Introduction
Precambrian crystalline rock is well exposed in northeastern Minnesota, along the international border with Canada and in west-central Minnesota along the Minnesota River valley (Fig. 1). Elsewhere, Precambrian rock is buried by glacial sediment and Cretaceous and Paleozoic marine strata. Where buried by Cretaceous strata, the crystalline rock typically retains a thick weathering profile that developed on land prior to Late Cretaceous time (Reference ParhamParham, 1970). Where buried by Quaternary deposits alone, the saprolitic cap is partially eroded to a highly erratic preserved thickness that ranges from near zero to 60 or more meters.

Fig. 1. Study area and site locations along Minnesota River valley. Precambrian bedrock exposure, black (adapted from, Reference MoreyMorey, 1981); areas where Precambrian rock is overlain by sub-crop of Upper Cretaceous units, gray(adapted from Patterson, in press).
The bedrock-dominated regions of Minnesota have been interpreted as glacial landscapes of areal scour, the bedrock differentially eroded relative to the strength of contrasting rock types. The control exerted by bedrock type and structure is evident in the shape and trend of hills and lakes, especially in the border-lakes region of northeastern Minnesota (Zum-Reference Zumbergeberge, 1952; Reference Wright, Sims and MoreyWright, 1972). It is difficult to assess how much glacial erosion has taken place. In principle, the main effect of glaciation can be merely the stripping away of previously weathered rock to reveal a chemically etched, fresh-rock surface. Glacial erosion of fresh rock may be limited to abrasion, predominantly on the stoss side of bedrock protuberances.
The hypothesis that chemical weathering, rather than glacial erosion, was the dominant geomorphological agent in creating weathered-shield areas has been proposed for Fennoscandia (Reference GjessingGjessing, 1967; Reference SödermanSöderman, 1985; Reference Kejonen, Keilosto and LahtiKejonen and others, 1988; Reference Lidmar-BergströmLidmar-Bergström, 1988, Reference Lidmar-Bergström1989, Reference Lidmar-Bergström1995, Reference Lidmar-Bergström1997; Reference LundqvistLundqvist, 1988; Reference Lidmar-BergströmLidmar-Bergström and others, 1997), Britain (Reference Hall and SugdenHall and Sugden, 1987; Reference SugdenSugden, 1989) and North America (Reference FeiningerFeininger, 1971; Reference Lehr and HobbsLehr and Hobbs, 1992). It is well supported by comparisons to areas of tropical weathering that have never been glaciated (e.g. Reference FeiningerFeininger, 1971; Reference Lidmar-BergströmLidmar-Bergström, 1989; Reference ThomasThomas, 1994).
A buried subsaprolite surface (the weathering front) is developed under tropical conditions. It is characterized by: (1) undulating relief and significant overdeepenings, due to the progression of chemical weathering irrespective of base level (Reference ThomasThomas, 1966; Reference FeiningerFeininger, 1971; Reference Lidmar-BergströmLidmar-Bergström, 1989); (2) roche moutonnée like forms (Reference Von Engelnvon Engeln, 1937; Reference LintonLinton, 1955; Reference LindströmLindstrom, 1988; Reference Lidmar-BergströmLidmar-Bergström, 1988); (3) rounded bedrock overhangs (Reference Lidmar-BergströmLidmar-Bergström, 1989); (4) stepped bedrock surfaces with overdeepenings (Reference BakkerBakker, 1965; Reference FeiningerFeininger, 1971); (5) cavernous weathering forms (Reference Kejonen, Keilosto and LahtiKejonen and others, 1988) ; and (6) small weathering pits (Twidale and Bourne, 1975). In the overlying saprolite, rounded boulders (corestones) can develop in situ (Reference Ruxton and BarryRuxton and Barry, 1957; Reference OllierOllier, 1969). Removal of the saprolite reveals a smoothly undulating bedrock surface, with rounded bedrock knobs and closed depressions scattered with well-rounded boulders. Such a surface can be misinterpreted as having been extensively scoured by ice, with the boulders transported in from a distant source.
By comparing three exposures along the Minnesota River valley (Fig. 1), we develop the hypothesis that glacial erosion in parts of the Canadian Shield in Minnesota was limited to the removal of weathered crystalline rock. The crystalline bedrock in these exposures (1) has been stripped and glacially striated; (2) has been stripped after glaciation by meltwater, but has not been in direct contact with glacial ice; and (3) has not been stripped and retains the saprolite cover. The geomorphological similarity of these three areas implies that chemical weathering was the principal influence on shaping the crystalline bedrock surface.
General Description of Studyarea
The Precambrian rocks exposed in the Minnesota River valley form the southern edge of the Superior Province of the Canadian Shield and are an Archean gneiss terrane composed of four distinct blocks of quartzofeldspathic gneiss and granitoid intrusions (Reference Southwick and ChandlerSouthwick and Chandler, 1996). Weathering penetrated to an average depth of 30 m, but the fresh-rock—weathered-rock interface is irregular and undulates with as much as 45 m of relief (Reference ParhamParham, 1970; Reference Setterholm and MoreySetterholm and Morey, 1989). Where preserved, the upper part of the weathering profile displays soil development in the form of a pisolitic lateritic clay. The profile underlies Cretaceous marine sedimentary rocks, which led to its preservation in much of the western part of the state (Fig. 1; Reference Setterholm and MoreySetterholm and Morey, 1989).
Glacial History
Although the study area was repeatedly glaciated during the Pleistocene, it was also a site of glacial erosion, especially during late-Wisconsinan ice advances. The truncated remains of much older glacial units are unconformably overlain by late-Wisconsinan surficial glacial sediments. In places, the lowest glacial sediment directly overlies fresh crystalline rock, suggesting that the earliest glacial advances locally eroded through the Cretaceous marine sedimentary rocks and saprolite (Reference Patterson and PattersonPatterson, 1997; Reference Patterson, Knaeble, Gran and PhippenPatterson and others, 1999).
The surficial glacial sediment in the Minnesota River basin was deposited by the late-Wisconsinan Des Moines lobe (Fig. 2a; Reference Matsch, Sims and MoreyMatsch, 1972), which advanced repeatedly (Reference Patterson and PattersonPatterson, 1997). It was during an advance of the Des Moines lobe that the granite at sites la, b, and c (Fig. 1) was last striated, as inferred from striae orientations and a preliminary interpretation of cosmogenic-isotope dating of the striated surface (personal communication from P. Bierman, 1998). The granite outcrops were probably then shallowly buried by glacial sediment before final exhumation by meltwater.
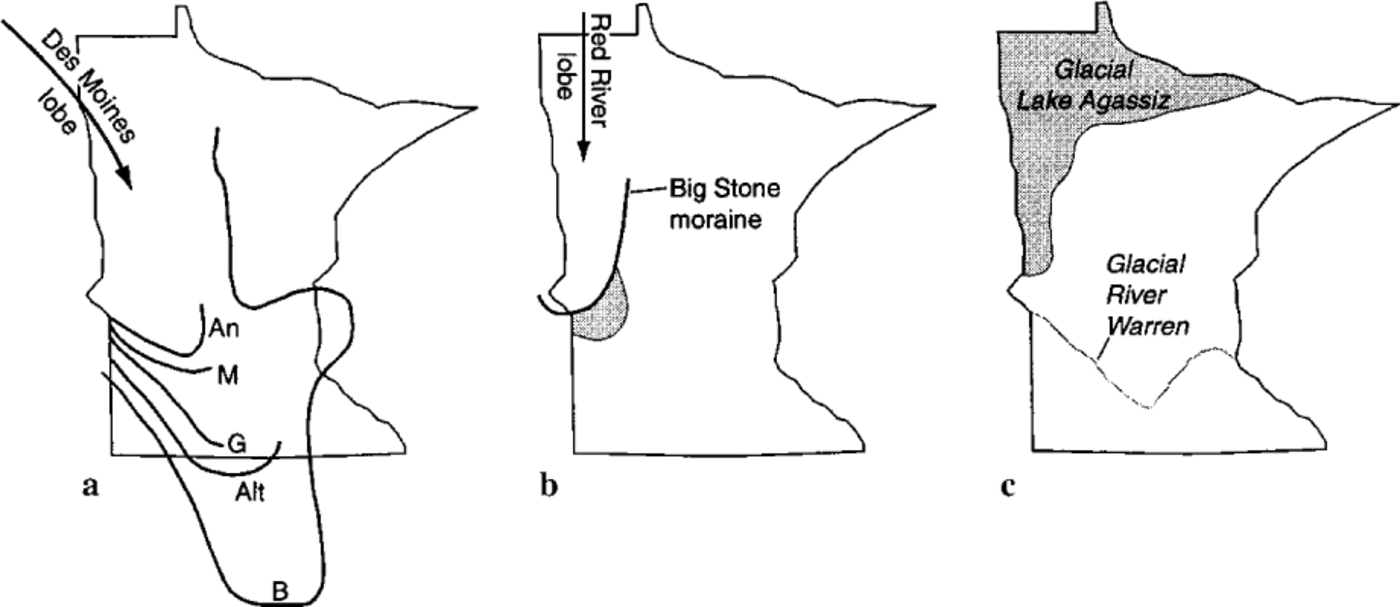
Fig. 2. General glacial geology of Minnesota: (a) moraines of the Des Moines lobe; (b) location of the Big Stone moraine and Glacial Lake Benson; (c) Glacial Lake Agassiz and Glacial River Warren, current trace of Minnesota River (adapted from Reference Patterson, Knaeble, Gran and PhippenPatterson and others, 1999).
An ice advance to the Big Stone moraine dated at 12 kyr BP terminated just north of site la (Fig. 2b). A large proglacial lake, Glacial Lake Agassiz, began to form as the ice retreated from the Big Stone moraine (Fig. 2c). For much of its existence, the lake was drained by Glacial River Warren, which flowed southeast from the southern tip of the lake (Reference UphamUpham, 1883, Reference Upham1896; Reference Matsch, Wright and Mayer-OakesMatsch and Wright, 1967). It was during the incision of River Warren that the striated bedrock at sites la, b, and c and the unstriated bedrock at sites 2a and b were exposed. Site 3 is not within the River Warren channel and the crystalline rock here has never been exposed; it remains 63 m below the surface.
Site Descriptions
Sites 1a, b, and c
Three localities that comprise site 1 were grouped because of proximity and similarity of bedrock type and exposure history. The largest area of outcrop, site la, best displays the undulating relief of the bedrock surface (Fig. 3). The granite is exposed in an upper terrace of River Warren from 304 m to 317 m a.s.l., a local high on the crystalline bedrock topography.

Fig. 3. Site la. Most extensive outcrop area, showing undulating nature of bedrock surface. View to south; ice advancedfrom the west (right).
Striae on the outcrops trend N40W to N35W (personal communication from D. L. Southwick, 1997). The steep, irregular bedrock outcrops are weakly streamlined in a similar direction. However, the linear shape of the outcrops is most likely inherited from bedrock structure. Brittle fractures oriented N30–50W and another orthogonal set oriented N55E control the shape of the outcrops. The outcrops are littered with rounded boulders of the same lithology.
At site 1b, bedrock crops out from 293–317 m a.s.l. in an upper terrace of River Warren. This outcrop is also a local crystalline bedrock high. The outcrop shape reflects the dominant lithologic fabric of the gneissic rock. Striae on the outcrops are oriented N18W (personal communication from D.L. Southwick, 1997) and N50W. They may reflect more than one ice-flow event or local variations in ice-flow direction around the bedrock protuberance. Vertical-cut faces in an abandoned quarry at site lb show how weathering has progressed along orthogonal joint sets and has begun rounding the joint-isolated blocks (Fig. 4). In another area of the quarry, recent sheeting of about a decimeter of rock has produced a parabolic bedrock surface of steep sides and rounded top that at first appears to have been glacially moulded (Fig. 5). However, the surface is not polished, but fresh, rough, and granular; sheeting probably occurred as the stone was being quarried, or shortly thereafter.

Fig. 4. Site 1b. Weathering, as seen in the quarry wall, has progressed along joints, isolating rounded-bedrock blocks.

Fig. 5. Site 1b. Recent sheeting of granite resulting from quarrying that has exposed afresh, parabolic rock surface.
Site 1c is a low-lying outcrop (293–296 m a.s.l.) along the valley wall of an upper terrace of River Warren, part of the same bedrock high as site 1b. Two small areas of striae (Fig. 6) on the outcrop trend N40W and N80W, again reflecting either minor variations in ice-flow direction or different ice- flow events. The long axes of the outcrops are roughly orthogonal to this trend, controlled by either joints, lithologic fabric, or both. This low, smooth outcrop has rounded overhangs (Fig. 7) and is strewn with rounded boulders of the same granite type. One area displays shallow weathering pits (Fig. 8).

Fig. 6. Site 1c. One of two small areas of striae; also note rounded boulders. Striae are unambiguously glacial in origin. Boulders may have been rounded chemically; their lithology is identical to that of the outcrop.

Fig. 7. Site 1c. Rounded bedrock overhang interpreted as a result of chemical weathering along joints.

Fig. 8. Site 1c. Shallow pits in rock surface interpreted as weathering pits, possibly even postglacial in age.
Sites 2a and b
Site 2 consists of two areas of outcrop that were exposed by River Warren. In site 2a, fractured granitic gneiss with hornblende andesite dikes (Reference Southwick and PattersonSouthwick and Patterson, 1998) is exposed from 284–305 m a.s.l. in the valley bottom. The dikes are more resistant to weathering, and their generally east— west trend is easily recognized. The irregular bedrock surface has many closed depressions, some of which are partly filled with river sediment. The imprint of fluvial erosion is apparent in the deep, vertical potholes and shallow scours on outcrop tops (Fig. 9). The scours may have originated as weathering pits, but they have been modified by flowing water.

Fig. 9. Site 2a. Shallow scour on outcrop tops interpreted as fluvially modified weathering pits.
Site 2b is also located in the valley bottom. Here, granite with zones displaying more gneissic fabric crops out from 280 m to 299 m a.s.l., another local bedrock high. In nearby shallow-to-bedrock areas, large rounded “boulders” are more likely in-situ bedrock knobs that have been stripped of weathered-rock fragments. The long axes of outcrops are oriented northwest and east-northeast, probably reflecting structural control. A shallow road cut in the tail of a bedrock outcrop, 1 km long, shows that the upper 3 m of bedrock is composed of interlocking, chemically rounded blocks having weathered rock along the joints (Fig. 10). Downstream areas of kaolinitic saprolite were protected by bedrock knobs from fluvial erosion.

Fig. 10. Site 2b. Road cut exposes 2-3 m of weathered rock in the tail of an east-trending bedrock ridge, 1km long.
Site 3
Although northeast of site 3 bedrock crops out in the river valley from 256–268 m a.s.l., site 3 itself is in the sub-surface. Test holes that were drilled to study the resource potential of buried kaolin have allowed contouring of the bedrock surface in 20 ft (about 6 m) intervals (Fig. 11; Setterholm and others, 1989). The contour map shows that the fresh-rock surface (the weathering front) is rounded and undulating with as much as 40 m of relief and is similar in appearance to nearby exposures. Bedrock in the valley floor is at about the same elevation, but appears to have steeper slopes that form narrower crests than the sub-surface topography. This is likely an artifact of the test-hole density and the assumptions made while interpolating between data points when contouring. The stratigraphy is exemplified by a hole drilled in the area that penetrated 13 m of glacial sediment, 13.7 m of Cretaceous strata, and 39 m of saprolite before encountering fresh crystalline rock at approximately 244 m a.s.l.

Fig. 11. Topography of the weathering front in the Precambrian bedrock surface, Redwood Falls area, Minnesota River valley, mapped by D. W. Lindgren (from Reference Setterholm and MoreySetterholm and others, 1989); bullets show test-hole locations.
Discussion
Recent articles noting the preservation of fragile glacial landscapes that survived subsequent glaciation (e.g. Reference KlemanKleman, 1994) suggest that some areas of the glacier bed can survive repeated glaciation with no significant erosion. It is important to separate the pre-existing effects of weathering from glacial erosion so the efficacy of glacial erosion is not overestimated. This is especially critical if the glacial landscape is used to infer the nature of subglacial processes for the purpose of modeling glacier flow (e.g. Reference SugdenSugden, 1978; Reference Clark and PollardClark and Pollard, 1998). The force required to remove loose grus from a surface is considerably different than that required to pluck and abrade fresh crystalline rock.
The three sites of bedrock exposure along the Minnesota River were chosen for their range of erosional histories in order to qualitatively evaluate the relative effect of chemical vs glacial erosion. Despite differences in rock type and exposure history, all three bedrock sites resemble each other in general morphology. The rock surfaces appear to have been shaped mainly by chemical weathering processes that took place while the surface was buried beneath a saprolite cover.
It is likely that glacial erosion had a more pronounced effect on the crystalline rock surface in northeastern Minnesota, where the Cretaceous marine strata were absent or thinner and glacial erosion could have exposed the surface earlier in the Pleistocene. This is also probably true for areas of the Canadian Shield in Canada, where the ice covered the area more continuously during the Pleistocene. But in the Minnesota River valley, where the bedrock was only recently subjected to subglacial and fluvial erosion, and where ice of at least the last advance was thin and the advances short-lived, the effects appear to be only a minor overprinting of the effects of chemical weathering.
Conclusions
In the upper Minnesota River valley, glacial advances have only eroded deep enough locally to expose the fresh Precambrian crystalline rock surface. Along the river valley, late-and post-glacial fluvial erosion have exposed the crystalline rock surface, in some cases for the first time. Elsewhere, the crystalline rock is buried and the complete stratigraphy, where preserved, includes a thick weathering residuum capped by Cretaceous marine strata overlain by varying thicknesses of glacial sediment. The features of these outcrops that we believe were inherited from chemical weathering include: (1) the smoothly rounded surface of the rock; (2) the steep sides; (3) closed depressions at all scales; (4) large linear grooves or highs, especially where aligned with structural or lithologic features such as joints, gneissic fabric, or dikes; and (5) rounded boulders of composition similar to the outcrops. Features of these outcrops that are most likely related to subglacial erosion include: (1)striae; (2) streamlining of the rock in a direction parallel to striae, but only convincingly glacial in origin if not parallel to structural control; and (3) polishing. However, polishing has been noted on the interior of potholes and in these locations, as well as on level rock surfaces, the polish has been created fluvially, not glacially. These sites serve as a caution to casual interpretation of exposed, undulating crystalline rock surfaces as due to glacial scouring. Care must be taken when incorporating evidence of subglacial erosion and bed conditions into models of glacier flow to avoid overestimating processes such as bedrock plucking, differential abrasion, and subglacial fluvial erosion.