Introduction
During the last century, abiotic stresses such as drought, salinity and extreme temperatures have become widespread as a consequence of climate change-related phenomena, mainly those prompted by increasingly intensive anthropogenic activities. Among the abiotic stresses, drought represents one of the major constraints on agricultural productivity and food security (Boyer et al. Reference Boyer, Byrne, Cassman, Cooper, Delmer, Greene, Gruis, Habben, Hausmann, Kenny, Lafitte, Paszkiewicz, Porter, Schlegel, Schussler, Setterh, Shanahan, Sharp, Vyn, Warner and Gaffney2013), linked strongly with ongoing climate changes (Sheffield et al. Reference Sheffield, Wood and Roderick2012). During drought events, water losses are greater than the water inputs in a particular agroecosystem (Gilbert & Medina Reference Gilbert and Medina2016). In semi-arid regions, drought or water deficit in the soil is common, while crop plants in temperate regions may undergo seasonal periods of water stress (Dubey & Pessarakli Reference Dubey, Pessarakli and Pessarakli2001). Simultaneously, agriculture is one of the top water-consuming sectors worldwide (0.69 of water withdrawal) and agricultural intensification is expected to increase the demand for water worldwide. According to Gilbert & Medina (Reference Gilbert and Medina2016), drought may be defined as a decrease in water inputs in an agro/ecosystem over time that is sufficient to result in soil water deficit, i.e. a decrease in available soil water. How drought affects plant growth and development is dependent on the plant's characteristics and the environment (Gilbert & Medina Reference Gilbert and Medina2016). Drought-induced damage, as well as plant responses to water stress, depend on the severity and duration of the water-shortage period and the plant growth stage and may be recoverable or not (da Silva et al. Reference Da Silva, Nogueira, Da Silva, de Albuquerque, Anjum and Lopez-Lauri2011; Gilbert & Medina Reference Gilbert and Medina2016). Moreover, plants that can acquire more water and those with improved water-use efficiency are more tolerant to drought. During the last decade, many studies have been performed to address in planta effects of this abiotic stress in order to deepen the complex network of responses and plan preventative strategies, focusing increasingly on sustainable approaches (Osakabe et al. Reference Osakabe, Osakabe, Shinozaki and Tran2014; Daryanto et al. Reference Daryanto, Wang and Jacinthe2017). Water deficit impacts plant growth and production adversely, affecting several morphological, biochemical and molecular traits that respond actively to limit drought-induced damage and permit recovery after water restoration (Farooq et al. Reference Farooq, Hussain, Wahid, Siddique and Aroca2012). In particular, abiotic stresses such as water deficit lead to dehydration responses, thus lowering water availability for crucial cellular functions and maintenance of turgor pressure. This leads to a series of damaging responses, such as the disruption of cellular ionic and osmotic homeostasis and the production of reactive oxygen and nitrogen species (RONS) in various organelles with high electron transport rates, causing irreversible cellular damage (Gill & Tuteja Reference Gill and Tuteja2010). Stomatal regulation is one of the key mechanisms that allow water saving, permitting plants to modulate and optimize carbon dioxide (CO2) assimilation v. evaporative water loss (Tombesi et al. Reference Tombesi, Nardini, Frioni, Soccolini, Zadra, Farinelli, Poni and Palliotti2015). Undersoil water limitation, plants promote biosynthesis/accumulation of abscisic acid (ABA), which has a key role in stomatal closure, leading to a decrease in stomatal conductance and minimizing transpiration losses (Yamaguchi-Shinozaki & Shinozaki Reference Yamaguchi-Shinozaki and Shinozaki2006; Nakashima & Yamaguchi-Shinozaki Reference Nakashima and Yamaguchi-Shinozaki2013; Nakashima et al. Reference Nakashima, Yamaguchi-Shinozaki and Shinozaki2014; McAdam et al. Reference McAdam, Brodribb and Ross2016; Vishwakarma et al. Reference Vishwakarma, Upadhyay, Kumar, Yadav, Singh, Mishra, Kumar, Verma, Upadhyay, Pandey and Sharma2017). However, stomatal conductance regulation driven by passive hydraulic-mediated mechanisms has been also reported, e.g. in grapevine (Tombesi et al. Reference Tombesi, Nardini, Frioni, Soccolini, Zadra, Farinelli, Poni and Palliotti2015). Improved knowledge on plant responses to water deficit, which include morphological and physiological adaptations, is crucial to improve drought tolerance in major crops and to develop novel water management and conservation strategies in agriculture. Efficient water management in agriculture will help to reduce crop yield losses and increase water use efficiency, and to cultivate areas currently unsuitable for cultivation due to low precipitation and/or salt accumulation. Different methodologies have been employed to enhance drought/salinity stress tolerance in plants and therefore to increase crop water use efficiency; some are particularly time-consuming (e.g. conventional breeding) and others are currently unacceptable in many countries around the world (e.g. plant genetic modification) (Cattivelli et al. Reference Cattivelli, Rizza, Badeck, Mazzucotelli, Mastrangelo, Francia, Marè, Tondelli and Stanca2008; Hu & Xiong Reference Hu and Xiong2014). As an alternative strategy, the use of root-associated microorganisms to increase crop tolerance and resilience to drought has been explored in recent years (Rolli et al. Reference Rolli, Marasco, Vigani, Ettoumi, Mapelli, Deangelis, Gandolfi, Casati, Previtali, Gerbino, Pierotti Cei, Borin, Sorlini, Zocchi and Daffonchio2015; Chitarra et al. Reference Chitarra, Pagliarani, Maserti, Lumini, Siciliano, Cascone, Schubert, Gambino, Balestrini and Guerrieri2016). Schmidt & Gaudin (Reference Schmidt and Gaudin2017) proposed that an ideotype root system that integrates several traits to improve water and nutrient use efficiency, ameliorating the soil organic matter metabolism and the exudate production, would affect rhizosphere hydraulic conductivity and water uptake positively. Root-associated microorganisms, such as arbuscular mycorrhizal (AM) fungi, might also confer those benefits under irrigated conditions. Arbuscular mycorrhizal fungi colonize plant roots and help their host plants to reach water and nutrients, while in turn receiving carbon compounds, and are considered to be essential elements for plant nutrition as their hyphae can extend for many metres in the ground, helping plants to acquire mineral nutrients present in the soil (Bucher et al. Reference Bucher, Hause, Krajinski and Küster2014). On the other hand, chemical priming is a rapidly emerging field in plant stress physiology and crop stress management (Savvides et al. Reference Savvides, Ali, Tester and Fotopoulos2016). Plants treated with certain natural or synthetic compounds (i.e. chemical agents) prior to stress events show enhanced tolerance when exposed to sub-optimal abiotic conditions (e.g. drought, heat, salinity, heavy metals). Stress impacts on plant growth and yield in primed plants are reduced remarkably in comparison with non-primed plants. In the current review, some aspects related to the application of both strategies (biological and chemical priming) will be reported (Fig. 1).
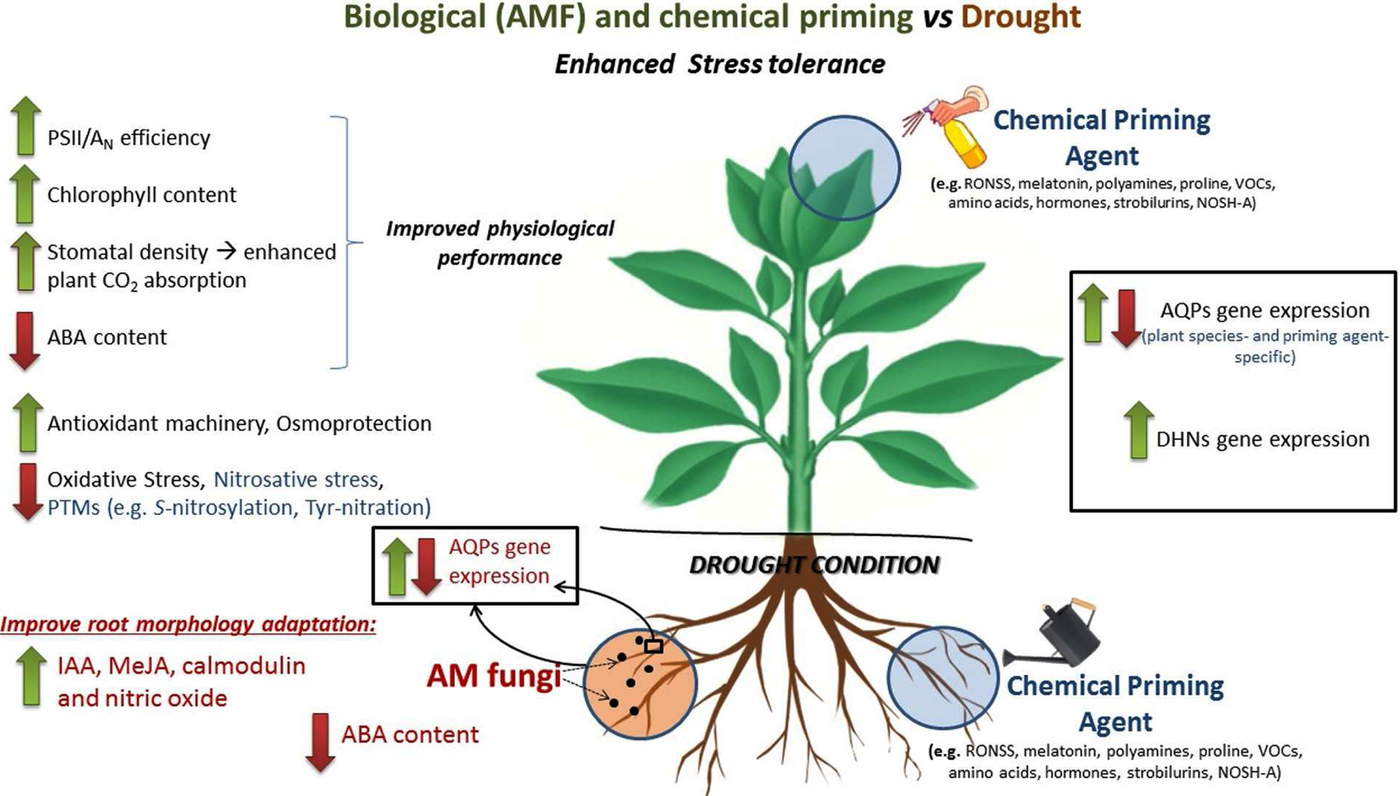
Fig. 1. Biochemical, molecular and phenotypic changes induced by arbuscular mycorrhizal (AM) symbiosis and chemical priming under water deficit conditions. Letters in black are for common defence mechanisms, in blue just for chemical priming and red for AM fungi (based on existing literature). For the AM symbiosis, responses also depend on the plant–AM fungus combinations (plant species/genotype-AM fungal species/isolate).
How arbuscular mycorrhizal fungi can improve plant tolerance to drought
It has been predicted that in future, plants will be exposed to more extreme abiotic stresses, such as drought events, and numerous studies have been carried out to develop sustainable techniques in agriculture (Chatzidaki & Ventura Reference Chatzidaki and Ventura2010). Plant-associated microbiota can increase plant tolerance to abiotic stresses such as flooding, drought, salinity, extreme temperatures and heavy metal contamination (Müller et al. Reference Müller, Vogel, Bai and Vorholt2016). Thanks to the ability of establishing mutualistic symbioses with the roots of most crops, AM fungi can have an important role as bio-fertilizing microorganisms (Berruti et al. Reference Berruti, Lumini, Balestrini and Bianciotto2015). Furthermore, AM fungi have been reported to improve plant tolerance to important abiotic environmental conditions such as drought, salt stress and cold (Porcel et al. Reference Porcel, Aroca and Ruíz-Lozano2012; Rapparini & Peñuelas Reference Rapparini, Peñuelas and Miransari2014; Lenoir et al. Reference Lenoir, Fontaine and Sahraoui2016; Pedranzani et al. Reference Pedranzani, Rodríguez-Rivera, Gutiérrez, Porcel, Hause and Ruiz-Lozano2016; Balestrini et al. Reference Balestrini, Chitarra, Fotopoulos, Ruocco, Lukac, Gamboni and Grenni2017). The development of plants with high productivity and survival rate under drought conditions is the main goal of crop breeding programmes (Osakabe et al. Reference Osakabe, Osakabe, Shinozaki and Tran2014). Water use efficiency (WUE), which can be calculated as the amount of CO2 taken up by photosynthesis (A N) divided by the amount of water transpired (E), represents a key parameter of plant performance under water stress and is an important selection trait (Osakabe et al. Reference Osakabe, Osakabe, Shinozaki and Tran2014). In this context, AM fungal colonization has shown noteworthy results in increasing tolerance to water stress (Augé et al. Reference Augé, Toler and Saxton2015). The impact of AM symbiosis on plant performance in conditions of water limitation has been studied extensively (Rapparini & Peñuelas Reference Rapparini, Peñuelas and Miransari2014; Lenoir et al. Reference Lenoir, Fontaine and Sahraoui2016; Balestrini et al. Reference Balestrini, Chitarra, Fotopoulos, Ruocco, Lukac, Gamboni and Grenni2017) and improved drought tolerance by AM fungal colonization has been reported for different plants, e.g. citrus, lettuce, maize, olive, pistachio, tomato, watermelon, wheat, Knautia arvensis and Robinia pseudoacacia (Abbaspour et al. Reference Abbaspour, Saeidi-Sar, Afshari and Abdel-Wahhab2012; Doubková et al. Reference Doubková, Vlasáková and Sudová2013; Wu et al. Reference Wu, Srivastava and Zou2013; Zhou et al. Reference Zhou, Ravnskov, Jiang and Wollenweber2015; Calvo-Polanco et al. Reference Calvo-Polanco, Sánchez-Castro, Cantos, García, Azcón, Ruiz-Lozano, Beuzón and Aroca2016; Mo et al. Reference Mo, Wang, Yang, Zheng, Liu, Li, Ma, Zhang, Wei and Zhang2016; He et al. Reference He, Sheng and Tang2017). The benefits of AM fungi have been observed across multiple scales, from soil structure to plant eco-physiological (e.g. hydraulic traits), molecular (e.g. stress marker genes) and biochemical responses (e.g. hormones and defence-related metabolites). A promotion of stomatal conductance to water vapour (g s) has been reported in different plant/AM fungi combinations independently from the soil moisture conditions, and the influence of AM symbiosis is more pronounced under drought (Augé et al. Reference Augé, Toler and Saxton2015). Recently, the positive effect of two AM fungi (Funneliformis mosseae and Rhizophagus intraradices) in tomato tolerance to water deficit has been reported. Interestingly, differences between the two fungal species have been observed (Chitarra et al. Reference Chitarra, Pagliarani, Maserti, Lumini, Siciliano, Cascone, Schubert, Gambino, Balestrini and Guerrieri2016), in agreement with observations that stress alleviation by AM fungal colonization may be environment- and symbiont-specific (Augé Reference Augé2001, Reference Augé2004; Augé et al. Reference Augé, Toler and Saxton2015; Eulenstein et al. Reference Eulenstein, Tauschke, Behrendt, Monk, Schindler, Lana and Monk2017). Arbuscular mycorrhizal symbiosis affected tomato eco-physiology parameters under severe water stress conditions (i.e. leaf water potential of about −1.3 MPa) with a positive impact on WUE values (Chitarra et al. Reference Chitarra, Pagliarani, Maserti, Lumini, Siciliano, Cascone, Schubert, Gambino, Balestrini and Guerrieri2016), in accordance with the net photosynthetic rate (AN). Under water deficit, an improved WUE (calculated as A N/E) in AM-colonized plants has been reported in Populus × canadensis ‘Neva’ plants colonized by R. irregularis (Liu et al. Reference Liu, Li, Hui, Tang and Zhang2016) as well as in lettuce (Ruiz-Lozano et al. Reference Ruiz-Lozano, Gómez and Azcón1995). However, several papers have also reported a positive impact of AM symbiosis on WUE calculated as the ratio between biomass and water consumed (reviewed in Aroca et al. Reference Aroca, Porcel, Ruiz-Lozano and Fulton2011). Improved efficiency in photosystem II (PSII) promoted by AM symbiosis was also observed in maize and tomato water-stressed plants (Bárzana et al. Reference Bárzana, Aroca, Paz, Chaumont, Martinez-Ballesta, Carvajal and Ruiz-Lozano2012; Ruiz-Lozano et al. Reference Ruiz-Lozano, Aroca, Zamarreño, Molina, Andreo-Jiménez, Porcel, García-Mina, Ruyter-Spira and López-Ráez2016), as well as an increased chlorophyll content in basil (Ocimum gratissimum; Hazzoumi et al. Reference Hazzoumi, Moustakime, Elharchli and Amrani Joutei2015). The levels of ABA, which is a hormone involved in the regulation of plant growth and development as well as in responses to abiotic stresses, are also affected by AM symbiosis. Under a severe water stress treatment, AM-colonized tomato plants showed a significantly lower ABA content (measured both in roots and in leaves at the end of the experimental period) with respect to non-colonized plants, suggesting that the latter probably faced more intense water stress than the colonized ones (Chitarra et al. Reference Chitarra, Pagliarani, Maserti, Lumini, Siciliano, Cascone, Schubert, Gambino, Balestrini and Guerrieri2016). This is in agreement with data reported by Li et al. (Reference Li, Sun, Ruan, Xu, Hu, Hao, Zhang, Li, Wang, Yang and Chen2016) in Zea mays inoculated with R. intraradices: these authors demonstrated that AM fungal colonization can decrease root ABA content mainly by down-regulating aldehyde oxidase (AO) expression under water deficit (Li et al. Reference Li, Sun, Ruan, Xu, Hu, Hao, Zhang, Li, Wang, Yang and Chen2016, Reference Li, Zhang and Chen2017). Interestingly, tomato root colonization resulted in a significant increase in stomatal density measured in leaves from irrigated plants, mainly for R. intraradices-colonized plants (Chitarra et al. Reference Chitarra, Pagliarani, Maserti, Lumini, Siciliano, Cascone, Schubert, Gambino, Balestrini and Guerrieri2016). Since an increased stomatal density may enhance plant CO2 absorption capacity, these data are in accordance with the fact that AM-colonized plants showed significantly greater AN, which is correlated directly with WUE values, in comparison with non-colonized ones not only upon drought but also under well-watered conditions (Chitarra et al. Reference Chitarra, Pagliarani, Maserti, Lumini, Siciliano, Cascone, Schubert, Gambino, Balestrini and Guerrieri2016). Ruiz-Lozano et al. (Reference Ruiz-Lozano, Aroca, Zamarreño, Molina, Andreo-Jiménez, Porcel, García-Mina, Ruyter-Spira and López-Ráez2016) also demonstrated a correlation between AM root colonization, strigolactone (SL) levels and drought severity. It is worth noting that water-related stresses influence SL production negatively, while the presence of AM fungi seems to have the opposite effect (Aroca et al. Reference Aroca, Ruiz-Lozano, Zamarreño, Paz, Garcia-Mina, Pozo and Lopez-Raez2013; López-Ráez Reference López-Ráez2016; Ruiz-Lozano et al. Reference Ruiz-Lozano, Aroca, Zamarreño, Molina, Andreo-Jiménez, Porcel, García-Mina, Ruyter-Spira and López-Ráez2016). It has been suggested that, due to the role of SLs in AM symbiosis establishment (López-Ráez Reference López-Ráez2016), plants increase SL production under stressed conditions to promote symbiosis establishment, thus improving responses to stress (Ruiz-Lozano et al. Reference Ruiz-Lozano, Aroca, Zamarreño, Molina, Andreo-Jiménez, Porcel, García-Mina, Ruyter-Spira and López-Ráez2016). Furthermore, trifoliate orange plants colonized by Diverispora versiformis showed improved root morphology adaptation in response to drought stress, probably correlated with AM-induced changes in root indole-3-acetic acid (IAA), methyl jasmonate (MeJA), calmodulin and nitric oxide levels, enhancing tolerance to water deprivation (Zou et al. Reference Zou, Wang, Liu, Ni, Zhang and Wu2017).
Arbuscular mycorrhizal symbiosis can also affect the content of molecules involved in osmoregulation and the activity of antioxidant enzymes in several plant/AM fungus interactions subjected to water-related stresses (Liu et al. Reference Liu, Li, Hui, Tang and Zhang2016; Mo et al. Reference Mo, Wang, Yang, Zheng, Liu, Li, Ma, Zhang, Wei and Zhang2016; Huang et al. Reference Huang, Zou and Wu2017; Wu et al. Reference Wu, Zou, Rahman, Ni and Wu2017), although results can be different probably in relation to the species involved in the symbiosis as well as the stress severity and duration. Additionally, the presence of the AM fungus can lead to a significant reduction in ROS (e.g. hydrogen peroxide (H2O2)) accumulation (Chitarra et al. Reference Chitarra, Pagliarani, Maserti, Lumini, Siciliano, Cascone, Schubert, Gambino, Balestrini and Guerrieri2016; Liu et al. Reference Liu, Li, Hui, Tang and Zhang2016), which often increase under drought. Using a split-root system to obtain maize AM-colonized plants with either the whole of the root system colonized or only half of it and to induce drought affecting the whole plant or only half of the root system, Bárzana et al. (Reference Bárzana, Aroca and Ruiz-Lozano2015) demonstrated an impact of AM colonization on the accumulation of compounds with a role in osmoregulation and aquaporins (AQPs), as well as on the antioxidant apparatus. The authors suggested that the positive impact of AM symbiosis is not only due to decreased oxidative stress in the host plants but also to a localized restriction of such oxidative stress (Bárzana et al. Reference Bárzana, Aroca and Ruiz-Lozano2015). Nonetheless, although different mechanisms might be involved in water stress alleviation in AM plants (Ruiz-Lozano Reference Ruiz-Lozano2003; Ruiz-Lozano et al. Reference Ruiz-Lozano, Porcel and Aroca2006; Rapparini & Peñuelas Reference Rapparini, Peñuelas and Miransari2014; Ruiz-Lozano & Aroca Reference Ruiz-Lozano, Aroca, Chaumont and Tyerman2017), several aspects of the enhanced drought tolerance in plants inoculated by AM fungi still remain to be fully clarified.
Water transport and regulation of plant and fungal aquaporin genes in arbuscular mycorrhizal-colonized roots
The thin AM fungal hyphae can explore soil pores inaccessible to root hairs, thus reaching water sources not available to non-AM plants. Furthermore, hyphal water transport to the root under drought conditions has been demonstrated previously (Khalvati et al. Reference Khalvati, Hu, Mozafar and Schmidhalter2005; Ruth et al. Reference Ruth, Khalvati and Schmidhalter2011), as well as the fact that AM-colonized plants are able to take up more water from the soil than non-colonized ones (Marulanda et al. Reference Marulanda, Azcón and Ruiz-Lozano2003). Ruiz-Lozano & Azcón (Reference Ruiz-Lozano and Azcón1995) reported that AM fungal hyphae could take up water, but there were considerable variations in the behaviour of two different AM fungi as well as in the mechanisms involved in their impact on plant-water relations. For several years, numerous studies focused on highlighting the impact of AM symbiosis on root hydraulic conductivity (L), which provide indications about the plant's capacity to take up water from the soil, suggesting that the decrease observed under drought may be partially reduced by AM symbiosis (Bárzana et al. Reference Bárzana, Aroca, Paz, Chaumont, Martinez-Ballesta, Carvajal and Ruiz-Lozano2012; El-Mesbahi et al. Reference El-Mesbahi, Azcón, Ruiz-Lozano and Aroca2012). Interestingly, Sánchez-Romera et al. (Reference Sánchez-Romera, Ruiz-Lozano, Zamarreño, García-Mina and Aroca2016) suggested that, under mild water stress, AM symbiosis prevents the inhibition of root hydraulic conductivity (L) in bean plants, and that this effect could be due to a reduction in the salicylic acid (SA) level in roots caused by drought. Additionally, the increase in L in AM-colonized bean plants under drought conditions was accompanied by an increase in the expression of a plant (i.e. plasma membrane intrinsic proteins, PIPs) and two fungal aquaporin (AQP) genes as well as an increase in the amount of phosphorylated proteins (PIP2Ph) in AM-colonized plants (Sánchez-Romera et al. Reference Sánchez-Romera, Ruiz-Lozano, Zamarreño, García-Mina and Aroca2016). The authors suggested that phosphorylation of PIP2 proteins can regulate L values with respect to environmental conditions. Aquaporins are membrane-intrinsic proteins that are involved in the control and regulation of passive water movement in different physiological conditions. In plants, AQPs belong to a large protein family with five major sub-groups divided on the base of their sequence similarity (Maurel et al. Reference Maurel, Boursiac, Luu, Santoni, Shahzad and Verdoucq2015). Plant AQPs are present in various tissues and their functions are central for plant growth and responses to abiotic stresses, playing determinant roles in hydraulic regulation in roots and leaves as well as in the plant response to several stimuli including drought and flooding (Maurel et al. Reference Maurel, Boursiac, Luu, Santoni, Shahzad and Verdoucq2015). An increased gene expression of AQP transcripts is also present in AM-colonized roots under non-stressed conditions (Uehlein et al. Reference Uehlein, Fileschi, Eckert, Bienert, Bertl and Kaldenhoff2007; Giovannetti et al. Reference Giovannetti, Balestrini, Volpe, Guether, Straub, Costa, Ludewig and Bonfante2012). For example, gene expression analysis has revealed that two putative Lotus japonicus aquaporin genes, namely LjNIP1 and LjXIP1, are both AM-responsive. Additionally, a good correlation between the expression of LjNIP1 and LjPT4, a phosphate transporter considered a marker gene of symbiosis functionality, has been reported (Giovannetti et al. Reference Giovannetti, Balestrini, Volpe, Guether, Straub, Costa, Ludewig and Bonfante2012) and LjNIP1 transcripts have been localized exclusively in arbuscule-containing cells as observed using laser microdissection (Giovannetti et al. Reference Giovannetti, Balestrini, Volpe, Guether, Straub, Costa, Ludewig and Bonfante2012), suggesting that LjNIP1 could be considered a novel molecular marker of mycorrhizal symbiosis. As demonstrated by functional complementation experiments, LjNIP1 seems to be involved in water, but apparently not ammonia, transport. Expression of plant AQP in mycorrhizal roots under drought conditions has also been assessed in several plant/AM fungi combinations (Porcel et al. Reference Porcel, Aroca, Azcón and Ruiz-Lozano2006; Aroca et al. Reference Aroca, Porcel and Ruiz-Lozano2007; Ruiz-Lozano et al. Reference Ruiz-Lozano, Alguacil, Bárzana, Vernieri and Aroca2009; Bárzana et al. Reference Bárzana, Aroca, Bienert, Chaumont and Ruiz-Lozano2014; Chitarra et al. Reference Chitarra, Pagliarani, Maserti, Lumini, Siciliano, Cascone, Schubert, Gambino, Balestrini and Guerrieri2016; He et al. Reference He, Zhang and Tang2016). As reported recently by Ruiz-Lozano & Aroca (Reference Ruiz-Lozano, Aroca, Chaumont and Tyerman2017), data originating from several studies indicate that AM symbiosis has an impact on host plant AQPs and alters both plant-water relationships and plant physiology in order to cope better with stressful environmental conditions such as drought. However, as also reported from other functional aspects related to AM symbiosis, the regulation of AQPs seems to be dependent on the plant and fungal species involved in the symbiosis (Ruiz-Lozano & Aroca Reference Ruiz-Lozano, Aroca, Chaumont and Tyerman2017). Bárzana et al. (Reference Bárzana, Aroca, Bienert, Chaumont and Ruiz-Lozano2014) analysed the expression of the whole set of maize aquaporin genes in AM-colonized roots under several growing and water-stressed conditions: they demonstrated that AM symbiosis can regulate a large number of AQP genes in the host plants in several sub-families and that the regulation of these genes is dependent on water status and the severity of the imposed stress. Furthermore, some of them have been characterized functionally to transport water and other molecules with a physiological importance in plant performance, suggesting that the improved AM-colonized plant performance under drought might be related not only to the movement of water but also to the mobilization of compounds with a role in plant performance (Bárzana et al. Reference Bárzana, Aroca, Bienert, Chaumont and Ruiz-Lozano2014). In tomato, three AQP genes belonging to three different sub-families (NIPs, NOD26-like intrinsic proteins; PIPs, plasma membrane intrinsic proteins; TIPs, tonoplast intrinsic proteins) have been reported to be expressed mainly or exclusively in roots (Reuscher et al. Reference Reuscher, Akiyama, Mori, Aoki, Shibata and Shiratake2013). All the selected genes showed an increasing trend of expression in roots from AM-colonized plants under irrigated conditions (Chitarra et al. Reference Chitarra, Pagliarani, Maserti, Lumini, Siciliano, Cascone, Schubert, Gambino, Balestrini and Guerrieri2016), while water stress treatment affected the transcriptional pattern of these aquaporin genes differently. LeNIP3;1 remained to be upregulated in AM-colonized plants, while LeTIP2.3 and LePIP1.1 were expressed less under severe water stress, in accordance with Bárzana et al. (Reference Bárzana, Aroca, Bienert, Chaumont and Ruiz-Lozano2014) who showed different regulation of AQP genes depending on the water status. Arbuscular mycorrhizal fungal AQPs can also have a role in helping AM fungi to resist drought stress as well as in the plant's tolerance to drought (Aroca et al. Reference Aroca, Bago, Sutka, Paz, Cano, Amodeo and Ruiz-Lozano2009; Li et al. Reference Li, Hu, Hao, Li, Wang and Chen2013a, Reference Li, Hu, Hao, Li and Chenb). After identification of the first AQP in an AM fungus (Aroca et al. Reference Aroca, Bago, Sutka, Paz, Cano, Amodeo and Ruiz-Lozano2009), Li et al. (Reference Li, Hu, Hao, Li, Wang and Chen2013a) identified and characterized two additional genes in R. irregularis (RiAQPF1 and RiAQPF2). A potential water transport via AM fungus to the host plant has been proposed looking at the gene expression profiles for the two functionally characterized R. irregularis AQP genes (Li et al. Reference Li, Hu, Hao, Li, Wang and Chen2013a, Reference Li, Hu, Hao, Li and Chenb). These fungal genes were both found to be activated in maize arbuscule-containing cells under drought. In addition, expression analysis of the two R. intraradices AQP genes RiAQPF1 and RiAQPF2 in tomato plants subjected to severe water stress conditions showed a significant upregulation of RiAQPF2 under water stress (Chitarra et al. Reference Chitarra, Pagliarani, Maserti, Lumini, Siciliano, Cascone, Schubert, Gambino, Balestrini and Guerrieri2016), thus supporting the theory of a direct AM fungus involvement in plant tolerance to drought. The discrepancy between the two experiments might be related to the fact that water stress was induced using polyethylene glycol (PEG) to simulate water deficit conditions and through a suspension of water supply, respectively. However, further studies aimed at functional characterization of the several AQPs regulated by AM symbiosis, both at local and systemic level, will be required to highlight the role of these proteins in drought tolerance by AM symbiosis. The characterization of fungal AQPs from other AM fungi could also be useful to identify fungal species/isolates more efficient to improve drought tolerance in host plants.
Improvement of plant tolerance to drought with the use of chemical priming agents
Chemical priming with the use of natural or synthetic compounds offers a cost-effective (i.e. low-cost chemical compounds used at low concentrations) methodology for the amelioration of abiotic stress-induced damage, suggesting a low cost–benefit ratio if applied in crop stress management (Antoniou et al. Reference Antoniou, Savvides, Christou and Fotopoulos2016). The increasing attention this approach has been receiving can be attested by the fact that >130 research articles have been published since 2012 where chemical priming approaches were followed towards the mitigation of drought stress in plant studies (source: http://www.scopus.com).
Chemical priming is characterized by its broad nature in terms of compounds, methods of application, tissue in which it is applied and so on (Savvides et al. Reference Savvides, Ali, Tester and Fotopoulos2016). In regard to the type of compounds that demonstrate priming activity, many natural molecules have the potential to act as a priming agent against a range of different abiotic stresses including amino acids (e.g. proline, Islam et al. Reference Islam, Hoque, Okuma, Banu, Shimoishi, Nakamura and Murata2009), hormones (e.g. salicylic acid, Li et al. Reference Li, Hu, Du, Tang, Shen and Wu2014), reactive oxygen–nitrogen–sulphur species (RONSS, Tanou et al. Reference Tanou, Fotopoulos and Molassiotis2012a, Reference Tanou, Filippou, Belghazi, Job, Diamantidis, Fotopoulos and Molassiotisb; Christou et al. Reference Christou, Manganaris, Papadopoulos and Fotopoulos2013), polyamines (Tanou et al. Reference Tanou, Ziogas, Belghazi, Christou, Filippou, Job, Fotopoulos and Molassiotis2014), melatonin (Antoniou et al. Reference Antoniou, Chatzimichail, Xenofontos, Pavlou, Panagiotou, Christou and Fotopoulos2017), volatile organic compounds (VOCs; Cho et al. Reference Cho, Kang, Han, Anderson, Park, Lee, Cho, Yang, Ryu and Kim2008) and even water (i.e. hydropriming; Casenave & Toselli Reference Casenave and Toselli2007). Interestingly, synthetic chemistry can also be employed to produce powerful priming agents, with examples including the fungicidal compounds strobilurins (Filippou et al. Reference Filippou, Antoniou, Obata, Harokopos, Van Der Kelen, Kanetis, Aidinis, Van Breusegem, Fernie and Fotopoulos2016), as well as NOSH-aspirin (NBS-1120), a novel nitric oxide (NO)- and hydrogen sulphide (H2S)-releasing hybrid, which was formulated initially as an anti-cancer drug but also displays protective effects against drought stress in plants (Antoniou et al. Reference Antoniou, Chatzimichail, Kashfi and Fotopoulos2014; Kashfi & Fotopoulos Reference Kashfi and Fotopoulos2015).
Most of these compounds are characterized by a commonly found complexity of their modus operandi, frequently modifying multiple defence-related pathways simultaneously. Several recent reviews have appeared in light of the constantly increasing interest in chemical priming against abiotic stress conditions including drought, which provide a state-of-the-art overview of the molecular adjustments and tolerance mechanisms that are altered following priming phenomena (Perez & Brown Reference Perez and Brown2014; Balmer et al. Reference Balmer, Pastor, Gamir, Flors and Mauch-Mani2015; Paparella et al. Reference Paparella, Araújo, Rossi, Wijayasinghe, Carbonera and Balestrazzi2015; Antoniou et al. Reference Antoniou, Savvides, Christou and Fotopoulos2016; Merewitz Reference Merewitz, Hossain, Wani, Bhattacharjee, Burritt and Tran2016; Savvides et al. Reference Savvides, Ali, Tester and Fotopoulos2016; Wojtyla et al. Reference Wojtyla, Lechowska, Kubala and Garnczarska2016). Enhancing photosynthetic performance is an important parameter for plant survival under adverse environmental conditions, such as drought and salinity. Many studies have demonstrated that primed plants conserved photosynthetic performance at higher levels than non-treated plants under stressful conditions (Chen et al. Reference Chen, Wu, Wang, Zheng, Lin, Dong, He, Pei and Zheng2011; Christou et al. Reference Christou, Manganaris, Papadopoulos and Fotopoulos2013, Reference Christou, Manganaris and Fotopoulos2014; Filippou et al. Reference Filippou, Antoniou, Obata, Harokopos, Van Der Kelen, Kanetis, Aidinis, Van Breusegem, Fernie and Fotopoulos2016; Antoniou et al. Reference Antoniou, Chatzimichail, Xenofontos, Pavlou, Panagiotou, Christou and Fotopoulos2017). This is manifested by enhancing chlorophyll content (Christou et al. Reference Christou, Manganaris and Fotopoulos2014), regulating proteins and genes encoding enzymes implicated in photosynthesis processes and chloroplast development (Chen et al. Reference Chen, Wu, Wang, Zheng, Lin, Dong, He, Pei and Zheng2011; Wei et al. Reference Wei, Li, Chu, Reiter, Yu, Zhu, Zhang, Ma, Lin, Zhang and Chen2015), as well as affecting processes related to stomatal movements (García-Mata & Lamattina Reference García-Mata and Lamattina2001; Fan & Liu Reference Fan and Liu2012).
In any case, close examination of the existing literature reveals certain shared targets of chemical priming agents towards acquired tolerance to water deficit. First, upregulation of the antioxidant apparatus (which includes both enzymatic and non-enzymatic antioxidants) and resulting ROS detoxification. For example, Christou et al. (Reference Christou, Manganaris and Fotopoulos2014) showed that H2S-primed strawberry plants under non-ionic hyperosmotic stress were more tolerant compared with non-primed, stressed samples. This protection was manifested via lower levels of synthesis of H2O2 in leaves and the maintenance of high ascorbate and glutathione redox states, while differential expression levels of key ascorbate and glutathione biosynthesis (GCS, GDH, GS) transcripts were also observed. Furthermore, as a consequence of chemical treatment, increased biosynthesis of osmoprotective molecules such as soluble carbohydrates and proline has been observed. Shi et al. (Reference Shi, Jiang, Ye, Tan, Reiter, Zhang, Liu and Chan2015) provided metabolomic evidence that pre-treatment of bermudagrass with exogenous melatonin prior to drought stress imposition lead to a significant increase in the concentration of proline as well as a number of sugars and sugar alcohols, correlating with increased overall performance under stress. It has also been suggested that post-translational protein modifications and nitrosative homeostasis may be regulated by chemical priming molecules. Ziogas et al. (Reference Ziogas, Tanou, Belghazi, Filippou, Fotopoulos, Grigorios and Molassiotis2015) demonstrated that PEG-treated sour orange plants (thus simulating drought stress in hydroponic culture) had lower damage levels following priming with NO and H2S through Tyr-nitration and S-nitrosylation of a number of proteins, including well-known and novel targets. A recent report by Antoniou et al. (Reference Antoniou, Chatzimichail, Xenofontos, Pavlou, Panagiotou, Christou and Fotopoulos2017) also revealed that priming of alfalfa plants with melatonin prior to severe drought stress leads to significant protection through the suppression of reactive nitrogen species (RNS) content by regulating reactive nitrogen species metabolic enzymes (nitrate reductase, NR; NADH dehydrogenase) at the enzymatic and/or transcript level.
On a molecular level, application of priming agents prior to stress exposure results in many transcriptional modifications which can be specific, or not, to the agent used. Several of the priming agents are characterized as signalling molecules, such as RONSS (Molassiotis & Fotopoulos Reference Molassiotis and Fotopoulos2011; Hancock & Whiteman Reference Hancock and Whiteman2016). This feature results from their ability to regulate the expression of essential genes, such as those implicated in antioxidant machinery (Christou et al. Reference Christou, Manganaris and Fotopoulos2014; Fotopoulos et al. Reference Fotopoulos, Antoniou, Filippou, Mylona, Fasoula, Ioannides and Polidoros2014; Antoniou et al. Reference Antoniou, Chatzimichail, Xenofontos, Pavlou, Panagiotou, Christou and Fotopoulos2017), RONS biosynthesis and scavenging (Christou et al. Reference Christou, Manganaris, Papadopoulos and Fotopoulos2013; Ziogas et al. Reference Ziogas, Tanou, Belghazi, Filippou, Fotopoulos, Grigorios and Molassiotis2015), cell homeostasis (Christou et al. Reference Christou, Manganaris and Fotopoulos2014; Lai et al. Reference Lai, Mao, Zhou, Li, Wu, Zhang, He, Cui and Xie2014) and osmoprotection (Ishibashi et al. Reference Ishibashi, Yamaguchi, Yuasa, Iwaya-Inoue, Arima and Zheng2011), as well as inhibition of proteolysis (Filippou et al. Reference Filippou, Antoniou, Obata, Harokopos, Van Der Kelen, Kanetis, Aidinis, Van Breusegem, Fernie and Fotopoulos2016), towards enhancement of plant tolerance against stresses. Soybean plants treated with H2O2 showed higher expression of genes implicated in oligosaccharide biosynthesis which are known osmoprotective molecules, such as GmMIPS2 (i.e. myo-inositol synthase) and GmGolS (i.e. galactinol synthase) under drought stress (Ishibashi et al. Reference Ishibashi, Yamaguchi, Yuasa, Iwaya-Inoue, Arima and Zheng2011). Another recent study highlights the potential use of the agrochemical fungicide kresoxim-methyl, which can act as a priming agent by regulating the expression of genes implicated in protein hydrolysis and enhance plant tolerance against drought and salinity. More information about gene regulation after RONSS application can be found in a recently published review by Antoniou et al. (Reference Antoniou, Savvides, Christou and Fotopoulos2016).
Water transport and regulation of plant aquaporin and dehydrin genes in chemical agent-primed tissues
Several reports have linked protection of plants against water deficit conditions with chemical priming via the regulation of AQP and dehydrin (DHN) expression levels. Together with AQPs (see previously), DHNs are key players in the survival of cells under water deficit conditions in order to counteract hyperosmotic stress and maintain turgor pressure; they are thought to be involved in the stabilization of both macromolecules and membranes (Wang et al. Reference Wang, Vinocur and Altman2003). Pandey et al. (Reference Pandey, Ansari, Tula, Sahoo, Bains, Kumar, Tuteja and Shukla2016) carried out a comprehensive study where treatment of rice plants with Ocimum sanctum leaf extracts resulted in improved tolerance to drought stress, correlating this improved tolerance with suppressed expression of AQP genes and induced expression of DHN genes. This supported previously observed positive correlations of DHN expression and negative correlations of AQPs with drought tolerance, the latter generally supported by several reports employing genetic modification approaches (Pandey et al. Reference Pandey, Ansari, Tula, Sahoo, Bains, Kumar, Tuteja and Shukla2016). Similarly, spermine pre-treatment of white clover prior to drought stress imposition resulted in the alleviation of negative effects caused by the stressor, linked with DHN accumulation and the transcription of genes encoding DHNs (Li et al. Reference Li, Jing, Peng, Zhang, Ma, Huang and Yan2015). Priming of drought-stressed wheat plants with 24-epibrassinolide also resulted in improved tolerance compared with non-primed, stressed plants correlating with increased accumulation of DHN protein levels (Shakirova et al. Reference Shakirova, Allagulova, Maslennikova, Fedorova, Yuldashev, Lubyanova, Bezrukova and Avalbaev2016). In addition, exogenous application of selenium and silicon in drought-stressed rice plants has been shown to lead to enhanced growth, while gene expression levels of a DHN were significantly upregulated in primed, stressed plants (Khattab et al. Reference Khattab, Emam, Emam, Helal and Mohamed2014). Finally, a report by Chen et al. (Reference Chen, Fessehaie and Arora2012) provided solid evidence that spinach seed osmopriming results in improved tolerance to desiccation by altering DHN metabolism as evidenced by increased protein and transcript levels.
Interestingly, the positive correlation of DHN involvement in the regulation of water transport and the general response of primed plants under water deficit conditions is observed uniformly, whereas AQPs provide contradictory results in some cases. Chen et al. (Reference Chen, Shang, Wang, Chen, He, Zheng and Shangguan2016) showed that H2S-mediated drought tolerance in spinach seedlings was linked with increased expression levels of SoPIP1;2, while Shi et al. (Reference Shi, Zhang, Han, Feng, Hu, Guo and Gong2016) demonstrated that enhanced water stress tolerance in tomato plants following silicon application was not linked with the transcriptional regulation of plasma membrane aquaporin genes, as mRNA levels were not obviously changed by Si under water stress. In contrast, Chen et al. (Reference Chen, Fessehaie and Arora2013) presented findings where aquaporin transcript levels exhibited higher expression in osmoprimed spinach seeds that also had greater drought tolerance. It is possible that AQP-related responses are isoform-specific, as these are usually members of multigene families and evidence suggests that regulation is variable. For example, Sánchez-Romera et al. (Reference Sánchez-Romera, Ruiz-Lozano, Zamarreño, García-Mina and Aroca2016) carried out a MeJA pre-treatment in Phaseolus vulgaris plants prior to drought stress conditions, and comprehensive reverse transcription quantitative polymerase chain reaction (RT–qPCR) analysis revealed that the expression of certain PIP genes was not regulated, PvPIP2;2 was suppressed in MJ-treated, stressed plants compared with non-primed, stress plants, while PvPIP1;3 was induced.
Conclusions
Priming plants against abiotic stresses using biological (i.e. AM fungi) and chemical (natural or synthetic) agents is a promising sustainable method in crop stress management. Although several recent reports have highlighted some of the physiological and molecular mechanisms involved in the enhanced tolerance/resistance, additional efforts are required mainly with the aim of optimizing these strategies and verifying the effects in field trials following real production management procedures. Moreover, an important point is that the impact of AM symbiosis on plant tolerance may depend on the plant species/genotype considered (i.e. genotypes with different drought tolerance levels) as well as on the AM fungal species/isolate. The identification of AM fungal species/isolates physiologically and genetically adapted to the stress conditions in a specific environment might also improve the use of these microorganisms. Additionally, the possibility of using root-associated microorganisms in combination with a chemical agent could be proposed and evaluated against an individual as well as combined abiotic stress factors, and further analyses should be devoted to verifying the impact of both strategies on the natural environment, e.g. on native microbial communities (in soil and plant). Further studies, combining both methods, are needed to verify that the two strategies can be complementary, thus rendering the application potential of this combined approach as potentially very promising. Notably, potential preparation and use of novel formulations containing both AM fungi and chemical priming molecules should examine the effect of the latter on the AM fungi in order to verify a synergistic/additive rather than a competitive interaction. Finally, the increased potential of priming at the seed stage should be highlighted and explored further, in line with well-recorded commercial interest, as the application of biological and/or chemical priming at this stage appears to be desirable considering reduced application costs and prolonged potential protection through epigenetic modifications (Vannier et al. Reference Vannier, Mony, Bittebière and Vandenkoornhuyse2015).