The Scarce Resources for a Nuclear Detonation Project, presented in this issue of Disaster Medicine and Public Health Preparedness, was undertaken to guide community planning for the first 4 days after a nuclear detonation incident. During this period, the demand for resources will vastly outstrip available supplies. The article by Knebel et alReference Knebel, Coleman and Cliffer1 in this issue includes basic descriptions of nuclear detonation scenarios and a response framework. A nuclear detonation differs from other mass casualty incidents in 2 ways: the very high number of affected individuals and the release of ionizing radiation. Thus, plans for responding to a nuclear detonation must consider the many consequences of radiation (environmental, clinical, and psychological) across a wide population.
The present article briefly reviews pertinent aspects of clinical radiation injury gleaned from reports of human exposure and research in animal models. Specifically, we describe the features of acute radiation syndrome (ARS), outline data supporting a synergistic effect on mortality from radiation in combination with either traumatic injury or cutaneous thermal and/or radiation burns, and discuss evidence for clinical benefit from some facets of supportive care, including antibiotics and myeloid cytokines (eg, granulocyte colony-stimulating factor [G-CSF]). To provide context specific to nuclear detonation response, the article includes model-based projections of the number of irradiated casualties, their resource needs, and the anticipated resources available. Subsequent articles in this issueReference Coleman, Weinstock and Casagrande2Reference Hick, Weinstock and Coleman3Reference Murrain-Hill, Coleman and Hick4 address key aspects of radiation injury management within the context of medical response to a mass casualty event. Because the Scarce Resources for a Nuclear Detonation Project focuses on the initial response after the detonation, the long-term effects of radiation (eg, cancer, teratogenesis, and heritable genetic effects) are not discussed.
IRRADIATED CASUALTIES AND RESOURCES AFTER A NUCLEAR DETONATION
Computer modeling in Knebel et alReference Knebel, Coleman and Cliffer1 includes casualty estimates across a range of potential nuclear detonation scenarios. As illustrated in Figure 1, computer modeling of a 10-kT nuclear detonation (identified in the National Planning Scenarios5) suggests that the zone of significant infrastructure damage would extend approximately 2 mi from ground zero, with glass breakage and traffic crashes beyond 2 mi. As such, wider regional infrastructure would be left largely intact and would contribute to the response.Reference Hick, Weinstock and Coleman3

FIGURE 1 Approximate prompt radiation and fallout pattern from a 10-kT nuclear detonation
Prompt radiation extends approximately 1 mile from the epicenter, while the shape of the fallout zone is determined by upper-level winds. (Buddemeier 2009.)
Despite extended regional capacity, the mismatch between casualties and available resources close to the detonation would be striking. Using Washington, DC, as an example, Tables 1 and 2 include general estimates of demand for and availability of selected resources, using modeling similar to the casualty modeling in Knebel and colleagues.Reference Knebel, Coleman and Cliffer1
TABLE 1 Resource Demand and Availability after a Nuclear Detonation in Washington, DC

TABLE 2 Needs and Availability for Blood Products and Skin Grafts After a Nuclear Detonation in Washington, DC

The limited availability of local resources contrasts starkly with the expected overwhelming demand. For example, approximately 500 vacant functioning adult hospital beds per million people population are available at any given time in the United States.Reference Hall7 Based on computer modeling, approximately 1000 beds are vacant within the Washington, DC, metropolitan area, compared with a predicted need for 180 000 beds (Table 1). For pediatric patients, the number of vacant beds is closer to 250/millionReference Hall7 and estimates for critical care beds are even lower. For example, only 62 pediatric intensive care unit beds are available on an average day in all of New York City.Reference Fliedner, D Dörr and Meineke8
Fewer than 100 ambulances serve Washington, DC, proper (Table 1). Thus, the overwhelming majority of people who present for medical care will arrive without assistance from first responders or rescue vehicles. In addition, emergency responder operations will be limited within highly radioactive zones due to radiation and, in some areas, infrastructure damage.5 Most severely injured patients with or without radiation injury would not receive life-sustaining medical care quickly, so many would succumb to their injuries. Thus, the effective demand for many resources such as blood products would be far less than estimates of need based solely on the expected numbers and extents of injuries (Table 2).
Resource scarcity after a nuclear detonation will be both location and time dependent. Figure 2 is an illustration of this concept. Because resource availability (y-axis) declines after detonation, the operative standard of care (defined by the Institute of Medicine9) transitions from conventional to contingency to crisis. The 5 curves represent different medical care settings that vary by distance from the epicenter. A radiation treatment, triage, and transport (commonly known as RTR1) site (outlined in Knebel et alReference Knebel, Coleman and Cliffer1) that forms close to the detonation is likely to have drastic resource scarcities and then disband after evacuation is complete. Resource scarcity at local medical care sites will improve with time after the detonation, but they may not reach normal levels for an extended period. Regional hospitals will differ in their availability of resources based on location relative to the detonation and their role in the community (eg, trauma or pediatric center). Finally, hospitals and referral centers distant from the incident may not be affected for several days, but they may face shortages or be overwhelmed as casualties arrive at their centers or supplies cannot be replenished due to intense demand.

FIGURE 2 Hypothetical representation of resource availability (left y-axis) after a nuclear detonation based on location and type of site
The operative standard of care (right y-axis) follows the definitions outlined by the Institute of Medicine.Reference Hick, Weinstock and Coleman3 Centers close to the site would be immediately impacted and require crisis standards of care. RTR1 (Radiation TReatment, TRiage, and TRansport) sites will be established close to the epicenter shortly after the event and may be disbanded after a few days, as salvageable victims are evacuated. Distance from the detonation will be the primary determinant of timing and severity of resource shortages at regional medical centers (MC). Even referral centers in other regions may experience abrupt resource shortages due to patient transfers or depletion of nationwide supplies.
ARS
The effects of radiation are dependent on the overall dose, dose rate, radiation quality, and fraction of the body that is irradiated. Higher doses (Gray units are used, which equal 100 rad), greater dose rates (Gy/h), and irradiation to larger fractions of the body produce greater injury. The same radiation exposures can cause drastically different signs and symptoms across a population.Reference Friesecke, Beyrer and Fliedner11 Many genetic, demographic, dietary, and other factors can contribute to the manifestations of radiation injury, but most of the interindividual differences are poorly understood. Thus, although radiation exposure is used to guide triage and initial management, the overall management for each person will depend on his or her medical course and the availability of resources.Reference Hick, Weinstock and Coleman3
As outlined by Knebel and colleaguesReference Knebel, Coleman and Cliffer1 and illustrated in Figure 1, 2 groups of casualties would be exposed to radiation after a nuclear detonation. The first group is within 1 to 2 km of a 10-kT blast and would receive a high dose of prompt irradiation released in a circumferential pattern at an extremely high dose rate. Because of their proximity to the explosion, many of the casualties exposed to prompt irradiation would also sustain traumatic and/or burn injuries.Reference Knebel, Coleman and Cliffer1Reference Coleman, Hrdina and Bader12
The second group of irradiated casualties would be exposed in the fallout zone downwind of the detonation, where radioactive fallout from the mushroom cloud would contaminate the environment.5 The dose rate of fallout radiation is lower than that for prompt radiation and decreases rapidly with time.Reference Coleman, Hrdina and Bader12 The clinical consequences of fallout radiation at a given dose may be significantly fewer than for the same dose of prompt irradiation because of the lower dose rate from radioactive fallout. In addition, many of the individuals exposed within the fallout zone would have radiation injury only and lack trauma or burns.
Mild symptoms may develop after whole-body radiation exposures as low as 1 Gy. Casualties exposed to >2 Gy are at risk for developing clinically significant ARS. ARS represents a constellation of signs and symptoms that occur between several minutes and several weeks after exposure.Reference Waselenko, MacVittie and Blakely10Reference Friesecke, Beyrer and Fliedner11Reference Dainiak, Waselenko and Armitage13 ARS primarily involves the 4 organ systems with the greatest acute sensitivity to ionizing radiation: hematologic, gastrointestinal, cutaneous, and cardiovascular/central nervous systems. Figure 3 illustrates the nature and time course of symptoms, which can vary based on radiation dose. The signs and symptoms of radiation exposure mimic those observed after therapeutic whole-body irradiation or treatment with clastogenic chemotherapy and can range from mild nausea at low doses to rapid neurovascular collapse at high doses (ie, >10-20 Gy). While specific organ syndromes are discussed, multiorgan effects may mediate many clinical signs and symptoms.Reference Fliedner, Chao and Bader14

FIGURE 3 Simplified time courses for hematologic, gastrointestinal (GI) and central nervous system (CNS) symptoms at different whole-body dose exposures
The relative severity of signs and symptoms is on an arbitrary scale. (Modified from WaselenkoReference Waselenko, MacVittie and Blakely10).
ARS classically progresses through 4 clinical phases: prodrome, latency, manifest illness, and either recovery or death. The prodromal period is characterized by nausea, vomiting, fatigue, and, at higher doses, autonomic instability and even loss of consciousness.Reference Cerveny, MacVittie and Young15 The latency period is characterized by partial or complete resolution of symptoms. The extent and duration of latency are inversely proportional to dose. Casualties who receive doses >6 Gy may have little or no latency because signs and symptoms of end-organ injury can develop within hours to days after exposure.
Peripheral blood cytopenias can develop at doses as low as 1 Gy (Figure 3) due to the heightened apoptotic response of both differentiated and progenitor hematopoietic cells.Reference Milyavsky, Gan and Trottier16 The rapidity of onset and severity of peripheral blood cytopenias are proportional to dose. Lymphocytes are particularly sensitive to irradiation and the kinetics of lymphocyte depletion is a useful parameter for rapidly assessing the extent of radiation exposure.Reference Hick, Weinstock and Coleman3 Importantly, patients experiencing burns or trauma may develop lymphopenia in the absence of radiation exposure.Reference Cheadle, Pemberton, Robinson, Livingston, Rodriguez and Polk17Reference Maldonado, Venturoli, Franco and Nunez-Roldan18
White blood cell counts may also follow a predictable time course depending on the extent of exposure (Figure 4). Evidence from radiation accident casualties indicates that a transient increase in granulocyte count (an “abortive rise”) preceding a slow decline for 10 to 15 days may indicate reversible hematologic ARS, and thus a higher likelihood of survival (Figure 4). In contrast, irreversible hematologic ARS (ie, myeloablation) follows a different pattern of granulocyte kinetics, with a rapid fall between 4 and 6 days after exposure.Reference Fliedner, Graessle, Meineke and Dörr19 In a review of 60 radiation accident casualties with granulocyte kinetics that were consistent with reversible hematologic ARS, all 60 ultimately achieved autologous hematopoietic recovery, although in some cases not until 60 to 80 days after exposure.Reference Fliedner, Graessle, Meineke and Dörr19

FIGURE 4 Leukocyte counts based on exposure dose in patients exposed to radiation in Chernobyl
Note that doses less than 5 Gy are associated with an early abortive rise (transient increase) in leukocytes, which are primarily composed of granulocytes. The onset of neutropenia may not occur for weeks, especially with lower exposures, and the duration of neutropenia may be prolonged. (Modified from Vorobiev 1997.)
Many radiation accident casualties received heterogeneous doses from partial-body shielding. Similar heterogeneity may be common in casualties exposed to prompt irradiation from a nuclear detonation because the radiation may be blocked by buildings and other structures. If small areas of bone marrow are relatively spared by partial shielding, then hematologic reconstitution may occur even if the vast majority of the marrow receives a dose that is adequate to confer irreversible injury. Dose heterogeneity may be less prevalent among casualties exposed to fallout because fallout would broadly contaminate the environment.
Although whole-body doses as low as 2 Gy can cause clinically significant ARS, both the timing of death and the organ system most likely to mediate death differ between lower and higher dose exposures. Death from hematologic ARS can occur with exposures between 2 and 6 Gy as a result of infection and/or bleeding from neutropenia and thrombocytopenia, respectively. The long circulating life span of red blood cells makes anemia less of an issue in the early period after radiation exposure. Death from hematologic ARS typically occurs between 2 and 8 weeks after exposure.Reference Waselenko, MacVittie and Blakely10 At doses >5 to 6 Gy, gastrointestinal ARS may cause death through extensive diarrhea and transmigration of enteric organisms into the bloodstream, typically within 1 to 2 weeks of exposure.Reference Madonna, Ledney, Moore, Elliott and Brook20 Symptoms of gastrointestinal ARS include nausea, vomiting, and diarrhea; however, nausea and vomiting are nonspecific symptoms that can occur at lower doses or even in the absence of radiation.Reference Demidenko, Williams and Swartz21 At doses >8 to 10 Gy, death resulting from cardiovascular/central nervous system ARS may occur within days after exposure; symptoms include headache, confusion, loss of consciousness, seizures, and autonomic instability.Reference Waselenko, MacVittie and Blakely10
The LD50/60 (dose at which only 50% of humans will survive at least 60 days) for adults in the absence of supportive care is approximately 3.5 Gy.Reference Waselenko, MacVittie and Blakely10Reference Anno, Young, Bloom and Mercier22 Death at this dose would primarily result from hematologic ARS and would occur 2 to 6 weeks after exposure.Reference Waselenko, MacVittie and Blakely10Reference Anno, Young, Bloom and Mercier22 Casualties who survive long enough for hematologic reconstitution are likely to fully recover. In contrast, much higher doses lead to earlier death and almost no likelihood of full recovery. A Nuclear Regulatory Commission studyReference Haskin, Harper and Goossens23Reference Haskin, Harper and Goossens24 estimated that the LD50/7–14 for humans is 9.6 Gy (95% confidence interval, 5.7-17.2 Gy). Death from this higher dose occurs within 7 to 14 days because of serious gastrointestinal tract and possibly cerebrovascular injury, in addition to potentially irreversible hematologic ARS. As outlined below, interventions for hematologic ARS, including fluid support, antimicrobials, and transfusions, may increase the LD50/60 to 600 to 700 cGy,Reference Hall7 but it remains unclear whether any available intervention can reduce early death after higher doses.
ARS in organ systems other than the hematologic, cutaneous, gastrointestinal, and neurovascular may be underappreciated, especially in casualties exposed to higher doses. In a review of radiation accident casualties, 32 of 45 cases with severe ARS had respiratory involvement, 20 had cardiovascular involvement (primarily manifested as heart failure), 25 had liver involvement, and 32 had urogenital involvement.Reference Fliedner, D Dörr and Meineke8 Delayed effects of acute radiation exposure, which can involve almost any organ system, can develop months to years after exposure.
SOURCES OF DATA ON RADIATION INJURY
Despite more than 6 decades of research, major gaps exist in our understanding of how genetic, demographic, geographic, and other factors would affect radiation injuries after a nuclear detonation in a modern US city. The available literature on radiation injury derives from 4 sources of observational and experimental research, and was largely collected in the 1950s and 1960s. None of the 4 sources fully captures the spectrum of injuries expected after a ground-level nuclear detonation within a modern US city, but each provides some insights.
Nuclear Bomb Detonations
Cohorts of nuclear bomb casualties from the Hiroshima and Nagasaki detonations have been followed for more than 6 decades. Up to 70% of casualties postdetonation had combined injury (ie, radiation plus trauma and/or burns)Reference Iijima25Reference Kishi2627; however, the detonations at Hiroshima and Nagasaki were both air bursts. Air bursts produce higher rates of combined radiation and burn injury than would be expected after a ground-level detonation, which is the type of event specified in National Planning Scenario 1.5 In addition, both Japanese cities contained primarily wooden structures, so building collapse and secondary fires were more common than would be anticipated in a modern US city.5 As a result, lower rates of combined injury are expected in current scenarios, especially within the fallout zone.Reference Knebel, Coleman and Cliffer1 Most important, little medical care was available in the aftermath of the Hiroshima and Nagasaki detonations, making it difficult to estimate the potential benefits of supportive care interventions.
Medical and Industrial Radiation Accidents
Between 1944 and 2003, there were 426 major radiation accidents worldwide involving more than 130 000 people.Reference Dainiak and Ricks28 Nearly 90% of the exposures resulted from the Chernobyl accident in 1986. Most of the other accidents led to only a few casualties. As a result, extensive resources and supportive care were available to treat those injured by these smaller-scale accidents. Databases on radiation accident casualties, including 1 at the Radiation Emergency Assistance Center/Training Site)29 have been established but are not open access. The SEARCH database, established by the University of Ulm, contains details from more than 800 whole-body radiation exposures from 70 accidents.Reference Fliedner, Graessle, Meineke and Dörr19 Data from SEARCH was used by the European Medical Treatment Protocols effort to establish triage and treatment approaches for radiation accident casualties,Reference Friesecke, Beyrer and Fliedner11 as outlined for granulocyte kinetics above.
There are important shortcomings in the literature of radiation accidents that limit its applicability to nuclear detonation response planning. First, there were no standardized approaches applied to the casualties. Treatment was essentially ad hoc, and each center used different combinations of cytokines, antibiotics, and even cellular therapies. Thus, the interpretation of outcomes and even the relations between dose and clinical parameters, are highly confounded. Second, the nature of radiation injury is likely to differ between radiation accident and nuclear detonation casualties. For example, the fraction of radiation from neutrons was significantly higher in industrial accidents like the Tokaimura accident than would be expected after a nuclear detonation.Reference Hirama, Tanosaki and Kandatsu30 Finally, industrial radiation accidents affect primarily relatively young healthy men, so the effects of a particular exposure across a range of demographic groups cannot be determined.
Therapeutic Radiation
The third source of information on radiation injury (and the benefit of targeted radiation countermeasures) is from patients who have been treated with therapeutic radiation. Extrapolation from the therapeutic radiation experience to nuclear detonation scenarios is also problematic. Patients treated with therapeutic radiation commonly have underlying illnesses (primarily cancer) that affect their overall health, response to radiation, and recovery. These patients are frequently receiving other agents, such as chemotherapy, that further complicate interpretation of the effects from radiation. In addition, most therapeutic radiation is highly focused on a limited portion of the body and given in multiple fractions to minimize toxicity. Even in the setting of total body irradiation for hematopoietic stem cell transplantation (HSCT), partial shielding of radiosensitive organs, such as the lungs, is common. Finally, therapeutic radiation is provided at expert centers as part of a comprehensive approach designed to maximize efficacy while minimizing toxicity. Thus, many patients treated with therapeutic radiation will also receive supportive care, myeloid cytokines (eg, G-CSF), and even HSCT.
Animal Models
The use of human data to plan for nuclear detonation response avoids the need to extrapolate from results in animal models; however, animal models are essential for prospective research, including the evaluation of potential radiation countermeasures.Reference DiCarlo, Hatchett and Kaminski31Reference Prasanna, Blakely and Bertho32Reference Williams, Brown and Georges33 As outlined in Table 3, the sensitivity of different mammalian models to whole-body irradiation varies considerably, with LD50/30 ranging between 240 and 1000 cGy. The variability in effect that radiation exposure has on individuals, the potential for combined injuries to affect radiation outcomes, and interspecies variability make it extremely difficult for a single animal model to capture the effects of radiation exposure and predict medical countermeasure efficacy with adequate fidelity. Thus, a variety of animal species have been used. It is important to note that depending on the radiation syndrome being analyzed, different models and/or strains may be appropriate. For example, swine are widely considered to be the most appropriate human surrogates for studies involving radiation injury to skin.Reference Barbero and Frasch34Reference Hopewell35Reference Simon and Maibach36
TABLE 3 Comparison of LD50/30 Across Multiple Mammalian Species Compiled From the Published Literature
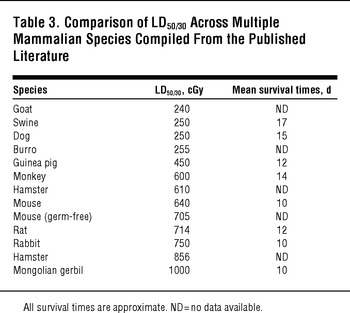
Rodent models, particularly mice, have been used extensively for mechanistic and proof-of-concept studies and to evaluate new and existing radiation medical countermeasures (MCMs). Rodent models are relatively inexpensive, readily available in a wide selection of genetic backgrounds, and can be studied humanely using survival as an endpoint. A primary shortcoming of all of the rodent models is the challenge of providing supportive care similar to what may be provided to a human patient.
As noted in Table 3, the average LD50/30 for mice exposed to whole-body gamma irradiation is 640 cGy, but it ranges between 540 and 850 cGy depending on the strain (Figure 4). Other strain-specific differences have been noted in radiation response. For example, a recent report investigating the development of radiation-induced, late lung complications in different strains of mice found that certain strains were more representative of human responses than othersReference Jackson, Vujaskovic and Down38; for example, C57BL/6 mice exhibited pleural effusions at 6 to 9 months, which are not normally observed in irradiated humans. Rat models are useful for determining the pharmacokinetics and pharmacodynamics of candidate MCMs, but there is less literature available to support their use as an efficacy model for radiation syndromes. Nonetheless, rats have a long track record as the preferred animal models for studies of radionuclide decorporation.Reference Dukhuongl39
Canines (especially beagles) have been studied extensively as animal models of hematologic ARSReference Handford, Stonestreet and Johnson40 and for radionuclide decorporation.Reference Melo, Lundgren, Muggenburg and Guilmette41 Canines have radiation responses similar to humans and respond well to supportive care and antibiotics.Reference Cerveny, MacVittie and Young15Reference MacVittie, Farese and Jackson42 Other animal models for testing radiation effects and potential MCMs have been described in the published literature, including ovine, avian, bovine, equine, and a wide array of nonhuman primate species, especially the rhesus macaque. The latter now represents the gold standard for most acute radiation and MCM studies because of its close evolutionary relation to humans and the similar effects of supportive care (Table 4).Reference DiCarlo, Hatchett and Kaminski31
TABLE 4 LD50 Values (Gy) for Humans and Rhesus Monkeys Exposed to Different Radiation Types, With Different Levels of Supportive Care

ADDITIONAL Factors Affecting Outcome
Studies in animal models have established several radiation- and organism-specific determinants of radiation response. Presumably, these same determinants affect humans exposed to radiation. The factors include the range of doses selected, radiation quality, dose rate, and the fraction of the body exposed (eg, partial vs total body). Even the time of day of irradiation can affect outcome.Reference Haus57 In animal models, sex and age can alter not only the outcome after radiation exposure but also pharmacologic parameters important for an MCM, such as clearance, metabolism, and safety profile. Female rodents, in general, have decreased radiation LD50/30 values as compared with their male counterparts.Reference Kallman58Reference Thomson, Tourtellotte, Carttar, Cox and Wilson59 The age of rodentsReference Casarett60Reference Grahn61 and caninesReference Garner, Phemister, Angleton, Lee and Thomassen62 at the time of irradiation significantly influences their radiation sensitivity, with LD50/30 values for mice ranging nearly 4-fold, from 210 to 740 cGy, depending on age at the time of irradiation. As expected, the health status of the animals within a colony can affect radiation response. LD50/30 values are substantially lower for mice exposed to conventional environmental pathogens than for animals maintained in “pathogen-free” environments.Reference McLaughlin, Dacquisto, Jacobus and Horowitz63 Even the type of food and water administered to animals can affect their radiation response.Reference Hall, White and Lang64
Radiation Combined Injury
Based on multiple animal models and observations in humans, the combination of radiation with either trauma or burns markedly increases mortality compared with the same dose of radiation alone. Outcomes among casualties of the Chernobyl accident with combined injury were universally poor.Reference Baranov, Guskova, Nadejina and Nugis VYu65 In a study by Ledney,Reference Ledney, Elliott and Moore66 the LD50/30 in mice for radiation alone was approximately 963 cGy but dropped to 820 cGy when combined with an otherwise nonlethal 15% body surface area burn.Reference Vorobiev67 LD50/30 dropped from 963 cGy to 761 cGy when radiation was combined with an otherwise nonlethal puncture wound. A similar study of radiation with or without nonlethal wound is outlined in Figure 5.

FIGURE 5 C3H/H3N female mice were exposed to radiation alone or radiation in addition to a non-lethal wound (combined injury)
The combination increased mortality at all doses, but mortality was reduced by applying topical gentamicin or silvadene. (Modified from Ledney.Reference Garner, Phemister, Angleton, Lee and Thomassen62)
In canines, a 20% body surface area burn would result in minimal mortality, but mortality increases to 73% if the same burn is combined with only 1 Gy of whole-body irradiation.Reference Brooks, Evans, Ham and Reid68 In rats, lethality from a burn increased from 50% when the burn was administered alone to 65% when combined with 1 Gy and 100% when combined with 2.5 Gy.Reference Alpen and Sheline69 Similar findings were reported when burn was combined with radiation in pigsReference Baxter, Drummond, Stephens-Newsham and Randall70 or guinea pigs.Reference Korlof71
The additional mortality from combined injury likely results, at least in part, from infection by enteric organisms. Sublethal wound or burn injury increases the translocation of gut bacteria into the bloodstream induced by sublethal irradiation.Reference Mishima, Yukioka, Matsuda and Shimazaki72Reference Yan, Ran and Wei73 Whitnall et al74 reported that sublethal doses of both radiation and Klebsiella pneumoniae administered to mice produce 100% mortality because of sepsis. In fact, even doses of radiation as low as 0.5 Gy increase the mortality from bacterial infection in this model.Reference Whitnall, Elliott and Harding74 Wound closure is also delayed by radiation in a dose-dependent manner,Reference Ran, Yan, Cheng, Lin, Wei and Zheng75 increasing the potential for infection from a cutaneous source after either burn or traumatic injury.
Supportive Care
Current data suggest that supportive care can be an extremely effective radiation countermeasure.Reference MacVittie, Farese and Jackson42 Supportive care in animal studies may be generally classified as “normal support,” defined as administration of fluids, blood products, antibiotics, and in some cases parenteral nutrition, or “heroic support,” defined as comprehensive, individualized care up to and including cytokines and HSCT. In addition, the management of combined injuries, both surgical and nonsurgical, is an essential component of supportive care.
A series of studies has demonstrated the efficacy of antibiotic support and platelet transfusions in dogs exposed to radiation,Reference Furth, Coulter, Miller, Howland and Swisher76Reference Jackson, Sorensen, Cronkite, Bond and Fliedner77Reference Perman, Cronkite, Bond and Sorensen78 either in randomized trials or when compared with historical controls followed without supportive care. Antibiotics also improve survival in mice after combined injury.Reference Ledney and Elliott37 Even topical antibiotics can improve survival after combined radiation and wound injury. Figure 5 demonstrates the efficacy of topical silvadene or gentamicin applied to wounded mice also exposed to varying doses of radiation.Reference Ledney and Elliott37 This suggests a direct effect on survival from infection by cutaneous organisms, even at low doses, and the potential for simple interventions to improve outcome.
Supportive care in irradiated humans has been estimated to increase LD50/60 values from approximately 3.5 to 4 Gy up to approximately 6 to 7 GyReference Dainiak, Waselenko and Armitage13 (Table 4). The LD50/60 after the Chernobyl incident was 8.88 Gy, although many casualties received relatively low dose ratesReference Anno, Young, Bloom and Mercier22 and some underwent HSCT.Reference Baranov, Gale and Guskova80 Recommended approaches for antibiotic and myeloid cytokine use (for both prophylaxis and treatment) after radiation exposure largely follow algorithms for the treatment of chemotherapy-associated neutropenia.Reference Dainiak, Waselenko and Armitage13Reference Fliedner, Chao and Bader14Reference Gourmelon, Benderitter, Bertho, Huet, Gorin and De Revel81 Of note, the management of patients with severe ARS, combined injuries, or both is highly labor and resource intensive; a single casualty of the Tokaimura radiation accident required abundant personnel and resources for comprehensive and intensive care, including approximately 10 L of fluid daily and extensive transfusion support.Reference Ishii, Futami and Nishida82 Presumably, resource scarcities after a nuclear detonation would greatly reduce the number of casualties who could receive this level of support.
Cytokine Therapy
Myeloid cytokines enhance neutrophil recovery in patients with neutropenia. Three myeloid cytokines are approved by the Food and Drug Administration for the management of chemotherapy-associated neutropenia: granulocyte-colony stimulating factor (G-CSF), granulocyte macrophage-colony-stimulating factor (GM-CSF), and pegylated G-CSF. Note that these agents are not approved by the Food and Drug Administration for use after a nuclear detonation but would likely be used either under an emergency use authorization or off-label, as discussed by Murrain-Hill and colleagues.Reference Murrain-Hill, Coleman and Hick4
In a meta-analysis of trials that used G-CSF for patients treated with chemotherapy for solid tumors or lymphoma,Reference Kuderer, Dale, Crawford and Lyman83 G-CSF reduced infection-related mortality by 45% from an absolute frequency of 2.8% to 1.5%. Although this absolute benefit is small, in a scarce resources setting, the prevention or shortening of febrile neutropenia would offer the added benefit of reducing the need for additional medical care, including inpatient hospitalization. In the meta-analysis, G-CSF reduced the frequency of febrile neutropenia from 39.5% to 22.4%,Reference Kuderer, Dale, Crawford and Lyman83 suggesting a significant potential for benefit.
Studies in irradiated primates have consistently demonstrated more rapid neutrophil recovery after irradiation in animals treated with myeloid cytokines.Reference Waselenko, MacVittie and Blakely10 In rhesus macaques, G-CSF or GM-CSF increased overall survival after irradiation when given for 14 to 21 days.Reference Farese, Hunt, Grab and MacVittie84Reference Neelis, Dubbelman, Qingliang, Thomas, Eaton and Wagemaker85Reference Neelis, Hartong, Egeland, Thomas, Eaton and Wagemaker86 Some reports have suggested that the survival benefit is maximized if myeloid cytokines are initiated within 24 hours after exposure. Studies to verify this possibility are planned or in progress.
G-CSF and GM-CSF have been used in a subset of radiation accident casualties. In a series of 28 cases compiled by the Radiation Emergency Assistance Center/Training Site, neutrophil recovery appeared to have been hastened in 25; however, many of these casualties received both agents and some also received interleukin-3.Reference Waselenko, MacVittie and Blakely10 Myeloid cytokines may be beneficial even several weeks after exposure. Recently, 2 radiation accident casualties were treated with cytokines beginning as late as 28 days after exposure and both had rapid responses with complete correction of neutropenia.Reference Gourmelon, Benderitter, Bertho, Huet, Gorin and De Revel81
Few states have plans for response to a nuclear detonation,Reference Dainiak and Ricks28 but even among states that do, stockpile targets for individual hospitals are in the range of 150 doses of myeloid growth factors and antibiotics,29 compared with the hundreds of thousands of casualties expected to sustain radiation injury (Table 1). Thus, it is essential to use thoughtful, effective triage protocols for selecting which casualties should receive myeloid cytokines from local caches or from rapid deployments from the Strategic National Stockpile. Triage guidelines along with recommendations for myeloid cytokine use are included in the article by Coleman et alReference Coleman, Weinstock and Casagrande2 in this issue.
Ideally, casualties who qualify for myeloid cytokines should initiate a treatment course within approximately 24 hours after exposure. Because early administration of the first dose appears to be important, it is essential to develop approaches for rapidly offering these agents to as many significantly irradiated casualties as possible. A user-managed inventory concept is being developed (C. N. Coleman, personal communication, 2010) that would make additional drugs available locally at a number of medical facilities by virtue of maintaining a bubble in the supply line of dual-use drugs, such as cytokines used in cancer therapy. This would result in more first doses available early after the detonation so that treatment of irradiated casualties could be initiated locally and then be referred to less resource-scarce settings to receive the remaining doses. In so doing, a 10-day course for 1 person locally becomes a 1-day course for 10 people, who can be evacuated and then receive subsequent doses in a more resource-rich setting.
The initial dose-finding studies of G-CSF demonstrated a clear linear dose-response effect.Reference Brooks, Evans, Ham and Reid68Reference Alpen and Sheline69Reference Baxter, Drummond, Stephens-Newsham and Randall70 Thus, approaches to extend the number of recipients by reducing the dose, extending the interval between doses, or abbreviating the course of therapy are likely to compromise efficacy and should be avoided. Children with ARS will also benefit from myeloid cytokines, so stockpiles should include formulations with dosing flexibility.
HSCT
Some casualties of a nuclear detonation will receive sufficient doses of radiation to cause irreversible bone marrow damage. Allogeneic HSCT in this setting has the potential to restore hematopoietic function. It remains unclear, however, whether HSCT has the potential to improve survival in radiation casualties, especially those with traumatic and/or burn injuries. HSCT has demonstrated a survival benefit in animal modelsReference Ledney and Elliott37; however, animals in these studies typically received syngeneic (or highly genetically similar) stem cell transplants, which eliminates or markedly reduces the likelihood of graft-vs-host disease.
To date, 31 patients have undergone allogeneic HSCT after accidental whole-body radiation exposure. The preparative regimens given, if any, varied widely, as did antibiotics and graft-vs-host disease prophylaxis.Reference Dainiak and Ricks28Reference Weisdorf, Chao and Waselenko87 The median survival after transplantation among these patients was approximately 1 month. Only 4 patients survived 1 year after HSCT; all 4 rejected the transplant and reconstituted autologous hematopoiesis, suggesting that the transplant offered little benefit. Among the 31 HSCT recipients, graft-vs-host disease contributed to mortality in more than 20%. It is unknown whether any of the casualties who succumbed to transplant-related complications would have reconstituted autologous hematopoiesis if the transplant had been withheld. Thus, there is no evidence that HSCT improves survival in humans exposed to nontherapeutic radiation.
GUIDANCE AND SURGE CAPACITY FOR IRRADIATED CASUALTIES
The detonation of a nuclear device within a US city would create a national need for health care practitioners to manage casualties exposed to radiation; however, only a small fraction of practitioners have either training or experience in the field of radiation injury.
Radiation Emergency Medical Management Web Site
To establish a resource for pre- and postevent information, training and communication, the Department of Health and Human Services (DHHS) launched the Radiation Emergency Medical Management (REMM) Web site in March 2007.Reference Bader, Nemhauser and Chang88 Recognizing the limitations of available human and animal data as an evidence base for guiding medical management, REMM has the following goals:
• Provide guidance for health care providers, primarily physicians, about clinical diagnosis and treatment of radiation injury during radiological and nuclear emergencies
• Provide just-in-time, evidence-based, usable information with sufficient background and context to make complex issues understandable to those without formal radiation medicine expertise
• Provide Web-based information that is also downloadable in advance so that it would be available during an event if the Internet is not accessible
Since its establishment, REMM content has expanded significantly and REMM versions have been launched for various mobile platforms.89 The primary managers of the site are content and technology experts at various DHHS agencies, including the National Cancer Institute, the National Library of Medicine, the Centers for Disease Control and Prevention, and the Office of the Assistant Secretary for Preparedness and Response, which has its own public health emergency Web site (http://www.phe.gov/preparedness/Pages/default.aspx).
Guidelines for the diagnosis and management of radiation injury are included on the REMM site and extensively referenced from the peer-reviewed literature. Template admission orders for irradiated casualties are also available (http://www.remm.nlm.gov/adultorderform.htm) and include antibacterial, antifungal, and antiviral prophylaxis; decorporation agents; myeloid cytokines; and other elements of radiation casualty management. Content from the Scarce Resources for a Nuclear Detonation Project outlined in this special issue of Disaster Medicine and Public Health Preparedness is being incorporated into the site.
Radiation Injury Treatment Network
Many of the casualties with radiation-only injury will be salvageable but require outpatient and/or inpatient care. Recognizing this, the US National Marrow Donor Program, the US Navy, and the American Society for Blood and Marrow Transplantation collaboratively developed the Radiation Injury Treatment Network (RITN), which comprises 55 HSCT centers, stem cell donor centers, and umbilical cord blood banks across the United States.Reference Davids, Case and Hornung90Reference Weinstock, Case and Bader91 The goals of RITN are to develop treatment guidelines for managing hematologic toxicity among victims of radiation exposure, to educate health care professionals about pertinent aspects of radiation-exposure management, to assist in coordinating the medical-situation response after a radiation event, and to provide comprehensive evaluation and treatment for victims at participating centers.
In recent tabletop exercises,Reference Davids, Case and Hornung90 RITN centers volunteered to provide surge capacity for <2000 total irradiated people at their institutions. Strategies to increase bed availability and extend staff and resources could increase surge capacity 10-fold but would require changes in operating standards.Reference Davids, Case and Hornung90 Considering the large number of irradiated casualties anticipated after a nuclear detonation (Figure 1), many centers across the country in addition to those in RITN will be asked to participate in the management of casualties with ARS.
CONCLUSIONS
An extensive literature base has described the effects of radiation injury in humans and animal models. Many gaps in our understanding still exist and some injuries cannot be modeled in the laboratory, but several important findings have emerged. First, there is marked variability in radiation response within and across species. Second, many factors related to either the radiation or the recipient can affect the outcome of radiation injury. Third, traumatic and burn injuries can synergistically increase the mortality from radiation. Fourth, supportive care measures such as antibiotics, fluids, transfusion support, and possibly myeloid cytokines can reduce the mortality of radiation injury. These findings establish a foundation for the triage and treatment approaches outlined in this special issue. The need is critical for just-in-time guidance and surge capacity for irradiated casualty management. The REMM Web site89 was established to provide available evidence and expert opinion, including pre- and postevent training for health care workers and planners. RITN is a model for voluntary planning at centers across the country.
Author Disclosures: Dr Weinstock is a paid consultant by Genzyme and Novartis.
Acknowledgments: The authors acknowledge the members and contributors of the National Institutes of Health's 2004 Animal Endpoint Working Group and the 2006 Ad-hoc Radiological and Nuclear Subcommittee Product Development Tools Working Group of the Weapons of Mass Destruction Steering and Integration Group. The authors also thank Alicia Livinski, biomedical librarian, National Institutes of Health Library, and Paula Murrain-Hill, program analyst, Office of the Assistant Secretary for Preparedness and Response, DHHS, for their assistance with the preparation of this article.