucheur, Aleman and Baeken42 The therapeutic use of rTMS is usually as add-on treatment, especially for refractory symptoms. A consensus panel reported that TMS can be recommended for treatment of major depressive disorder, neuropathic pain (NP), post-traumatic brain injury-related headaches, postoperative pain, and migraine. Reference Lefaucheur, Aleman and Baeken42,Reference Leung, Shirvalkar and Chen43 However, many conditions such as NP, motor symptoms of PD, stroke, and its complications: dysphagia, aphasia, hemispatial neglect, multiple sclerosis, tinnitus, schizophrenia, obsessive-compulsive disorder showed promising therapeutic evidence as shown by Class II and III studies or level B recommendation. Reference Blumberger, Vila-Rodriguez and Thorpe41
Adverse Effects of rTMS
Although very rare, TMS can induce seizures under ordinary clinical use in 1 out of 30,000 treatments. Reference Carpenter, Janicak and Aaronson44 According to the safety guidelines of Rossi et al. (2021), stimulation parameters within the safety guidelines usually have minimal side effects. Reference Rossi, Antal and Bestmann24 An interesting observation that even the highest intensity (within the technical limitations of the machine) of TBS could not induce seizures in a series of eight patients in an epilepsy monitoring unit. Reference Udupa, Tai and Saha45 Syncope is another potential side-effects of rTMS especially in patients with high degree of anxiety, dehydration, or hypoglycemia. Reference McClintock, Reti and Carpenter46
Summary of rTMS
rTMS is a safe, noninvasive NIBS therapy which is being increasingly incorporated into clinical practice. Additional research to test the therapeutic application of rTMS in neurological and psychiatric disorders is warranted.
Transcranial Direct Current Stimulation (tDCS)
Basics of tDCS
tDCS is a commonly used NIBS technique that stimulates the brain using electric current (typically 1–2 mA) through the electrodes placed on the scalp (as anode and cathode; Figure 3A).
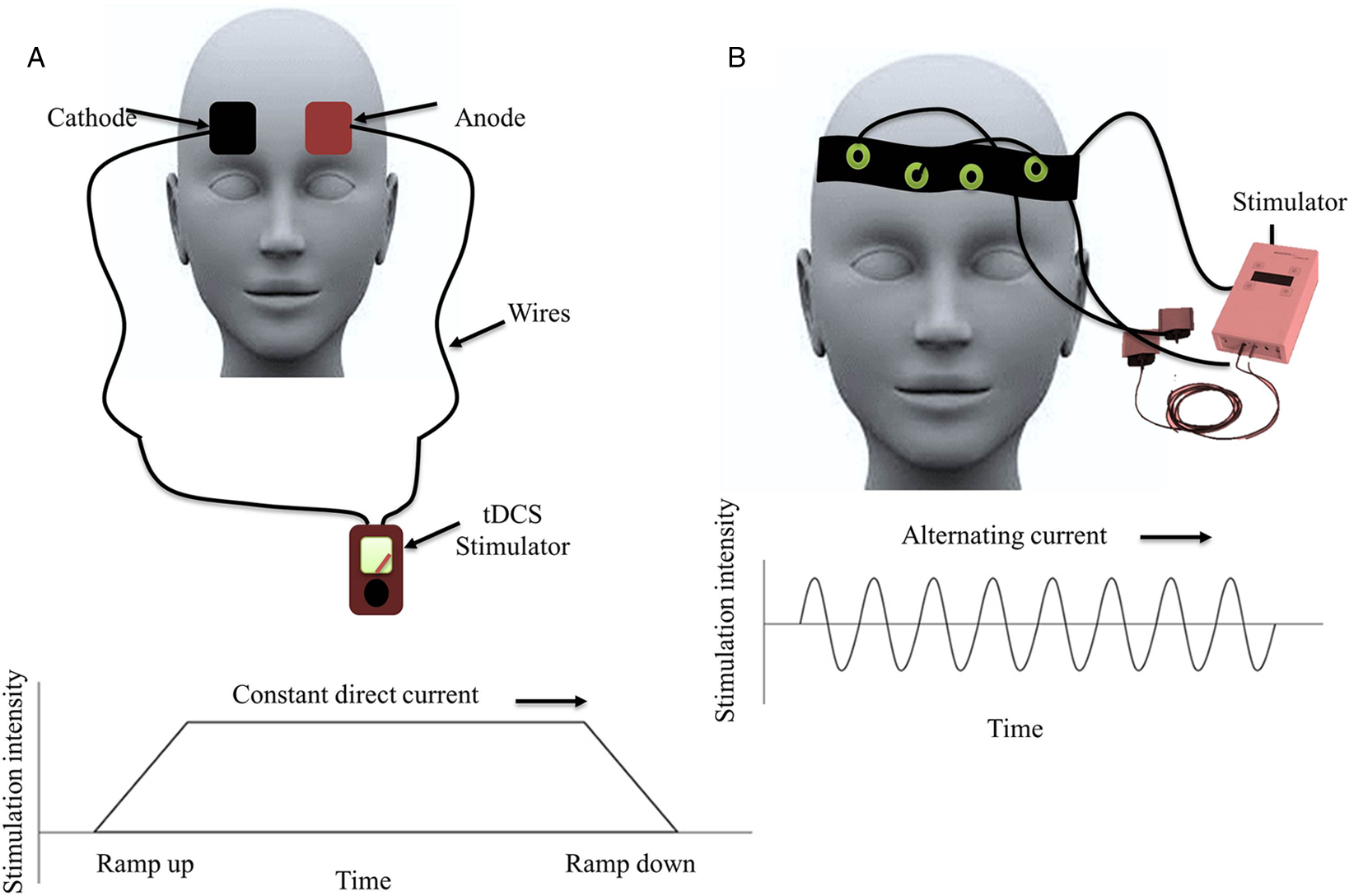
Figure 3: Schematic representation of the transcranial direct (tDCS) and alternating (tACS) current stimulation: (A) tDCS: transcranial direct current stimulation showing anodal and cathodal electrodes placed over bifrontal regions and the graph below plots stimulation intensity overtime demonstrating that the intensity ramps up and down, and the intensity provided over the stipulated time. (B) tACS: transcranial alternating current stimulation showing headband containing electrodes and the graph below shows that the stimulation intensities vary in a sinusoidal manner overtime with the alternating polarity of current applied.
Changes in Brain Excitability with tDCS
Studies have demonstrated that anodal tDCS (positive stimulation) increases cortical excitability likely due to depolarization of the resting membrane potential that increases neuronal excitability, leading to increased spontaneous neuronal firing. On the other hand, cathodal tDCS (negative stimulation) reduces cortical excitability, likely related to hyperpolarization of the resting membrane potential. Reference Nitsche, Cohen and Wassermann47,Reference Nitsche and Paulus48 The efficacy of these changes depends on the duration of stimulation. Reference Stagg, Antal and Nitsche49 Animal studies in the 1960s had demonstrated that a few minutes of direct current (DC) stimulation of the sensorimotor cortex caused a prolonged change in neuronal activities for 1–5 h. Reference Bindman, Lippold and Redfearn50 Glutamatergic synapses are involved in DC induced plasticity, especially with the involvement of NMDA receptors. Pharmacological studies in humans have revealed that blockade of NMDA receptors with dextromethorphan prevents anodal and cathodal tDCS induced plasticity. Reference Nitsche, Fricke and Henschke51 Moreover, NMDA receptor agonist D-Cycloserine led to an increase in anodal tDCS induced excitability. Reference Nitsche, Jaussi, Liebetanz, Lang, Tergau and Paulus52
Mechanistic Basis of tDCS
The influx of calcium ions (Ca2+) into the cell due to the activation of NMDA receptors is needed for the generation of LTP and LTD as demonstrated in animal slice preparation studies using patch-clamping techniques. Several neurotransmitters are involved in the induction of LTP and LTD. With a rapid rise in postsynaptic Ca2+ concentration, Ca2+ binds to the C-terminal of calmodulin which activates the kinase pathways, resulting in an increase of α-amino-3-hydroxy-5-methyl-4-isoxazolepropionic acid (AMPA) receptor density on the postsynaptic membrane leading to LTP. On the other hand, with a slower increase in postsynaptic Ca2+ concentration, Ca2+ binds to the N-terminal of calmodulin activating the phosphatase pathways, resulting in a decrease of AMPA receptor density on the postsynaptic membrane, leading to LTD (Figure 4). Reference Rossi, Antal and Bestmann24,Reference Udupa and Chen53
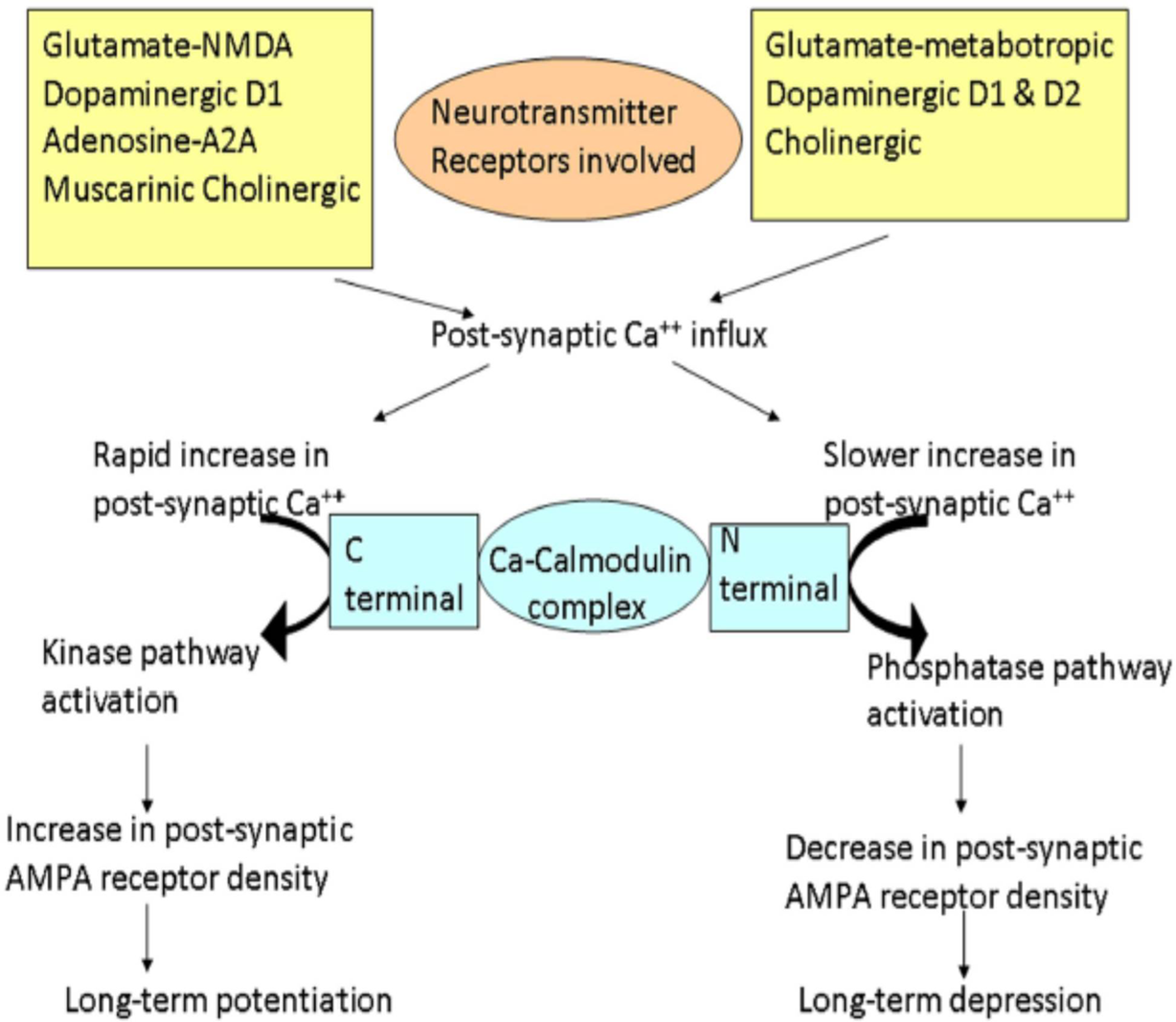
Figure 4: Schematic representation of the cascades of events involved in long-term potentiation (LTP) and depression (LTD). Different neurotransmitters are involved in these cascades. Different neurotransmitter and their receptors are shown in yellow rectangular boxes. Glutamate acting through N-methyl D-Aspartate, dopamine through D1, adenosine through A2A, and acetylcholine through muscarinic receptors leads to LTP. On the other hand, glutamate acting through metabotropic receptors, dopamine through both D1 and D2 receptors, and cholinergic activation lead to LTD. Different changes occur depending on the rate of increase of postsynaptic calcium (Ca2+). The rapid influx of Ca2+ preferentially promotes binding of Ca2+ to the C-terminal of calmodulin, activating the kinase pathways. These reactions lead to an increase in AMPA receptor density on the postsynaptic membrane resulting in LTP. On the other hand, slower release of Ca2+ leads to Ca2+ binding to the N-terminal of calmodulin, activating the phosphatase pathways. This leads to a decrease in AMPA receptor density on the postsynaptic membrane, resulting in LTD. (Adapted from Udupa K and Chen R, Motor Cortical Plasticity in Parkinson’s Disease, Front. Neurol. 4 [2013].)
Duration and strength of stimulation were too long (≥25 min) or too strong (≥2 mA) can change the direction of the tDCS effects. Reference Udupa and Chen53–Reference Jamil, Batsikadze and Kuo56 These changes are presumed to occur because of calcium ion changes in the postsynaptic cells due to changes in membrane polarity. However, other neurotransmitters and neuromodulators are also involved such as acetylcholine, dopamine, and serotonin, and they may play important roles. Reference Fritsch, Reis and Martinowich57,Reference Nitsche, Müller-Dahlhaus, Paulus and Ziemann58
For example, dopamine blockers prevent plasticity induction by tDCS. Reference Nitsche, Lampe and Antal59,Reference Márquez-Ruiz, Leal-Campanario and Sánchez-Campusano60 Therefore, these neurotransmitters affect plasticity induction. Reference Nitsche, Müller-Dahlhaus, Paulus and Ziemann58 In summary, tDCS induces calcium-dependent plasticity at glutamatergic synapses, which is gated by the reduction of gamma-aminobutyric acid (GABA) activity. Reference Fritsch, Reis and Martinowich57,Reference Nitsche, Müller-Dahlhaus, Paulus and Ziemann58
Clinical Applications
Studies have reported that anodal tDCS over the DLPFC improves cognitive functions as well as emotional processes in patients with major depression. Reference Fregni, Boggio, Nitsche, Marcolin, Rigonatti and Pascual-Leone61 In patients with bipolar or unipolar depression, five tDCS sessions of 20 min each with anodal stimulation over the left DLPFC led to a reduction in depressive symptoms after the fifth session. Reference Brunoni, Ferrucci and Bortolomasi62 In patients with schizophrenia refractory to antipsychotic drugs, auditory verbal hallucinations were reduced by tDCS relative to sham stimulation as indicated by the Positive and Negative Syndrome Scale. Reference Brunelin, Mondino and Gassab63
Apart from improving psychiatric symptoms, tDCS administered bilaterally over the cerebellar cortex to treat neurodegenerative ataxic symptoms has shown significant improvement based on the Scale for the Assessment and Rating of Ataxia, the International Cooperative Ataxia Rating Scale, the 9-Hole Peg Test, and the 8-Meter Walking Time. These results suggested that a single session of anodal tDCS over the cerebellar cortex may enhance motor performance, and improve motor and upper limb coordination in patients with neurodegenerative ataxia. Reference Benussi, Koch, Cotelli, Padovani and Borroni64 Another study reported that 10 sessions of anodal tDCS over the cerebellar area improved symptoms for 3 months and modulated cerebello-motor connectivity measured by cerebellar inhibition in patients with cerebellar ataxia. Reference Benussi, Dell’Era and Cotelli65
Previous studies demonstrated that the benefits of tDCS may require multiple sessions. In a study in patients with fibromyalgia, Reference Carvalho, Brietzke and Gasparin66 a home-based tDCS device that permits daily use for 20 min with a minimal interval of 12 h between session was used and suggested that a portable device for home use is feasible with proper monitoring of adherence and contact impedance. Reference Carvalho, Brietzke and Gasparin66 Studies have also investigated the accessibility of tDCS on patients with PD using a remotely supervised tDCS protocol paired with cognitive training to reduce clinician, patient, and caregiver burden. It was found that remotely supervised tDCS was safe, tolerable with minimal side effects such as mild sensations of transient itching, and patients with PD reported improvement of fatigue as well as increased cognitive performance. Reference Dobbs, Pawlak and Biagioni67
Side Effects and Limitations of tDCS
A study summarized the adverse effects of tDCS sessions over motor and non-motor cortical areas in healthy subjects, neurological, and psychiatric patients. The results demonstrated that during an around 18,000 sessions of tDCS administration, no serious adverse events have been reported. Moderate adverse events were rare; however, skin burns due to suboptimal electrode-skin contact have been reported. Rarely cases such as mania or, hypomania were induced in patients with depression. Fatigue and headache were also reported followed by light itching and burning sensation under the stimulation electrodes. Reference Antal, Alekseichuk and Bikson68 Skin redness has also been reported. Reference Frank, Wilfurth, Landgrebe, Eichhammer, Hajak and Langguth69,Reference Palm, Reisinger and Keeser70 The low spatial resolution and difficulty in precisely localizing the electric field current in the brain are some of the limitations of tDCS. It is to be noted that the inability to target deeper structures except superficial cortex is a limitation for most NIBS techniques except for transcranial ultrasound stimulation (TUS).
Summary of tDCS
tDCS is an NIBS technique which has been applied in psychiatric and neurological disorders with some promising results. While there are a large number of tDCS studies, the mechanisms and neural correlates underlying tDCS are not fully understood. The behavioral and neural effects of different montages of tDCS should be systematically tested.
Transcranial Alternating Current Stimulation (tACS)
Basics of tACS
tACS is an NIBS technique which delivers oscillating electrical currents to the brain. Reference Antal, Boros, Poreisz, Chaieb, Terney and Paulus71–Reference Guerra, Suppa and Bologna73 tACS is a variant of tDCS, was designed to modulate the brain oscillations and the cognitive functions, and to serve as a therapeutic tool in restoring dysfunctional cortical oscillations in neurological disorders. Reference Krause, Wach, Südmeyer, Ferrea, Schnitzler and Pollok74–Reference Ahn, Mellin and Alagapan78 tACS involves the application of sinusoidal current between two electrodes where current and polarity alternates according to the sine wave pattern. Reference Antal, Boros, Poreisz, Chaieb, Terney and Paulus71,Reference Antal and Herrmann79 tACS can be applied in any frequency. Previous studies have used a range from DC to 5 kHz for induction of sustained changes in cortical excitability Reference Chaieb, Antal and Paulus80 and 200 kHz for tumour therapy Reference Kirson, Dbalý and Tovaryš81 (Figure 3B).
Entrainment
The main effect of tACS is to modulate and entrain ongoing rhythmic brain activities. Reference Kuo, Polanía, Nitsche, Brunoni, Nitsche and Loo82 Studies in nonhuman primates have demonstrated that tACS affect the timing of neuron spiking activity in the region targeted. Reference Krause, Vieira, Csorba, Pilly and Pack83 A study performed in young and older adults stimulated at the individual alpha frequency for 10 min (1.5 mA) over the left motor cortex to understand the relationship between effects of tACS and electroencephalography (EEG) alpha power, which decreases with ageing. Although tACS increased motor cortical excitability (increased MEP amplitude) in both groups, short-interval intracortical inhibition (SICI, a measure of GABA-A receptor-mediated inhibitory circuit) decreased in young subjects but increased with older subjects. Since the increase in cortical excitability was similar in both groups, there is no indication that tACS was more effective in the elderly population i.e., the group with lower alpha power. Reference Fresnoza, Christova and Feil84 Another study applied tACS at the alpha frequency (8–12 Hz) for 11–15 min in four sessions with intermittent or sham tACS to investigate as to what extent plasticity can account for tACS aftereffects when controlling for entrainment “echoes” (an entrained activity that remains stable after the end of rhythmic stimulation). The study used successive tACS events which were either phase continuous or phase discontinuous for short (3 s) or long (8 s) duration. The study suggested that α-tACS can be used as a therapeutic tool with induction of short term neural plasticity rather than entrained activity. Reference Vossen, Gross and Thut85 Previous studies have demonstrated that tACS applied at an alpha frequency range resulted in enhancement of EEG amplitude for 10–30 min after stimulation. These studies indicate that tACS modulates spontaneous cortical oscillatory activity. Reference Zaehle, Rach and Herrmann86,Reference Neuling, Rach and Herrmann87 Furthermore, synaptic modifications from exposure to the alternating electrical field might alter neurochemical mechanisms such as calcium entry in the presynaptic terminals that can lead to short-term synaptic plasticity. Reference Citri and Malenka88
tACS and Behavioural Changes
tACS over the M1 may improve cortical functions as identified by the improved cognition compared with sham or no stimulation. Reference Paulus, Nitsche and Antal89 tACS has been used to modulate the M1 when applied within the beta (13–30 Hz) or the high-gamma (60–90 Hz) frequency ranges during a visuomotor task and concurrent functional MRI. The results showed that tACS not only changed neural activities underneath the stimulation electrode but also led to compensatory modulation within connected and functionally related brain networks. Reference Pogosyan, Gaynor, Eusebio and Brown90,Reference Moisa, Polania, Grueschow and Ruff91 Furthermore, tACS improves motor performance which correlated with increased synchronization of the gamma frequency band in M1. Reference Moisa, Polania, Grueschow and Ruff91,Reference Joundi, Jenkinson, Brittain, Aziz and Brown92 A study co-stimulated the M1 in healthy subjects with tACS during iTBS which is known to induce LTP-like plasticity to determine whether gamma tACS on iTBS-induced plasticity are related to changes in GABA-A receptor-mediated interneuronal activity. Reference Guerra, Suppa and Bologna73 Gamma frequency tACS but not beta frequency tACS increased as well as prolonged iTBS-induced LTP-like plasticity in the human M1, indicating a link between gamma oscillations, interneuronal GABA-A-ergic activity, and LTP-like plasticity in the human M1. Reference Guerra, Suppa and Bologna73 A study examined the aftereffects of tACS (10 and 20 Hz) of the parietal cortex on bimanual coordination. The participants received 10 and 20 Hz tACS, or sham stimulation of 1 mA at the parietal brain areas (P3/P4 positions) for 20 min. No specific effect of tACS on the bimanual coordination task was observed. However, there was a rise in the parietal alpha activity following the 20 Hz tACS in the right parietal area which was accompanied by decreased oxygenated haemoglobin (Hb) concentration in the right motor cortex as measured by functional near-infrared spectroscopy. Thus, tACS affects cortical physiology. Reference Berger, Pixa, Steinberg and Doppelmayr93 The behavioral effects of tACS have been reported in many studies. tACS has been found to modulate auditory perception as thresholds of detection were dependent on the phase of the oscillation that was entrained by α-tDCS, establishing a correlation between stimulation phase and modulation of perception. Reference Neuling, Rach, Wagner, Wolters and Herrmann94 tACS applied at 0.75 Hz frequency to subjects during early nocturnal non-rapid-eye-movement sleep enhanced EEG delta activity resulting in the improved recall of memory the next morning. Reference Marshall, Helgadóttir, Mölle and Born95 Another study found that left parietal tACS at theta frequency improved performance on working memory of an attention task along with decrement in P300 latency in the left hemisphere. Reference Jaušovec and Jaušovec96 Multiple studies on tACS suggested that cross-frequency phase-amplitude coupling of theta and gamma oscillations play a role in neuronal computation, communication, and learning. Reference Mormann, Fell and Axmacher97,Reference Demiralp, Bayraktaroglu and Lenz98
Clinical Applications
tACS can modulate impaired oscillatory patterns such as in PD leading to reduced tremor amplitude Reference Brittain, Probert-Smith, Aziz and Brown99 and attenuation or resetting pathological oscillations in schizophrenia. Reference Ahn, Mellin and Alagapan78 Patients with PD have been found to have reduced γ oscillatory activity in the basal ganglia-thalamo-cortical network, Reference Anzak, Tan and Pogosyan100,Reference Florin, Erasmi and Reck101 and plasticity induced by iTBS is reduced in these patients. Reference Suppa, Marsili and Belvisi102 A recent study showed that iTBS induced plasticity in PD patients was normalized when iTBS was applied during tACS at γ frequency. Reference Guerra, Asci and D’Onofrio103 Furthermore, tACS administered over 3 months to the orbitofrontal cortex personalized to the intrinsic beta gamma frequency in patients with obsessive compulsive disorder modulated reward learning and improved obsessive compulsive behavior. Reference Grover, Nguyen, Viswanathan and Reinhart104 A study used 200-kHz tACS in patients with recurrent glioblastoma suggested that this type of stimulation may inhibit the growth of this treatment-resistant tumor, with little or no side effects. Reference Kirson, Dbalý and Tovaryš81 Another study applied tACS of the cerebellum phase locked to the tremor in patients with essential tremor and showed that tremor suppression was due to disruption of temporal coherence of the aberrant oscillations in the olivocerebellar loop. Reference Schreglmann, Wang and Peach105
Side Effects of tACS
The most common side effects in tACS studies are nausea, discomfort, and twitching. tACS administered over the motor cortex can lead to perception of phosphenes or flashes in the subjects’ visual field. Reference Antal, Boros, Poreisz, Chaieb, Terney and Paulus71 Phosphene perception peaks at stimulation frequencies between 10–20 Hz. Reference Kanai, Chaieb, Antal, Walsh and Paulus106 Posterior montages with frequency of 4 Hz have caused dizziness due to stimulation of the vestibular nerve. Reference Raco, Bauer, Olenik, Brkic and Gharabaghi107
Summary of tACS
tACS acts by modulating ongoing brain rhythms and altering neuronal properties and networks. While tACS is the only method of modulating the individual alpha frequency, larger studies to validate results are required due to variability in the response to tACS protocols. Despite advances in the field of neuromodulation, further studies are need to unravel the effects of tACS at multiple levels from molecular to animal neurophysiology and to clinical applications. It is also important to explore the utility of this technique in understanding the aberrations in neuronal networks in neurological and psychiatric disorders and to use this technique effectively to bring about optimal clinical benefits.
Transcranial Random Noise Stimulation (tRNS)
Basics of tRNS
tRNS is a unique kind of tACS where low-intensity alternating current is administered with randomized intensity and frequency. Reference Antal and Herrmann79 tRNS has so far been explored within the frequency spectrum of 0.1–640 Hz (full spectrum) or 101–640 Hz (high-frequency stimulation) with a “white noise” characteristic. Reference Terney, Chaieb, Moliadze, Antal and Paulus108,Reference Fertonani, Pirulli and Miniussi109 The probability function of the RNS is a Gaussian curve with zero mean and a variance. In most studies, the current intensity used to stimulate is around 1 mA. Reference Antal and Herrmann79 The physiological mechanisms of tRNS remain unclear. Higher frequencies of tRNS are supposed to modulate brain activity. However, due to the neuronal membrane acting as a low pass filter, a very small amount of current reaches the neuron with high frequencies tRNS. For example, AC electric fields at 1 V/m (max) in the brain at 100 Hz can polarize neurons by only 50 μV. Reference Deans, Powell and Jefferys110 However, this small change in many connected neurons can provide amplification of stimulation leading to physiological effects. Reference Fröhlich and McCormick111,Reference Reato, Rahman, Bikson and Parra112 The effect of tRNS could be contingent on other mechanisms such as stochastic resonance. Reference Stacey and Durand113 Stochastic resonance is a ubiquitous and conspicuous phenomenon—where a weak signal is amplified by adding noise to exceed its threshold of stimulation. Reference Moss, Ward and Sannita114 tRNS might increase neural firing by amplifying subthreshold oscillatory activity, also leading to decrement in the endogenous noise. This improved signal to noise ratio in the brain could lead to increased perception or cognition. Reference Moss, Ward and Sannita114–Reference Miniussi, Harris and Ruzzoli116
tRNS and Cortical Excitability Changes
tRNS administered over the primary motor cortex (M1) for 10 min with 1 mA application of full-spectrum or high-frequency transcranial random noise (hf-tRNS) elevated cortical excitability for 1–1.5 h. Reference Terney, Chaieb, Moliadze, Antal and Paulus108 Five to six minutes of stimulation also showed significant facilitation but with shorter duration of plasticity post stimulation. Reference Chaieb, Paulus and Antal117 In another study, it was observed that unilateral M1-tRNS enhanced motor learning. Reference Prichard, Weiller, Fritsch and Reis118 tRNS at low intensity of 0.4 mA had greater inhibitory aftereffects compared with cathodal tDCS at 1 mA or 140-Hz tACS at 0.4 mA, which suggests that inhibitory neurons might have lower thresholds for tRNS. Reference Moliadze, Atalay, Antal and Paulus119 In healthy subjects, it was noted that tRNS had an immediate effect of increased MEP amplitude with a duration of 40 min post stimulation, whereas anodal tDCS induced a gradual increase until 60 min following stimulation. Reference Laczó, Antal, Rothkegel and Paulus120 tRNS applied over the lateral occipital cortex was found to facilitate facial identity perception. Reference Romanska, Rezlescu, Susilo, Duchaine and Banissy121 hf-tRNS over the auditory cortex either unilaterally or bilaterally during a verbal dichotic listening task led to a significant increase in right ear advantage during bilateral hf-tRNS compared with sham stimulation. Reference Prete, D’Anselmo, Tommasi and Brancucci122
Clinical Applications
A study reported that tRNS over the temporoparietal cortex in patients with nonpulsatile tinnitus reduces tinnitus loudness when low frequency-tRNS (0.1–100 Hz) or hf-tRNS (100–640 Hz) were applied. Reference Joos, De Ridder and Vanneste123 A study investigated the efficacy of tRNS over the left DLPFC on attention and NP in patients with multiple sclerosis and reported that tRNS decreased the N2–P2 amplitudes of pain-related evoked potentials and improved pain ratings. Reference Palm, Chalah and Padberg124 However, there were no improvements in attention and mood scales.
Side Effects of tRNS
Although tRNS is a safe technique, burns on the skin where the electrode was applied have been reported. tRNS can cause headache in subjects. Reference Antal, Alekseichuk and Bikson68
Summary of tRNS
tRNS is a painless, noninvasive, as well as reversible neuromodulation that can temporarily enhance the excitability of the cortex. The high-frequency tRNS can increase cortical excitability. Reference Moret, Donato, Nucci, Cona and Campana125 However, the side effects are generally mild and temporary. Reference Lema, Carvalho, Fregni, Gonçalves and Leite126 Also, multiple sessions are required to produce clinical benefit. Larger sample size studies with standardized protocols are required to validate the results of earlier studies.
Transcutaneous Auricular Vagus Nerve Stimulation (taVNS)
Basics of taVNS
The vagus nerve (10th cranial nerve) is a mixed parasympathetic nerve which is an important component of the autonomic nervous system. It plays a key role in several body functions including swallowing, heart rate control, speech, respiratory control, gastric secretion, as well as intestinal motility. Reference Breit, Kupferberg, Rogler and Hasler127 The vagus nerve can be stimulated in two different ways: by a direct invasive stimulation (most frequent application) or by an indirect transcutaneous noninvasive stimulation (Figure 5). Vagus nerve stimulation (VNS) is a common invasive neuromodulation approach with a pulse generator implanted below the clavicle, and the lead is wrapped around the vagus nerve in the carotid sheath. Reference González, Yengo-Kahn and Englot128 VNS therapy reduces seizure frequency and improves the quality of life in patients with epilepsy. Reference Englot129 Previous studies have applied VNS therapy in patients with partial seizures. A study found that high-frequency VNS led to least 50% reduction in seizure frequency and low-frequency VNS also led to a 50% reduction in seizure frequency after 14 weeks. Reference Ben-Menachem, Mañon-Espaillat and Ristanovic130 Another study found that high-frequency VNS resulted in an average reduction of 28% in total seizure frequency compared with a 15% reduction in the low-frequency stimulation group. Reference Handforth, DeGiorgio and Schachter131 Invasive VNS is a safe and efficacious therapeutic technique and newer VNS systems have the advantage of minimizing VNS related adverse events such as infection and vocal cord paresis. Reference Ben-Menachem, Mañon-Espaillat and Ristanovic130,Reference Schulze-Bonhage132

Figure 5: Illustration of transcutaneous auricular vagal nerve stimulation showing the locations of the stimulating electrodes over the auricle which send impulses to the brainstem, frontal, parietal, and other subcortical regions through the auricular branch of the vagus and its neuronal connections. The stimulation current figure indicates the stimulation parameters, and the bottom figure shows a portable stimulator which generates the impulses with specific stimulation parameters with a wireless design.
The outer ear of a human is supplied by three sensory nerves: the auriculo-temporal nerve, the great auricular nerve, and the auricular branch of the vagus nerve (ABVN) (Figure 2). Reference Peuker and Filler133 taVNS is a relatively new method of noninvasive neural stimulation which targets the cutaneous receptive field of the ABVN at the outer ear. Reference Ellrich134 It has been introduced as an alternative to the invasive VNS procedure. Reference Kreuzer, Landgrebe and Husser135 taVNS is given at 10–25 Hz with a pulse width of 250–500 μs. The amplitude of stimulation varies from 0.25 to 10 mA depending on the experimental protocol, and some groups investigated different intensities as a function of individual perceptual threshold. Reference Busch, Zeman, Heckel, Menne, Ellrich and Eichhammer136–Reference Badran, Yu and Adair138 taVNS has been reported to increase heart rate variability (HRV), suggestive of a shift in cardiac autonomic function toward parasympathetic predominance. Thus, taVNS can influence human autonomic balance and provides an alternative to invasive VNS. Reference Clancy, Mary, Witte, Greenwood, Deuchars and Deuchars139
taVNS and Behavioural Changes
taVNS was used to test the causal relationship between VNS and the flow (pleasant psychological state of mind that individuals tend to experience when completely absorbed into specific action) measured using the Flow Short Scale. It was found that active compared with sham taVNS decreased the flow (as indexed by absorption scale scores), suggesting that the vagus nerve and the noradrenergic system are causally involved inflow. Reference Colzato, Wolters and Peifer137 To complete a complex task, different responses need to be prioritized and organized into different actions. A single-blind, sham-controlled study that assessed online (i.e., stimulation overlapping with the critical task) effects demonstrated that taVNS led to faster response selections during multiple tasks and helped in cascading different actions, and vagal stimulation might have improved performance in action cascading by modulating the noradrenergic and GABA systems. Reference Steenbergen, Sellaro, Stock, Verkuil, Beste and Colzato140 taVNS has also been found to increase the efficiency of action cascading measured by a stop-change paradigm. Reference Steenbergen, Sellaro, Stock, Verkuil, Beste and Colzato140 An interesting study investigated whether taVNS can reduce negative thought intrusions in high worriers. Participants exposed to taVNS reported less negative thought intrusions. Reference Burger, Van der Does, Thayer, Brosschot and Verkuil141 To examine whether taVNS affects autonomic modulation and spontaneous cardiac baroreflex sensitivity (cBRS), a study showed decreased resting HRV and increased cBRS, indicating modulation of autonomic functions by taVNS. Reference Antonino, Teixeira and Maia-Lopes142 A recent study found that participants who received taVNS tend to have lower declarative fear during fear extinction. Reference Burger, Van Diest and Van der Does143
Clinical Applications
A study in patients with unilateral, nonpulsatile chronic tinnitus applied taVNS over 2 weeks (pulse width, 200 μs; frequency, 30 Hz; stimulation sites [in sequence], the cavum, cymba, and the outer surface of the tragus; stimulation duration 4 min for each site) found that taVNS can be used to improve tinnitus. A ball-type electrode was placed on the stimulation site with the intensity increased by 1 mA every 5 s as long as the patients could tolerate without pain. The study found that tinnitus distress level decreased and a positive correlation between the level of tinnitus distress and the initial stimulus intensity of taVNS was observed. Reference Suk, Kim, Chang and Lee144 In a randomized, sham-controlled pilot study in patients with major depression, taVNS was administered for 15 min once or twice a day, five days per week, for two weeks. The Beck Depression Inventory scores improved with taVNS compared with sham stimulation. Reference Hein, Nowak and Kiess145 A study in patients with chronic stroke administered taVNS combined with robotic rehabilitation to enhance upper limb functions and reported a slight improvement in the patients post-intervention. Reference Capone, Miccinilli and Pellegrino146 taVNS has been applied to patients with drug-resistant epilepsy in a randomized, double-blinded, controlled trial. There was a significant decrease in seizure frequency after 20 weeks in the active treatment (25 Hz stimulation) group compared with the control (1 Hz) group. Reference Hamer and Bauer147 taVNS has also been tested on a randomized, double-blinded pilot study in PD patients with gastrointestinal complaints. The authors reported that scores of the Gastrointestinal Symptom Rating Scale improved post taVNS in the real stimulation but not in the sham stimulation group. Reference Kaut, Janocha, Weismüller and Wüllner148
Side Effects and Limitations of taVNS
The side effects of taVNS are generally minimal and skin irritation or redness are the most common side effect. Reference Badran, Yu and Adair138 A limitation of taVNS is the vast parameter space. It is still uncertain whether pulse width or frequency are more important. Reference Fallgatter, Neuhauser and Herrmann149
Summary of taVNS
taVNS is a noninvasive tool with few side effects and maybe a promising noninvasive therapy in patients with depression, migraine, and other neurological conditions compared with the invasive and more costly VNS. taVNS can increase or decrease activities in different brain areas. The mechanisms of action is not fully understood and optimal stimulation parameters have not been established. It is important to develop systematic studies which can elucidate the utility of taVNS and understand its mechanism of action.
Other Noninvasive Neuromodulation Techniques
In addition to transcranial magnetic or electric noninvasive neuromodulation techniques, transcranial ultrasound and vestibular stimulation are two NIBS techniques with potential utility in neurophysiology and clinical practice.
Low-intensity TUS is gaining traction as a method of neuromodulation. One study investigated the efficacy of TUS on sensory-evoked brain activity and sensory discrimination abilities. Reference Legon, Sato and Opitz150 TUS targeted to the human primary somatosensory cortex (S1) enhanced performance on sensory discrimination tasks without affecting task attention or response bias. Reference Legon, Sato and Opitz150 In a recent study, 16 subjects were administered low-intensity 500-kHz TUS coupled to a TMS coil and found that TUS suppressed motor cortical excitability with longer TUS duration leading to greater effects. Reference Fomenko, Chen and Nankoo1 Another study used transcranial pulse stimulation (TPS) which involved ultrashort ultrasound pulses instead of periodic waves and long sonication trains to provide better skull penetration due to the dominance of lower frequencies. Reference Beisteiner, Matt and Fan151 Patients with probable Alzheimer’s disease were treated in an open-labeled study with TPS for 2–4 weeks. There were significant improvements found in the language domain along with memory performance in the patients following TPS therapy.
Stochastic vestibular stimulation, or galvanic vestibular stimulation (GVS), is a simple and safe method to induce neuronal activity in both the semicircular canals as well as the otolith organs of the peripheral vestibular system. Reference Fitzpatrick and Day152 GVS has been found to improve stability during balance tasks in healthy individuals by facilitating enhanced information transfer using stochastic resonance principles. A study found that GVS administered to 13 healthy subjects for 60 s while they were walking on a treadmill and simultaneously viewing perceptually matched linear optic flow resulted in improved walking stability. The results suggested that GVS can be used to improve dynamic stability during walking. Reference Mulavara, Kofman and De Dios153 A study using GVS in patients with PD delivered at 70% of cutaneous thresholds (mean current intensity = 0.22 ± 0.02 mA) demonstrated that the sway frequency was mildly reduced following GVS. Reference Tran, Shafiee and Jones154
Comparison between Different NIBS Techniques
Inukai et al. (2016) compared different NIBS techniques (tDCS, tRNS, and tACS) with similar stimulation patterns (1.0 mA and 10 min) in healthy individuals. Cortical excitability was investigated via single-pulse TMS elicited MEPs. The study demonstrated that all three NIBS techniques increased MEP amplitudes compared with baseline but the effects of tRNS were most pronounced. The results suggest that tRNS may be the most effective transcranial electric stimulation (tES) method. Reference Inukai, Saito and Sasaki155
In randomized controlled studies, sham stimulation is needed as a control condition. Sham stimulation is relatively easy with tDCS, tACS, and other electrical stimulation techniques. A typical sham stimulation setting is that the stimulation is applied and then slowly ramped down, and this usually cannot be perceived by the subject. Sham stimulation is more difficult with rTMS due to clicking sound, muscle twitches, and scalp sensations. New designs of sham coils produced some scalp sensation, and some studies used electrical scalp stimulation to mimic scalp sensation to produce a realistic sham condition for rTMS.
There are few studies that directly compared different NIBS modalities. Although, TMS is most established and the only approved modality for treatment of brain disorders, electrical stimulation techniques have the advantage of lower cost, more portable, potentially be applied at home, and can be combined with rehabilitation or training during the application. More research studies comparing different modalities of NIBS and a combination of these modes of stimulation in neurological and psychiatric disorders are needed.
Concluding Remarks
The different neuromodulation techniques of NIBS such as TMS, tDCS, tACS, RNS, VNS, TUS, and GVS not only helped in understanding the brain physiology but can be a useful treatment in some of the neurological and psychiatric disorders. Further research is needed to expand the clinical utility of NIBS and combine various modes of NIBS to optimize the neuromodulation induced clinical benefits.
Acknowledgments
All authors acknowledge the funding support of National Institute of Mental Health and Neuro Sciences (NIMHANS) intramural research funding for part of this work.
Conflicts of Interest
All authors declare no conflicts of interest related to this study.
Statement of Authorship
AB: prepared the initial draft and assisted in revision. KM and SS: assisted in preparation of the initial draft and subsequent drafts. STN, PKP, and RC: reviewed and suggested modification at various stages of preparation of the final draft and revision of the manuscript. KU: conceptualized the review and provided the guidance and overall supervision.