Enhancement of skeletal muscle protein deposition is of vital importance for livestock meat production( Reference Shavlakadze and Grounds 1 ). Apart from being essential substrates for protein synthesis, branched-chain amino acids (BCAA; leucine (Leu), isoleucine (Ile) and valine (Val)), especially Leu, have been well demonstrated to be able to promote muscle anabolism by stimulating protein synthesis and inhibiting protein degradation( Reference Louard, Barrett and Gelfand 2 – Reference Columbus, Fiorotto and Davis 5 ). Recently, we found that the mass of most muscles was increased in young pigs with supplementation of BCAA to reduced-protein diet( Reference Zheng, Wei and Cheng 6 ). However, the mechanisms underlying the anabolic effect of BCAA on muscle growth remain largely unknown.
Postnatal growth of skeletal muscle depends on the balance between protein synthesis and degradation. That is to say, to increase the muscle mass, protein synthesis should exceed protein degradation (resulting in protein deposition)( Reference Shavlakadze and Grounds 1 ). Of note, the elevation of muscle protein synthesis does not always result in the increase of muscle mass( Reference Columbus, Steinhoff-Wagner and Suryawan 7 , Reference Manjarín, Columbus and Suryawan 8 ). Thus, only measuring protein synthesis may not be able to accurately and objectively present the mechanisms for the increase of muscle mass. As skeletal muscle primarily utilises amino acids (AA) for protein deposition, the increase of muscle net AA flux (calculated by arteriovenous difference technique) may contribute to the elevation of muscle protein deposition and mass. Therefore, it is of great scientific interest to measure AA flux across skeletal muscle in vivo and clarify in what ways AA flux can be regulated( Reference Jourdan, Deutz and Cynober 9 – Reference Thivierge, Bush and Suryawan 11 ). However, little has been known about the contribution of net AA flux to BCAA-induced increase of mass in skeletal muscle.
Apart from having a high protein synthesis capacity, skeletal muscle is also a major site for initiating extensive catabolism of BCAA( Reference Shimomura, Yamamoto and Bajotto 12 , Reference Harper, Miller and Block 13 ). Skeletal muscle catabolises BCAA for the synthesis of alanine, glutamate, glutamine, aspartate and asparagine( Reference Holeček 14 , Reference Li, Knabe and Kim 15 ). Among these AA, 97 % of glutamate, 95 % of aspartate and 67–70 % of glutamine in the diets are catabolised by small intestine( Reference Stoll and Burrin 16 , Reference Wu, Bazer and Burghardt 17 ). It seems that BCAA catabolism in muscle may promote the resynthesis of these three non-essential AA (NEAA) to modulate intramuscular AA balance, and thereby enhances muscle protein synthesis. In addition, Leu transamination product α-ketoisocaproic acid (KIC) can act as a nutrient signal to stimulate protein synthesis through activating translation initiation factors( Reference Columbus, Fiorotto and Davis 5 , Reference Escobar, Frank and Suryawan 18 ) and to inhibit protein degradation through down-regulating the expression of proteolytic-related genes in skeletal muscle( Reference Nakashima, Yakabe and Ishida 19 ). Thus, besides meeting the requirements for protein synthesis, supplementation of BCAA may affect protein turnover through their metabolism in skeletal muscle.
Taken together, we hypothesised that supplementation of BCAA to reduced-protein diet increases not only the arterial and intramuscular concentrations of BCAA but also their intramuscular catabolism, and thus contributes to elevated net AA fluxes across skeletal muscle, leading to the increase of muscle growth. Therefore, a hindlimb flux model was established by carotid artery, femoral artery and vein catheterizations to explore the impacts of BCAA supplementation on muscle net AA fluxes in young pigs fed reduced-protein diets. Metabolomics studies have been successfully applied to the investigation of the global changes of metabolites in animals in response to external stimuli (diet, diseases, drugs and environments), which could provide insights into the end points of metabolic fluxes in cells, tissues or the whole body( Reference Jones, Park and Ziegler 20 , Reference Paris, Johnson and Aguilar 21 ). In addition, hindlimb flux model enables the detection of net AA fluxes as well as specific AA metabolism in skeletal muscle. Therefore, the combination of metabolite profiling and AA flux model holds great promise for identifying potential metabolites and translating these metabolic changes into specific alterations in certain pathways at an organ level following a dietary intervention( Reference Brennan 22 ). Here, we performed a metabolomic analysis of femoral vein using HPLC-quadrupole time-of-flight MS (HPLC-QTOF-MS) method to obtain global metabolic profiles in the outflow from skeletal muscle, and correlated the muscle net AA fluxes with the arterial and intramuscular concentrations of BCAA and the net production of branched-chain α-keto acids (BCKA).
Methods
Animals and diets
The protocol of present study was approved by the Animal Care and Use Committee of College of Animal Sciences and Technology, Huazhong Agricultural University, and was carried out in accordance with the National Research Council’s Guide for the Care and Use of Laboratory Animals. In all, fourteen 28-d-old barrows (Large White×Landrace) were individually housed in metabolic cages, and randomly assigned to receive one of two experimental diets: the reduced-crude protein (CP) diets supplemented with (treatment) or without (control) BCAA (n 7 pigs/group), as we previously described( Reference Zheng, Wei and Cheng 6 ). The two diets were fortified with lysine (Lys), methionine (Met), threonine (Thr) and tryptophan (Trp) to satisfy the standardised ileal digestible (SID) AA requirements for pigs weighing 11–25 kg( 23 ), and had the same CP content of 16·7 %, which was 20 % lower than that recommended by the National Research Council (NRC)( 24 ). Control diet was formulated without addition of Val, Leu and Ile, but was supplemented with 0·42 % alanine, which therefore was deficient of Val and Ile. The contents of SID Val, Ile and Leu in control diet were 86·8, 91·4 and 107·2 % of the NRC( 23 ) recommended contents, respectively. The treatment diet was formulated by supplementing 0·17 % Ile, 0·24 % Leu and 0·16 % Val (all of 99 % purity; Amresco) to control diet to provide SID BCAA equal to the level in the diet with protein content (20 % CP) recommended by the NRC( 24 ). The ingredients of the diets and the amounts of added synthetic AA are shown in Table 1. All pigs had free access to feed and drinking water. Room temperature was maintained at 25–27°C. One pig from control group was excluded because of unexpected death due to unknown causes at the beginning of week 1.
Table 1 Composition of experimental diets (as-fed basis)
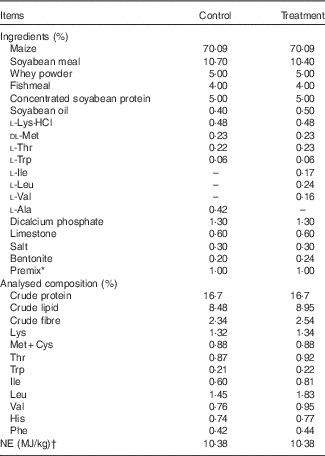
NE, net energy.
* Provided per kg of diet (as-fed basis): vitamin A, 45 00 µg; vitamin D3, 37·5 µg; vitamin E, 30 mg; vitamin B1, 3·2 mg; vitamin B2, 8 mg; vitamin B6, 4 mg; vitamin B12, 0·03 mg; vitamin K3, 3·2 mg; niacin, 34 mg; folate, 1·6 mg; pantothenic acid, 18 mg; biotin, 0·2 mg; choline chloride, 500 mg; Cu, 150 mg; Fe, 120 mg; Mn, 45 mg; Zn, 90 mg; I, 0·6 mg; Se, 0·3 mg; Co, 0·3 mg; chlortetracycline, 35 mg; tiamulin 12 mg.
† Values for NE were calculated according to National Research Council( 23 ).
The remaining thirteen pigs were weighed after a 4-week acclimation period, and the feed intake was determined daily. As for animal growth, our previous work showed that the final body weight (BW) and average daily feed intake of BCAA-supplemented pigs were significantly greater than that of control pigs( Reference Zheng, Wei and Cheng 6 ). Then, catheters were placed into one carotid artery, femoral artery and vein on the left side of body as described below. The femoral artery catheter was used for infusion of p-amino hippurate (PAH) to measure plasma flow across the hindlimb muscle. The carotid artery and femoral vein catheters were used for blood collection.
Surgery
After overnight food deprivation, pigs were surgically implanted with catheters in one carotid artery (2·41 mm outer diameter) under isoflurane anaesthesia and strict aseptic conditions as previously described( Reference Fang, Luo and Qi 25 , Reference Stoll, Henry and Reeds 26 ). The left femoral artery and vein were isolated, and cannulated with central venous catheters (1·4 mm outer diameter) as described by Ettrup et al.( Reference Ettrup, Glud and Orlowski 27 ). In brief, femoral artery and vein on the left side of body were identified in skin fold between gracilis and sartorius muscle where pulsation of superficial part of medial saphenous artery disappears. After making a longitudinal superficial skin incision cranial to this point, the underlying subcutaneous tissue was dissected using blunt-tip scissors. The two muscle groups were then separated with a small self-retaining tissue retractor. The artery was isolated for a length of approximately 1–2 cm using blunt dissection, and cannulated with central venous catheter. The femoral vein is located just medial to artery. After isolating the vein with blunt dissection, the vein was also cannulated. The catheters were subsequently flushed with heparinised saline in order to maintain catheter patency. The femoral artery and vein were tightly ligated to secure the catheters. All thirteen pigs were successfully and accurately implanted with catheters in the carotid artery, femoral artery and vein.
Postoperative management
Postoperatively, pigs were placed in individual metabolism cages for recovery, and received daily intramuscular injection of penicillin sodium (6000 IU/kg BW) and streptomycin sulfate (20 mg/kg BW) for 4 d. On each day, the catheters were checked for patency, flushed and filled with heparinised saline solution. 10 ml blood was drawn from femoral vein immediately before, and at days 2 and 5 after the surgery, and plasma was obtained after centrifugation at 4°C at 3000 g for 10 min for C-reactive protein (CRP) analysis. There was no significant weight loss during the 4-d recovery phase (mean weight change of 0·59 (sd 1·08) kg). At day 5 post surgery, twelve of the thirteen test pigs were in good health with well-kept catheters, with six pigs for each group. All these pigs achieved presurgery feed intake (1·01 (sd 0·3) kg/d), and the patency rates of the carotid arterial, femoral arterial and venous catheters reached 100 %. The catheters were subsequently used for infusion of PAH and blood collection.
Infusion protocol and sample collection
On day 5 post surgery, pigs were deprived of feed from 18.00 to 07.00 hours. At 06.55 hours, a continuous infusion of 1 % (w/v) PAH (sodium salt, diluted in sterile saline at pH 7·5) was performed through the femoral artery catheter at a rate of 0·788 ml/min for 9 h after an initial bolus of 19·1 ml PAH solution( Reference Fang, Luo and Qi 25 ). At 07.45 hours, baseline arterial and venous blood samples (10 ml) were withdrawn into heparinised tubes. At 08.00 hours, pigs were offered with their corresponding diets at hourly intervals, and the diet was equivalent to 1/24 of the daily intake (45 g/kg BW), which was continued for 8 h to achieve a constant fed state( Reference Fang, Luo and Qi 25 , Reference Riedijk, Stoll and Chacko 28 ). From 09.00 to 16.00 hours, arterial and venous blood samples (10 ml) were simultaneously withdrawn every 1 h, and immediately placed on ice. Plasma samples were obtained after centrifugation, and stored at –80°C for the analysis of the concentrations of PAH, AA and BCAA transamination products, including KIC, α-keto-β-methylvaleric acid (KMV) and α-ketoisovaleric acid (KIV) for Leu, Ile and Val, respectively.
Immediately after the completion of blood sample collection, pigs were killed with a venous injection of sodium pentobarbital (50 mg/kg BW) and sodium phenytoin (5 mg/kg BW). The biceps femoris muscle samples were rapidly removed and frozen in liquid nitrogen to be stored at –80°C for subsequent AA and 3-methylhistidine (3-MH) analysis.
Sample analysis
Venous concentrations of CRP were analysed by a Porcine CRP ELISA Kit (Tridelta Development Limited). Concentrations of PAH in plasma samples were detected spectrophotometrically( Reference Fang, Luo and Qi 25 ) on an automated analysis system (Infinite M200 Pro; TEKAN). Free AA concentrations in plasma and muscle samples as well as the amount of free 3-MH in muscle samples, which was commonly used as an index of myofibrillar proteolysis, were determined by HPLC-quadrupole-orbitrap high resolution MS method using AQC derivatisation as described by Yin et al. ( Reference Yin, Li and Li 29 ). Plasma BCKA was o-phenylenediamine derivatised, ethyl acetate extracted, and quantified by LC-MS as previously described( Reference Olson, Chen and Lynch 30 ).
Metabolomic analysis using HPLC-quadrupole time-of-flight-MS
Metabonomic profiling was performed by HPLC-QTOF-MS as previously described( Reference Lin, Liu and Feng 31 ) with some modifications. In brief, plasma samples were extracted with ice-cold acetonitrile–methanol solution (1:1, v/v) at a 1:4 sample: extract solution ratio. After being vortexed and extracted for 2 h at –20°C, the samples were centrifuged at 13 000 rpm for 10 min at 4°C. The supernatants were collected and evaporated to dryness in a vacuum concentrator. The residues were resuspended in 100 μl of methanol–water (1:1), vortexed and centrifuged again at 13 000 rpm for 10 min at 4°C. The supernatants were transferred to sampler vials for HPLC-QTOF-MS analysis in both positive and negative ionisation modes. The Masshunter Profinder software (version B.06.00; Agilent Technologies, Inc.) was employed for molecular feature extraction from the raw data. The data set was further processed with Mass Profiler Professional software (version 13.1.1; Agilent Technologies, Inc.) for multivariate analysis, such as principal component analysis (PCA). Differences in metabolic profiling between two groups were analysed by t test and metabolites that met a criterion of P<0·05 and a fold change >1·5 were selected to generate formulas and search against the METLIN database.
Calculations
Plasma flow across hindlimb muscle was estimated using the PAH indicator dilution technique( Reference Ten have, Bost and Suyk-Wierts 32 ), and was calculated using the following equation:

where MPF is the muscle plasma flow (litre/kg per h), C i the PAH concentration in infusion solution (g/l), IR the infusion rate (litre/h), PAH v and PAH a the concentrations (g/l) of PAH detected in the femoral vein and carotid artery, respectively, and BW the body weight (kg).
The net AA or BCKA fluxes across hindlimb muscle were calculated as follows:

where MNF is the muscle net flux (μmol/kg per h), MPF the muscle plasma flow (litre/kg per h), and C a and C v the carotid arterial and femoral venous plasma concentrations (μmol/l) of AA or BCKA, respectively. Therefore, a positive flux means uptake and a negative flux means production of AA or BCKA.
Statistical analysis
The effects of diet (control v. treatment) and time (baseline and eight time point intervals after feeding) on muscle plasma flow (MPF), muscle net fluxes (MNF) and plasma concentrations of CRP and different sums of AA were determined using two-way repeated-measures ANOVA. Post hoc testing was performed using the Bonferroni t test for multiple comparisons with baseline and control. Moreover, arterial AA concentrations and MNF of AA and BCKA in fed state were calculated by the mean values of samples collected between 4 and 8 h after feeding. Significant differences among dietary groups for these data and intramuscular AA and 3-MH concentrations were tested using Student’s t test. Effect of diet (control v. treatment), feeding state (fasted v. fed) and their interaction on MPF (mean values during 1–8 h for the fed state) was analysed using two-way ANOVA with a 2×2 factorial arrangement of treatments. Correlation analysis of MNF of total essential AA (EAA), NEAA and AA and concentrations of arterial and intramuscular BCAA, and net BCKA production in fed state was performed to determine whether a significant relationship existed between these variables. All analyses were performed using the SAS 8.0. Data were expressed as mean values with their standard errors. Differences were considered as significant at P≤0·05 and a trend when 0·05<P<0·10.
Results
C-reactive protein
Venous concentrations of CRP at day 2 after surgery in both groups were significantly increased when compared with baseline (day 0) (P<0·05), and fell back to the baseline value at day 5 after surgery (Fig. 1). However, no differences were observed between groups at each time point.
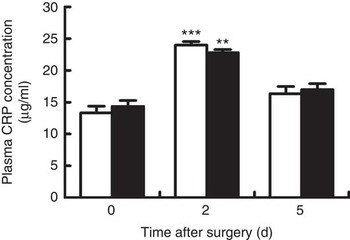
Fig. 1 Venous concentrations of C-reactive protein (CRP) in young pigs fed reduced-protein diets without (control, ) or with (treatment,
) supplemental branched-chain amino acids immediately before, and at days 2 and 5 after surgery. Values are means (n 6/group), with standard errors represented by vertical bars. ** P<0·01, ***P<0·001 v. baseline (day 0).
Muscle plasma flow
Pigs in both groups showed similar time course patterns of MPF after the start of feeding (Fig. 2(a)). MPF was rapidly and significantly increased within 1 h and continued to be elevated at 2 and 3 h in treatment group compared with baseline (P<0·05). There was a trend of increase in MPF at 3 h in control group relative to baseline (P=0·06). No group differences were observed at every time point. A further two-way ANOVA showed that MPF was higher in fed state than in fasted state (P<0·05; Fig. 2(b)), but there was no significant effect of dietary treatment or interaction between diet and feeding state on MPF.
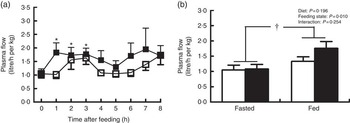
Fig. 2 Plasma flow across hindlimb muscle in young pigs fed reduced-protein diets without (control, ) or with (treatment,
) supplemental branched-chain amino acids at hourly intervals (a) and in fasted and fed state (b). Values are means (n 6/group), with standard errors represented by vertical bars. * P<0·05 v. baseline (t=0) based on repeated-measure ANOVA. † P<0·05 v. fasted state based on two-way ANOVA with a 2×2 factorial arrangement of treatments.
Amino acid concentrations in arterial plasma
Changes of AA concentrations in arterial plasma at different time points after the start of feeding are presented in Fig. 3. Compared with baseline, significant increases were observed for arterial concentrations of total BCAA at 1, 2, 7 h and total AA at 7 h in treatment group, and total EAA at 6 and 7 h in both groups (P<0·05). However, the arterial concentration of total NEAA did not differ at different time points in both groups. Furthermore, pigs in treatment group showed enhanced arterial concentration of total BCAA at 5, 7 and 8 h compared with the control (P<0·05), but no differences were detected regarding total EAA, NEAA and AA at any time points between two groups.
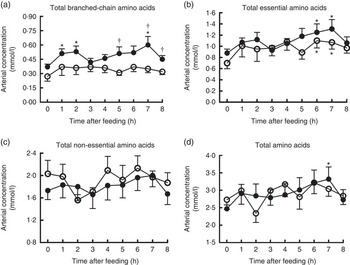
Fig. 3 Time course patterns of arterial concentrations of total branched-chain amino acids (BCAA) (a), essential amino acids (b), non-essential amino acids (c) and amino acids (d) in young pigs fed reduced-protein diets without (control, ) or with (treatment,
) supplemental BCAA. Values are means (n 6/group), with standard errors. * P<0·05 v. baseline (t=0). † P<0·05 v. control group at the corresponding time point.
The mean arterial concentrations of individual AA and total BCAA, EAA, NEAA and AA in fed state are presented in Table 2. There were no differences in arterial concentrations of total EAA, NEAA and AA between two groups, but total BCAA concentration was higher in treatment group than in control group (P<0·05). As expected, dietary BCAA supplementation enhanced the concentrations of individual BCAA (P<0·05). However, lower concentrations of phenylalanine and serine (P<0·05) and a trend (P=0·10) for lower cysteine concentration were detected after BCAA supplementation, even though their dietary contents were similar between two groups.
Table 2 Arterial concentrations (mmol/l) of individual and different sums of amino acids (AA) in young pigs fed reduced-protein diets without (control) or with (treatment) supplemental branched-chain AA (BCAA) in fed stateFootnote * (Mean values with their pooled standard errors; n 6/group)
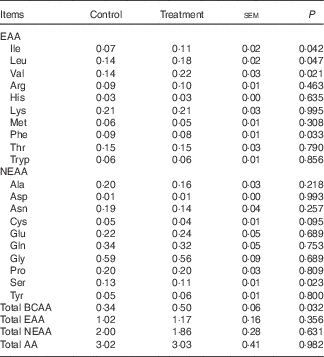
EAA, essential AA; NEAA, non-essential AA.
* Results are based on samples collected between 4 and 8 h after the start of the feeding protocol. Differences were considered significant at P<0·05.
Free amino acid and 3-methylhistidine concentrations in muscle
In line with elevated arterial concentrations, the concentrations of free individual and total BCAA in biceps femoris muscle were also increased by BCAA supplementation (P<0·05; Fig. 4(a)), whereas the concentration of serine was unexpectedly decreased (P<0·05; Fig. 4(b)). There was a trend for the decrease of histidine (P=0·08) and asparagine (P=0·06) concentrations in treatment group. Intramuscular concentrations of other individual AA and total EAA, NEAA and AA did not differ between two groups (Fig. 4(c)). Interestingly, intramuscular concentration of 3-MH, which is commonly used as an index of myofibrillar proteolysis, was lower in treatment group than in control group (P<0·05; Fig. 4(d)).
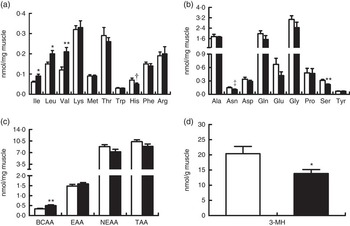
Fig. 4 Concentrations of free amino acids and 3-methylhistidine (3-MH) in biceps femoris muscle at the end of an 8-h feeding period in young pigs fed reduced-protein diets without (control, ) or with (treatment,
) supplemental branched-chain amino acids (BCAA). Individual essential amino acids (EAA) (a), individual non-essential amino acids (NEAA) (b), different sums of amino acids (c) and 3-MH (d) are shown. Values are means (n 6/group), with standard errors represented by vertical bars. * P<0·05, ** P<0·01, † P=0·08, ‡ P=0·06 v. control group. TAA, total AA.
Muscle net fluxes of amino acid and branched-chain amino acids transamination products
The time course patterns of the MNF of total BCAA, EAA, NEAA and AA after the start of feeding were similar in both groups (Fig. 5). Compared with baseline, treatment group showed elevated MNF of total BCAA, EAA and NEAA at 6 and 7 h as well as total AA within 4–7 h (P<0·05). With regard to control group, MNF were higher only for total BCAA at 7 h, but lower for total BCAA and EAA at 2 h compared with baseline (P<0·05). Moreover, MNF of total BCAA, EAA, NEAA and AA were detected to be higher at 7 h in treatment group than in control group (P<0·05).
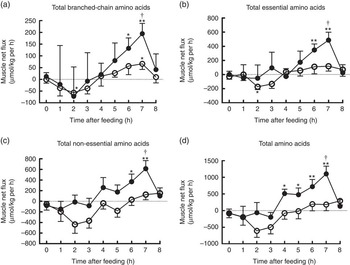
Fig. 5 Time course patterns of net fluxes of total branched-chain amino acids (BCAA) (a), essential amino acids (b), non-essential amino acids (c) and amino acids (d) across hindlimb muscle in young pigs fed reduced-protein diets without (control, ) or with (treatment,
) supplemental BCAA. Values are means (n 6/group), with standard errors. * P<0·05 v. baseline (t=0). ** P<0·01 v. baseline (t=0), † P<0·05 v. control group at corresponding time point.
The mean MNF of AA and BCAA transamination products in fed state are shown in Table 3. The treatment group showed higher positive net fluxes of total EAA, NEAA and AA as well as BCAA and their metabolites including alanine, glutamate and glutamine than control group (P<0·05). Meanwhile, BCAA supplementation also increased the MNF of histidine, Met and several functional NEAA including glycine, proline and serine (P<0·05), whereas no differences were observed between two groups for other AA. Interestingly, in fed state, both groups showed net uptake of almost all AA, except for asparagine and glutamine; besides, they also showed net production of BCKA, especially KIC, indicating that BCAA were catabolised in skeletal muscle. The first step of BCAA catabolism is transamination, which leads to the formation of KIC, KMV and KIV for Leu, Ile and Val, respectively. In accordance with the increased muscle net AA fluxes, net productions of KIC and KMV were greater in treatment group than in control group (P<0·05; Table 3). However, there was no difference in net KIV production between two groups.
Table 3 Net fluxes (μmol/kg per h) of amino acids (AA) and branched-chain AA (BCAA) transamination products across hindlimb muscle of young pigs fed reduced-protein diets without (control) or with (treatment) supplemental BCAA in fed stateFootnote * (Mean values with their pooled standard errors; n 6/group)
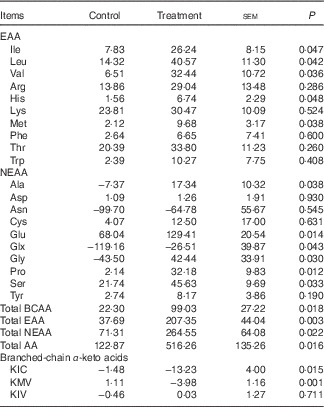
EAA, essential AA; NEAA, non-essential AA; KIC, α-ketoisocaproic acid; KMV, α-keto-β-methylvaleric acid; KIV, α-ketoisovaleric acid.
* Results are based on samples collected between 4 and 8 h after the start of feeding. Differences were considered significant at P<0·05. A positive flux means uptake and a negative flux means production.
Metabolomic profiling
PCA was employed to identify the possible relationships within classes of data. Data were visualised by plotting PCA scores, where each point on the plot represents a single sample. PCA score plot showed an excellent separation of plasma samples between the control and treatment groups (date not shown). Metabolomes of the plasma from two groups were differentially expressed, and most of these compounds were AA and their metabolites (Table 4). Compared with control group, pigs in treatment group displayed increases in BCAA and their transamination products BCKA, but decreases in Met, tryptophane, asparagine, glutamine, serine and proline (P<0·05). The trends of AA, except for those of BCAA, were consistent with the results of intramuscular AA concentrations, but were inconsistent with the results of muscle net AA fluxes. Moreover, a number of metabolites of lipid metabolism were more abundant in treatment group than in control group (P<0·05). Other metabolites, including purine, 6-hydroxyniacin, coenzyme Q9 and tributyrin, were also increased after BCAA supplementation (P<0·05).
Table 4 Metabolites that differed in femoral vein plasma between young pigs fed branched-chain amino acids (BCAA)-supplemented treatment diet and those unsupplemented control diet
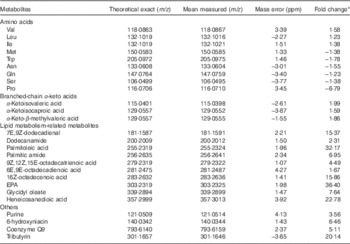
ppm, Parts per million.
* Fold change, which was based on the normalised data, was defined as the fold difference in the observed concentrations between control and treatment groups. A positive number for a fold change indicates that the value for treatment group was greater than control group, whereas the opposite is indicated by a negative number.
Correlations
The r correlation values are outlined in Table 5. There were significant positive correlations between the MNF of total EAA, NEAA and AA and the arterial concentrations of Leu and Ile (P<0·05). Moreover, the net fluxes of total EAA and AA were significantly positively correlated with the arterial concentration of Val (P<0·05). However, no significant relationships were found between the MNF of total EAA, NEAA and AA and the intramuscular concentrations of individual BCAA. As BCKA may potentially stimulate muscle protein synthesis, the correlations between the net fluxes of total EAA, NEAA and AA and the net productions of BCKA in muscle were also calculated. There were also significant positive relationships between the MNF of total EAA, NEAA and AA and the net production of KIC and KMV (P<0·05). These correlations indicated that muscle net AA fluxes are highly and positively correlated with arterial BCAA concentrations and BCAA catabolism in the muscle.
Table 5 Correlations between muscle net fluxes of different sums of amino acids (AA) and concentrations of arterial and intramuscular branched-chain AA (BCAA), and net branched-chain α-keto acids (BCKA) productions of young pigs in fed stateFootnote ‡
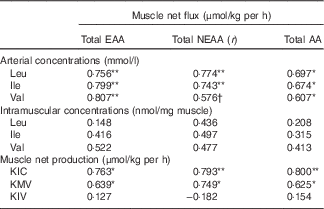
EAA, essential AA; NEAA, non-essential AA; KIC, α-ketoisocaproic acid; KMV, α-keto-β-methylvaleric acid; KIV, α-ketoisovaleric acid.
Significant correlation: * P<0·05, ** P<0·01. Trend towards statistical significance, † P=0·06.
‡ Results are based on samples collected between 4 and 8 h after the start of the feeding protocol (n 12).
Discussion
To our knowledge, this is the first study to investigate the roles of BCAA in regulating net AA fluxes across skeletal muscle and the related mechanisms. In this study, supplementation of BCAA to reduced-protein diet increased the MNF of total EAA, NEAA and AA (Table 3), which would facilitate the increase of muscle growth. Furthermore, the MNF of BCAA and their metabolites (including alanine, glutamate and glutamine) as well as histidine, Met and several functional NEAA (including glycine, proline and serine) were also increased after BCAA supplementation. Metabolomic profiling revealed a BCAA-related metabolite signature that was suggestive of increased intramuscular catabolism of BCAA induced by BCAA supplementation (Table 4). BCAA catabolism promotes the synthesis of BCKA and several NEAA, especially glutamine, both of which may contribute to the increase of muscle net AA fluxes.
Technical advantages
Skeletal muscle is the main site that synthesises protein with AA. The anabolic effect of BCAA on the growth of muscles including hindlimb biceps femoris muscle in pigs had been clarified in our recent previous study( Reference Zheng, Wei and Cheng 6 ). Therefore, the major goal of the present study was to determine the MNF of AA, and whether they are responsive to BCAA supplementation. To date, muscle net AA fluxes have been successfully detected by implanting catheters into abdominal aorta and inferior vena cava (inferior to the renal veins and superior to the common iliac vein) in several studies( Reference Deutz, Bruins and Soeters 10 , Reference Thivierge, Bush and Suryawan 11 ). However, this hindquarter flux model has several shortcomings, including difficulty of implanting catheters, a high risk of surgical-wound infection, and the fact that venous AA output from both hindquarter and several pelvic organs is also included in the estimation. Alternatively, hindlimb flux model by femoral artery and vein catheterization is more easily operated with a low risk of wound infection( Reference Ettrup, Glud and Orlowski 27 ). In this study, the surgical procedures for placing chronic catheters into one carotid artery, femoral artery and vein of pigs took approximately 1·5 h/animal, which is less than that required for hindquarter flux model.
CRP is often used as an indicator of inflammatory status after surgery( Reference Nason, Barry and Obinwa 33 ). In the present study, the plasma concentrations of CRP and feed intake in both groups were restored to basal levels at day 5 after surgery, indicating that the pigs did not suffer from inflammation and were in good health. Moreover, CRP concentration was not affected by BCAA supplementation. Thus, based on the samples collected at day 5 after surgery, any observed differences should be attributed to BCAA administration rather than to the changes in inflammatory state.
Muscle net amino acid fluxes
Few reports are available on the measurement of muscle net AA fluxes using hindlimb flux model in pigs. The results obtained using this model in the present study showed that pigs had positive MNF of total BCAA, EAA, NEAA and AA, and almost all individual AA except for glutamine and asparagine in both groups in fed state (Table 3), which supports the finding that feeding can induce the stimulation of protein synthesis in skeletal muscle( Reference Davis, Burrin and Fiorotto 34 , Reference Davis, Fiorotto and Burrin 35 ). Release of glutamine and asparagine from muscle even in fed state was also found in previous studies, which has important functions in extramuscular tissues( Reference Holeček 14 , Reference Wu 36 ).
It is noteworthy that the MNF of total EAA, NEAA and AA in pigs fed diet supplemented with BCAA increased significantly (Table 3), which would contribute to the elevation of muscle growth in pigs( Reference Zheng, Wei and Cheng 6 ). Moreover, dietary BCAA supplementation increased the MNF of BCAA and their metabolites including alanine, glutamate and glutamine. In addition to satisfying the requirement of protein synthesis, BCAA are also extensively utilised by muscle to produce alanine, glutamate, glutamine, aspartate and asparagine( Reference Holeček 14 , Reference Li, Knabe and Kim 15 ). This is supported by our results that BCAA accounted for 50–60 % of the EAA extracted by muscle, whereas they only accounted for 38 % of EAA in porcine muscle proteins( Reference Pond and Mersmann 37 ). Moreover, the synthesis of alanine, glutamate and glutamine from BCAA catabolism may explain the finding that the intramuscular concentrations of these three NEAA are not affected by BCAA supplementation despite of the increase of their net fluxes (Table 3). Although skeletal muscle catabolises BCAA also for the synthesis of aspartate and asparagine( Reference Harper, Miller and Block 13 , Reference Li, Knabe and Kim 15 ), no differences in net fluxes of these two AA were observed between groups in this study, which was probably due to the large pooled standard error of the mean (Table 3). As 97 % of glutamate, 95 % of aspartate and 67–70 % of glutamine in the diet are catabolised by pig small intestine in the first pass( Reference Stoll and Burrin 16 , Reference Wu, Bazer and Burghardt 17 ), it is likely that the extensive catabolism of BCAA in muscle may promote the resynthesis of these three AA to modulate intramuscular AA balance, and thereby increases muscle net AA fluxes.
Glutamate and ammonia are combined by glutamine synthetase to produce glutamine, which is required for maintaining an extremely high intramuscular concentration of glutamine( Reference Mittendorfer, Volpi and Wolfe 38 ). This high intramuscular glutamine level plays an important role in promoting protein synthesis and preventing protein degradation in the muscle( Reference Holeček 14 , Reference Wu and Thompson 39 ). It has been demonstrated that the increased production of glutamine from BCAA catabolism and protein mobilisation and the export of glutamine by muscle occur in a variety of atrophying conditions( Reference Lecker, Jagoe and Gilbert 40 ). Glutamine is simultaneously extracted by liver, gut and lymphocytes, which is of physiological importance( Reference Karinch, Pan and Lin 41 ). Interestingly, in this study, the release of glutamine from skeletal muscle was reduced after BCAA supplementation (Table 3), which might be required for the sustaining elevation of protein synthesis and reduction of protein degradation that lead to the elevation of protein deposition in skeletal muscle. The decrease of muscle protein degradation after BCAA supplementation was demonstrated by the reduction in intramuscular concentration of 3-MH (Fig. 4(d)). These results indicate that dietary supplementation of BCAA would be an effective way to improve muscle protein balance through increasing glutamine synthesis from BCAA and thus inhibiting protein mobilisation.
In addition, as the catabolism of histidine, glycine, proline and serine in muscle is negligible( Reference Goldberg and Odessey 42 , Reference Rennie and Tipton 43 ), the increased extraction of these four AA by muscle may be totally used for protein synthesis, which contributes to the increase of protein synthesis after BCAA supplementation. Although Met can be transaminated and is also subjected to transulfuration in muscle, the pathway is quantitatively unimportant( Reference Wu and Thompson 44 , Reference Wu and Thompson 45 ).
Modulation of muscle net amino acid fluxes by substrate concentrations
BCAA, particularly Val and Ile, are found to be limiting AA for growth following Lys, Met, Thr and Trp, and deficiency of dietary BCAA impairs the growth of young pigs( Reference Lordelo, Gaspar and Le Bellego 46 , Reference Soumeh, van Milgen and Sloth 47 ). As BCAA are EAA that cannot be synthesised de novo in animal cells( Reference Wu, Bazer and Dai 48 ), they must be obtained from the diet. Even though 30–50 % of dietary BCAA are degraded by pig small intestine in the first pass( Reference Stoll and Burrin 16 , Reference Wu, Bazer and Burghardt 17 ), it seems that the amounts of these AA that enter blood circulation are positively correlated with their amounts in the diets( Reference Soumeh, van Milgen and Sloth 47 , Reference Murgas Torrazza, Suryawan and Gazzaneo 49 – Reference Yin, Yao and Liu 51 ). In the present study, dietary BCAA supplementation, as expected, significantly increased both the arterial and intramuscular concentrations of individual BCAA in pigs (Table 2 and Fig. 4), which may provide sufficient substrates for protein synthesis in the muscle. It has been shown that the increase of blood AA concentrations occurs faster than can be accommodated by a stimulation of membrane transport( Reference Taylor, Rennie and Low 52 ), and when the demand for AA in muscle protein synthesis outstrips AA supply, intramuscular AA concentrations would decrease, even though the influx to the intracellular compartment increases( Reference Bohé, Low and Wolfe 53 ). These findings may explain our observation that the muscle net AA fluxes were strongly and positively correlated with arterial BCAA concentrations rather than with intramuscular BCAA concentrations (Table 5). For other AA, as their dietary contents were similar between two groups, the arterial concentrations of these AA (except for phenylalanine, serine and cysteine) were not affected by BCAA supplementation. A decrease in plasma concentration of serine in pigs was also detected after Leu supplementation( Reference Soumeh, van Milgen and Sloth 47 , Reference Yin, Yao and Liu 51 ). As phenylalanine, serine and cysteine can be utilised by portal-drained viscera and liver, the decreases in arterial levels are likely due to the increased utilisation in these two tissues. However, the reason and significance of this increase in catabolism following BCAA supplementation remain to be further investigated.
Modulation of muscle net amino acid fluxes by branched-chain amino acids as the direct signaling molecules
The feeding-induced stimulation of muscle protein synthesis in pigs is independently regulated by the increase of insulin and AA( Reference O’Connor, Bush and Suryawan 54 ). Our previous study has shown that the level of plasma insulin is not affected by BCAA supplementation( Reference Zheng, Wei and He 55 ), which is consistent with results of other studies obtained by the infusion of individual BCAA or mixed EAA( Reference Escobar, Frank and Suryawan 4 , Reference Volpi, Kobayashi and Sheffield-Moore 56 ). Thus, it seems that the anabolic effect of BCAA is unlikely mediated by insulin. Apart from building blocks for protein synthesis, BCAA, especially Leu, also serve as nutrient signals to promote muscle protein synthesis by stimulating translation initiation in pigs( Reference Murgas Torrazza, Suryawan and Gazzaneo 49 – Reference Yin, Yao and Liu 51 ). Our recent study showed that the increase in muscle mass of young pigs after supplementation of BCAA is associated with the activation of translation initiation factors, including mTOR and S6K1( Reference Zheng, Wei and Cheng 6 ). These results indicate that the supplementation of BCAA increases their intramuscular concentrations, and thus stimulates translation initiation, leading to an elevation of muscle net AA fluxes.
Modulation of muscle net amino acid fluxes by catabolism of branched-chain amino acids
BCAA not only serve as the substrate for protein synthesis but also are catabolised extensively in skeletal muscle. As described above, BCAA catabolism in muscle promotes the synthesis of glutamine, glutamate and aspartate, which are extensively catabolised by pig small intestine, and thus may reduce protein mobilisation and increase protein synthesis. Correspondingly, in this study, the metabolomic profiling of femoral vein of the control and BCAA-supplemented pigs revealed a BCAA-related metabolite signature that was suggestive of increased catabolism of BCAA (Table 4), which was associated with the increased muscle net AA fluxes. Two major classes of metabolites, BCKA and lipid metabolism-related products, were identified to be different between the control and BCAA-supplemented pigs. Similarly, the alteration of lipid metabolism induced by BCAA supplementation was previously reported for rats fed high-fat diet( Reference Newgard, An and Bain 57 ). Interestingly, the ability of Leu to act as a nutrient signal to stimulate muscle protein synthesis is specific for Leu and/or its transamination metabolite KIC. KIC is potentially used to promote muscle protein synthesis through stimulating translation initiation( Reference Columbus, Fiorotto and Davis 5 , Reference Escobar, Frank and Suryawan 18 ). Increased muscle net KIC and KMV production was observed after BCAA supplementation in the present study, which was strongly and positively correlated with muscle net AA fluxes (Table 5). Furthermore, infusion of another Leu metabolite β-hydroxy-β-methylbutyrate to piglets resulted in the activation of translation initiation and an increase in muscle protein synthesis( Reference Wheatley, El-Kadi and Suryawan 58 ). These results indicate that the elevation of BCAA catabolism may contribute to the increase of net AA fluxes in muscle after BCAA supplementation. Further studies are needed to determine the direct roles of these metabolites of BCAA in regulating muscle net AA fluxes and protein synthesis.
In summary, supplementation of BCAA to reduced-protein diet increases the substrate concentrations of BCAA in both arterial plasma and muscle, and enhances the intramuscular catabolism of BCAA, which promotes the synthesis of BCKA and several NEAA, particularly glutamine. The increased substrate concentrations and catabolism of BCAA may increase muscle net AA fluxes, and thereby lead to the elevation of muscle protein deposition in young pigs. These results provide a direct biochemical explanation for the anabolic effect of BCAA on muscle protein metabolism. Our findings also indicate that rather than to be a nutritional loss, the catabolism of BCAA in muscle is of enormous biological importance. Furthermore, our hindlimb flux model combined with metabolite profiling identified some BCAA-related metabolites (such as BCKA and EPA, etc.), which may potentially and partially mediate BCAA dependent-increase of muscle net AA flux and growth.
Acknowledgements
The authors are grateful to Associate Professor Zuoxiong Liu for English editing.
The work was jointly supported by the National Basic Research Program of China (973 Program) (no. 2013CB127305), and the Key Technology Research and Development Program of Hubei Province (nos 2014ABB014 and 2014ABC012).
The authors’ contributions are as follows: J. Peng, L. Z., H. W. and S. Z. designed the research; L. Z., F. Z., S. Z., P. H., Q. X. and J. Pang conducted the research; L. Z., F. Z. and P. H. analysed the data; L. Z., H. W. and J. Peng wrote the manuscript. J. Peng had primary responsibility for the final content. All authors read and approved the final manuscript.
None of the authors has any conflicts of interest to declare