Introduction
Early atmosphere and chemical evolution
The atmosphere of terrestrial planets could have developed through processes such as nebular gas capture, from the volatiles from impactors during the accretion or post-accretion stages, and volcanic outgassing (Kuwahara and Sugita, Reference Kuwahara and Sugita2015; Schaefer and Fegley, Reference Schaefer and Fegley2017). The atmospheric conditions of Earth in its first million years have not been determined due to the scarcity and limitations of geochemical and geological evidence (Marchi et al., Reference Marchi, Black, Elkins-Tanton and Bottke2016). However, Earth's atmosphere is described as a secondary atmosphere because the primary atmosphere was gravitationally captured gas from the solar nebula, which is composed mainly of hydrogen. This primordial atmosphere was lost and replaced by the secondary atmosphere, probably formed from volatiles delivered to Earth by asteroids, comets and volcanic emissions. The primitive atmosphere was probably dominated by CO2, N2 and H2O (Zahnle et al., Reference Zahnle, Schaefer and Fegley2010; Kasting, Reference Kasting and Kolb2018), and would have been a weakly reducing atmosphere.
Although models of the physiochemical characteristics of the atmospheric and terrestrial surface of the Hadean and early Archean eons are still debated, some evidence, such as the geochemistry of the oldest zircons (dated between 4.3–4.4 Ga), suggests that rocks were altered by liquid water at this time (Mojzsis et al., Reference Mojzsis, Harrison and Pidgeon2001; Zahnle, Reference Zahnle2006). Analysis shows that they formed in fayalite-magnetite-quartz environments similar to the current mantle oxidation state, suggesting the state of the mantle has not changed significantly; however, the composition and redox state of a planet mantle are related to the chemistry of its atmosphere (Trail et al., Reference Trail, Watson and Tailby2011; Itcovitz et al., Reference Itcovitz, Rae, Citron, Stewart, Sinclair, Rimmer and Shorttle2022). Despite the luminosity of the early Sun being lower than today (20–30%) (Sagan and Mullen, Reference Sagan and Mullen1972; Kasting et al., Reference Kasting, Pollack and Crisp1984), zircons require liquid water to form; the hydrosphere existed on Earth by 4.3 Ga ago (Mojzsis et al., Reference Mojzsis, Harrison and Pidgeon2001). The greenhouse effect is necessary for liquid water to exist, even if the possible sources of gases such as CH4 are difficult to explain. Elevated amounts of CO2 and CH4 are also necessary to explain the Young Sun paradox (pCH4 = 0.01–10 mbar) (Charnay et al., Reference Charnay, Wolf, Marty and Forget2020).
Understanding the atmospheric composition of the early Earth is essential for the studies related to prebiotic chemistry and the origin of life since the generation of the organic compounds relevant to living beings is easily achieved under reduced conditions, as Oparin (Reference Oparin1924) and Urey (Reference Urey1952) proposed and as was observed in Miller's work (Miller, Reference Miller1953).
Some mechanisms that contribute to the presence of CH4 and H2 in the atmosphere have been proposed: serpentinization at hydrothermal vents on the sea floor (Reeves and Fiebig, Reference Reeves and Fiebig2020), and chondritic material, given that asteroid impacts would have contributed to the formation of the secondary atmosphere due to the degassing of volatile compounds from the incoming asteroidal or cometary material. Theoretical studies have shown that primitive atmospheres would contain significant amounts of reducing species (CH4, H2) if they originate from chondritic material (Hashimoto et al., Reference Hashimoto, Abe and Sugita2007; Schaefer and Fegley, Reference Schaefer and Fegley2007, Reference Schaefer and Fegley2010); and the reducing potential of large impacts (Kuwahara and Sugita, Reference Kuwahara and Sugita2015; Benner et al., Reference Benner, Bell, Biondi, Brasser, Carell, Kim, Mojzsis, Omran, Pasek and Trail2020; Zahnle et al., Reference Zahnle, Lupu, Catling and Wogan2020; Itcovitz et al., Reference Itcovitz, Rae, Citron, Stewart, Sinclair, Rimmer and Shorttle2022). In the latter case, many of the impactors are in a much more reduced state than the mantle. For example, many meteorites (including ordinary chondrites and enstatites) contain substantial amounts of metallic iron and iron sulphides, so the gases balanced with these materials would be highly reducing (Zahnle et al., Reference Zahnle, Lupu, Catling and Wogan2020). Zahnle's et al.'s work (Reference Zahnle, Lupu, Catling and Wogan2020) supposes a greater contribution to the generation of CH4 by considering >100 km diameter projectiles that could have collided with Earth during the late accretion. Large impacts would have contributed to obtaining a transient reduced environment due to the production of H2 through the reduction of H2O by the metallic iron from the impactor (Genda et al., Reference Genda, Brasser and Mojzsis2017; Benner et al., Reference Benner, Bell, Biondi, Brasser, Carell, Kim, Mojzsis, Omran, Pasek and Trail2020; Zahnle et al., Reference Zahnle, Lupu, Catling and Wogan2020; Itcovitz et al., Reference Itcovitz, Rae, Citron, Stewart, Sinclair, Rimmer and Shorttle2022). After cooling, the atmosphere would have reduced conditions from the presence of CH4 and NH3. From these kinds of reduced molecules, it is possible to obtain HCN, an essential precursor of other molecular species relevant for prebiotic chemistry. Itcovitz et al. (Reference Itcovitz, Rae, Citron, Stewart, Sinclair, Rimmer and Shorttle2022) have found in their model that as the mass of the impactor increases, the production of H2 also increases, and H2 would be more abundant than CO2, and the production of CO is expected. They also found that small amounts of CH4 can be produced in the most reducing atmospheres, and the amount N2 does not change significantly with impactor mass. Considering the probable age of the formation of the Moon and the record of impact craters on its surface, it has been concluded that there was a high rate of impacts of asteroidal and cometary material between 4.5 and 3.5 Ga, which suggests that similar impacts also occurred on the surface of the Earth (Marchi et al., Reference Marchi, Black, Elkins-Tanton and Bottke2016; Zellner, Reference Zellner2017). The lunar crater record also shows that the flux and size of the impactors decreased drastically after this period, the end of which seems to coincide with the origin of life (Farmer, Reference Farmer2000; Hartman et al., Reference Hartman, Ryder, Done, Grinspoon, Canup and Righter2000).
The studies focused on proposing pathways of prebiotic chemical reactions for the subsequent origin of life mostly involve scenarios on continental or oceanic crust. Some authors have considered the energy contribution of the shock waves produced by the passage of asteroids through the atmosphere to model or simulate the production of abiotic organic compounds. Bar-Nun et al. (Reference Bar-Nun, Bar-Nun, Bauer and Sagan1970) have produced chemical reactions in a mixture of gases (CH4, C2H6, NH3 and Ar) simulating primordial conditions and inducing shock waves. Their results show that amino acids such as glycine and alanine can be obtained. McKay and Borucki (Reference McKay and Borucki1997) have used a laser to simulate the interaction of shock waves with an atmosphere composed of CH4, H2O, CO2, N2 and H2S and one composed of H2 and CO2. They detected hydrogen cyanide (HCN), formaldehyde (HCHO), ethane (C2H6) and methylamine in the first simulated atmosphere and water and CO in the second. Shock waves in the Martian atmosphere have also been proposed as a mechanism for nitrogen fixation in the early stage of this planet (Navarro-González et al., Reference Navarro-González, Navarro, Coll, McKay, Stern, Sutter, Archer, Buch, Cabane, Conrad, Eigenbrode, Franz, Freissinet, Glavin, Hogancamp, McAdam, Malespin, Martín-Torres, Ming and Vasavada2019).
In this work, we propose different models of primitive atmospheres, with and without a nitrogen source, and observe the possible role of shock waves from asteroid impacts on the atmosphere in producing relevant organic compounds for the chemical evolution on Earth.
Methodology
Theoretical calculations of impact energy
The energy of the shock waves produced by the passage of asteroids through the atmosphere of Earth could have produced compounds of astrobiological interest. We assessed the energy this kind of shock wave produced to determine how much energy could have been released and how many compounds could have been produced. Due to plate tectonics and weathering, the record of impact craters on the surface of the Earth has been obliterated, so the number and size of past impacts are unknown. However, the surface of the Moon can be used to estimate these parameters.
Possible early life indicators are presumed to be found in seafloor precipitates of hydrothermal vents and could be as old as 3.7 Ga (Dodd et al., Reference Dodd, Papineau, Grenne, Slack, Rittner, Pirajno, O'Neil and Little2017). Also, some structures can provide data on the dates of the presence of pristine life: stromatolites, which are defined by Schopf (Reference Schopf2006) as ‘accretionary sedimentary structures, commonly thinly layered, megascopic and calcareous, inferred to have been formed by mat-building communities of mucilage-secreting microorganisms, mainly photoautotrophic microbes’. Furthermore, sedimentary structures provide information about the environments where they were formed, that is, in shallow waters. The stromatolites discovered in the Isua greenstone belt have dated to ~3.7 Ga (Nutman et al., Reference Nutman, Bennett, Friend, Van Kranendonk and Chivas2016, Reference Nutman, Bennett, Friend, Van Kranendonk, Rothacker and Chivas2019). However, it has also been suggested that these structures are probably not of biological origin, so there is still a debate about these systems (Allwood et al., Reference Allwood, Rosing, Flannery, Hurowitz and Heirwegh2018). We are concerned about the chemical evolution period, i.e. the time before life became widespread. The period of interest in this study is before the first unambiguous forms of life (3.7 Ga). In this work, 3.8 Ga was the lower limit.
Figure 3 in Halliday (Reference Halliday2006) shows that the Moon was formed between 40 and 50 Ma after the solar system began to form. After the impact of Gaia and Theia, the surface of the Moon was a magma ocean that eventually hardened into the crust. Carlson et al. (Reference Carlson, Borg, Gaffney and Boyet2014) have performed isotopic analysis of several lunar rocks to assess the age of the crust. According to this analysis, the crustal rocks have formation ages between 4.32 and 4.50 Ga. The youngest age they reported was 4283 ± 23 Ma, although this could be due to the behaviour of the plagioclase, on which their results depend. On this basis, 4.2 Ga was the upper limit for assessing the number of impacts over the Moon and the Earth. This date range ensures that the period under consideration includes the presence of a lunar (and hence an impact record) and the time before life appeared on Earth.
Neukum et al. (Reference Neukum, Ivanov and Hartmann2001) have stated that the temporal dependence of the number of craters ≥1 km in diameter per km2 of surface area is represented by the equation:

where T is the crater accumulation time in Ga. Integrating equation (1) between 3.8 and 4.2 Ga and multiplying by the Moon's surface area (~37 962 900 km2 assuming an equatorial radius of 1738.1 km), around 1 233 834 impact craters ≥1 km were produced in this time interval.
On the other hand, Neukum et al. (Reference Neukum, Ivanov and Hartmann2001) have also represented the size-frequency distribution of lunar craters (their equation (2)), i.e. the number of craters with a diameter greater than D formed per km2 during 1 Ga as a polynomial of degree 11. This equation is valid for craters with diameters between 0.01 and 300 km. This polynomial keeps its shape for every interval of time. To consider the constraints of equation (1) and those of this polynomial, only craters whose diameters were between 1 and 300 km were considered. In this context, the shape of the polynomial and the result from the previous paragraph were used to calculate the number of craters of different sizes formed on the Moon between 4.2 and 3.8 Ga.
To do this, we normalize the number of impact craters with a diameter ≥1 km and multiply the result by 1 233 834. Then, to have a non-accumulated distribution function, we subtract the value of one interval from the previous one. Our results are in Table 1. There, the diameter, D, in the first column, is equal to $\sqrt {D_LD_R}$, where DL is the lower limit, and DR is the upper limit of the interval, as in Neukum et al. (Reference Neukum, Ivanov and Hartmann2001). In this study, we started with a diameter of 0.01 km, but in Table 1, we consider only the interval between 905 m and 327 km.
Table 1. Number of impact craters that must have been produced on the Moon and the Earth between 4.2 and 3.8 Ga

The third column shows the range of impact crater populations that could have been formed on the Earth if the impact flux was between 13.5 and 33.3 times the impact flux on the Moon
According to Canup and Asphaug (Reference Canup and Asphaug2001), the ratio of impact rate of the Moon to that of the Earth is

where R E is the radius of the Earth, R M is the radius of the Moon, and f M and f E are the gravitational focusing factors of the Moon and Earth, respectively. N 1 can have values between 0.03 and 0.074; that is, in the same time interval, between 13.5 and 33.3 times more impact craters could have been produced on the Earth than on the Moon (Table 1).
According to Table 1, between 4.2 and 3.8 Ga, at least 546 cosmic objects with diameters D, such that 115 km < D < 328 km, fell on Earth. The impacts of such objects could have contributed to generating CH4.
Based on Table 1, the energy released in the atmosphere due to the entry of cosmic bodies can be assessed. Globally, 95.1% of falls are stony meteorites, of which 93.1% are chondrites, of which 93.9% are ordinary chondrites (Korotev, Reference Korotev2021). This means that 83.1% of the falls are ordinary chondrites. In first approximation, impactors before 3.8 Ga are compositionally similar to present-day impactors; thus, the most common material that has impacted the Earth is ordinary chondrite, whose mean density is 3.54 g cm−3 (Britt and Consolmagno, Reference Britt and Consolmagno2003). The mean impact velocity of asteroids on our planet is 20.3 km s−1 (Brown et al., Reference Brown, Spalding, ReVelle, Tagliaferri and Worden2002). Therefore, the energy of each object in Table 1 was calculated using the equation for the kinetic energy and assuming that the objects are spheres. The results obtained are shown in the third column of Tables 2 and 3. The fourth column of these tables shows the total entry energies due to all the impactors of each size that fell into the atmosphere of the Earth between 4.2 and 3.8 Ga.
Table 2. Number of impact craters that could have formed on the crust of the Earth between 4.2 and 3.8 Ga and the putative energies that the entry of the impactors could have released to the atmosphere, assuming that the number of impacts on Earth was 13.5 times greater than the number of impacts on the Moon

Table 3. Number of impact craters that could have formed on the crust of the Earth between 4.2 and 3.8 Ga and the putative energies that the entry of the impactors could have released to the atmosphere, assuming that the number of impacts on Earth was 33.3 times greater than the number of impacts on the Moon

A portion of this energy is transformed into heat energy, which increases the temperature of the incoming body, melting and evaporating part of its material. As the body continues moving, only a part of its pre-entry kinetic energy is deposited in the atmosphere. The Earth Impact Effects Program is an interactive website that permits users to estimate several effects of the impact of a cosmic body on Earth (https://impact.ese.ic.ac.uk/ImpactEarth/ImpactEffects/). According to this program, an object with a diameter of 905 m, density of 3540 kg m−3, velocity of 20.3 km s−1 and an entry angle of 45° (the most probable angle) will lose ~1.2% of its entry energy in the atmosphere. This program does not give this information for more massive objects, possibly because the energy loss is lower. In this work, 1% of the entry energy is a reasonable upper limit for the energy deposited into the atmosphere due to the objects in Tables 2 and 3 (fifth column of both tables).
Considering the total energy released into the atmosphere by impactors >29 km (diameters ≥34.4 km in Table 2), they are responsible for 91.5% of the energy released into the atmosphere by all the impactors that hit the Earth in the time interval of interest. Considering that the larger the object, the less energy is deposited in the atmosphere, we could put an even greater restriction and consider only objects smaller than 29 km. In this case, the total energy deposited in the atmosphere (adding the first 10 rows from the fifth column of Tables 2 and 3) was between 2.1 × 1027 and 5.1 × 1027 J.
The work of Pérez-Rodríguez (Reference Pérez-Rodríguez2020) is similar to this section, as it is a compilation of various works that simulate the effect of a shock wave in atmospheres with different proposed compositions (Table 4).
Table 4. Studies of the production of organic molecules in the atmosphere. Their energy sources simulate those of shock waves

Taken from Pérez Rodríguez (Reference Pérez-Rodríguez2020).
1 α = CO2/(CO2 + N2)
Based on Table 4, and as an example, 1 J of energy produces 1016 molecules of NO in a neutral atmosphere, so if all the energy released by impactors that hit Earth between 4.2 and 3.8 Ga was used to produce this compound, then 2.46 × 1044–6.07 × 1044 molecules of NO, i.e. 1.2 × 1022–3.0 × 1022 g of NO, could have been formed during this period. If we consider our more restrictive values (2.1 × 1027 and 5.1 × 1027), the number of molecules formed was between 2.1 × 1043 and 5.1 × 1043, or equivalently between 1.0 × 1021 and 2.5 × 1021 g of NO. From these preliminary results, we decided to perform an experimental study of the simulated released energy by a shock wave in different models of primitive atmospheres.
Experimental procedure
The methodology was divided into three sections. The first set of experiments consisted of the laser irradiation of different gas mixtures (including CO, CO2, H2O and CH4) without a nitrogen source (details in Table 5) to evaluate the possibility of obtaining oxygenated compounds (e.g. alcohols, carboxylic acids) relevant to chemical evolution. The second set of experiments consisted of preparing CH4, CO2 and N2 atmospheres, varying the content of the last two gases, and keeping a constant 1 h irradiation time (see Table 6) to observe the differences among the products obtained in the first set of experiments. The third set was the analysis of a particular atmosphere from second set at different irradiation times to study the production yield of the relevant compounds. All experiments were performed in triplicate.
Table 5. Nitrogen-free atmospheres used in the first set of experiments

Table 6. Composition of the simulated atmospheres for the second set of experiments

Reactor filling system and simulated atmosphere irradiation
High-purity gases (CO2 = 99.99%, CH4 = 99.97%, N2 = 99.99%, CO = 99.99%) were used to simulate primitive atmospheres and were supplied by Praxair, Inc. The cylinders were connected to a two-step regulator and a filter. The gas flowed through a stainless-steel pipe to a mixing system, where the atmospheres to be simulated were prepared. The mixer used was a Linde FM-4660, equipped with an Omega DP-350 temperature gauge, a Combitron CM 351 vacuum gauge and an Omega DP-80 temperature gauge. It has eight electronic channels that control and measure gas mass flow through thermal conductivity (each channel supports a different gas). All the experiments were realized in the Plasma Chemistry and Planetary Studies Laboratory at the Nuclear Sciences Institute (ICN), UNAM. Figure 1 shows the experimental setting for preparing the gas mixture.
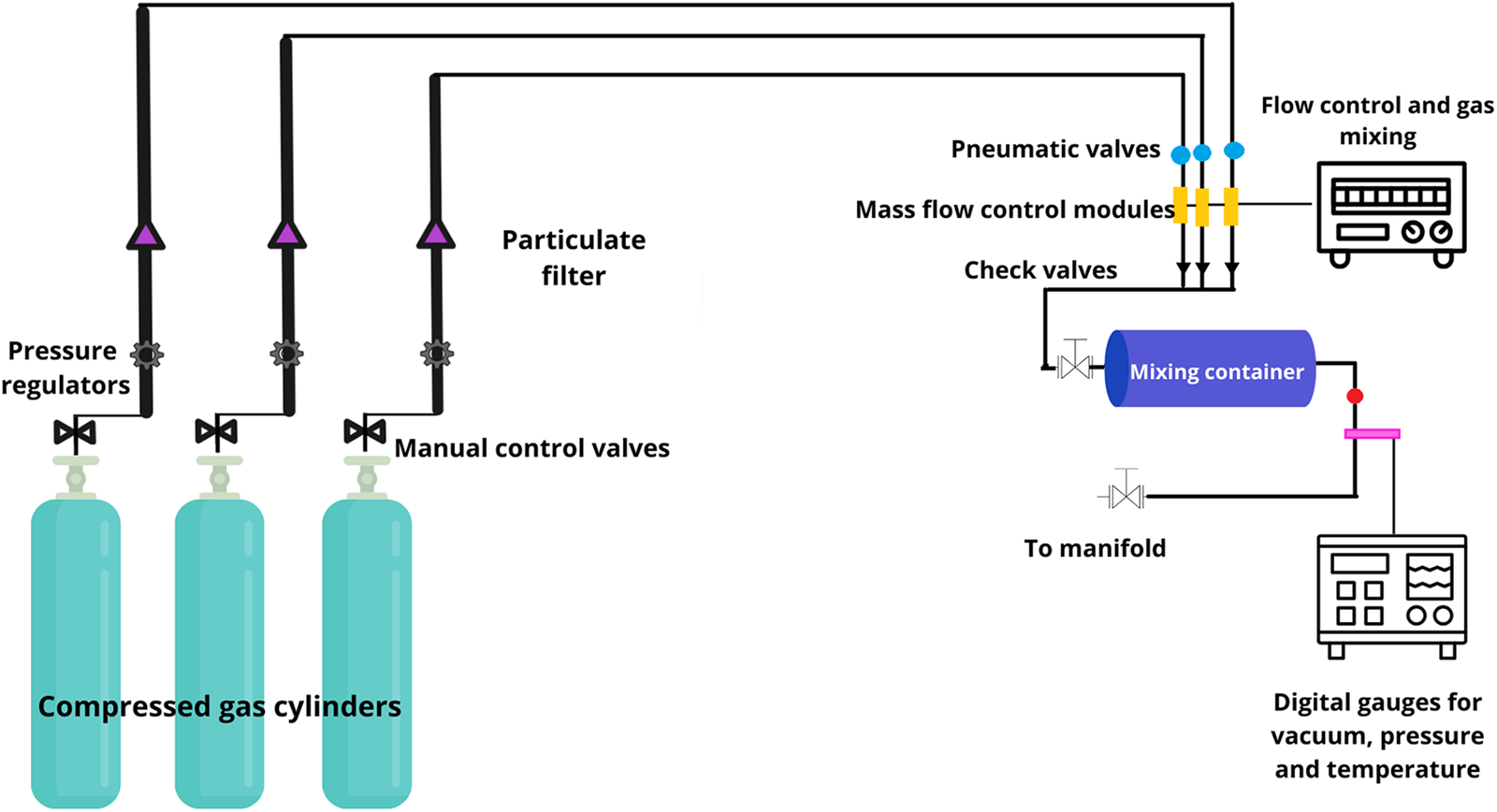
Figure 1. Experimental setting (adapted from Segura and Navarro-González, Reference Segura and Navarro-González2005).
The containers for the gaseous mixtures were spherical Pyrex glass flasks (1000 mL) with a high vacuum Teflon valve with 3 O-rings adapted; this tap allows the connection to fill the container. Once filled, the container was placed on the optical table for irradiation. The laser was a solid-state Nd-YAG with pulsed laser energy of 7 ns and a wavelength of 1.06 nm. The laser produces a short-duration plasma, as well as high temperatures and pressures, which can simulate the energetic process of a shock wave. The energy deposited into the system was determined by the difference between the input laser energy and the transmitted energy and was determined using a LabMaster model LM ULTIMA power and energy measurement system.
Analysis of samples
Gas products
An Agilent 7890a gas chromatograph with a six-port automatic injection system linked to a 2 mL pneumatic injection circuit was used for the chemical analysis and separation of the irradiation products. The temperature programme used for separating the compounds was initially operated at 50 °C for 5 min and continued at a temperature ramp of 10 °C per minute until the final temperature of 240 °C was reached. The carrier gas used was helium (grade ultra-high purity 99.999%), with a split ratio of 10:1 and a split flow of 120 mL min−1. The separation column was a fused silica capillary CP-PorabondQ of 50 m length, 0.32 mm internal diameter and a stationary phase thickness of 5 mm. After chromatographic separation, the products were analysed using an Agilent 5975C inert XL EL/CI MSD mass spectrometer with a quadrupole Triple-Axis detector. The electronic impact mode was used, with a spectral interval of 10–100 m/z and a resolution of 1 amu. The compounds were identified through SCAN mode or mass scanning and compared with the NIST 05 Mass spectral library, to detect the compounds that are generated after irradiation.
Solid product
The solid residue produced after the irradiation of some samples (details in section ‘Chemical analysis of the organic polymers’) was collected with methanol and later evaporated and analysed by different techniques:
Attenuated total reflectance-Gourier transform infrared ATR-FTIR spectroscopy
A Spectrum 100 Perkin Elmer® spectrophotometer was used. The sample was analysed using 20 scans. The interval between 400 and 4000 cm−1 was selected for the acquired spectra.
Raman spectroscopy analysis
An Optosky ATR 3000 portable Raman spectrometer was used with a Class IIIB laser positioned at an operating distance of 6 mm from the sample, and the laser was kept at a constant value of 400 mW. A NaCl window was used for the analysis. Raman and FTIR spectroscopy were performed at the Department of Radiation Chemistry and Radiochemistry at the ICN-UNAM.
Elemental analysis
A Perkin Elmer PE2400 Analyser was used for duplicated sample analysis of the percentile content of carbon, hydrogen and nitrogen. The carrier gas utilized was helium. The temperature of the chromatographic column was set at 82.2 °C, with a thermal conductivity detector. The combustion reactor was set at 975 °C and the reduction reactor at 501 °C. The analysis time was 430 s, and the calibration compound used was cystine. This analysis was conducted at the Research and Industry Support Services Unit (USAI) at the Faculty of Chemistry, UNAM.
Results
Atmospheres without a nitrogen source
All the atmospheres prepared were irradiated between 0.5 and 5 h. The gas composition of those mixtures is summarized in Table 7. Generally, the compounds obtained after irradiation were short-chain, saturated and unsaturated hydrocarbons (C2–6). No oxygenated compounds (i.e. alcohols, ethers, carboxylic acids, etc.) were found at the experimental conditions used in this study.
Table 7. Products obtained after the irradiation of the proposed primitive atmospheres.

CO2, CH4 and N2 atmospheres
Short-chain hydrocarbons were also produced in the nitrogen-containing atmospheres (Table 8); different molecules were produced as the CO2 content decreased. In the mixtures with a more significant amount of nitrogen (≥80% N2), the formation of organic polymers as yellowish solids deposited on the walls of the reactors was observed. Compounds containing two carbons were detected from the 60% N2, 10% CH4 and 30% CO2 atmosphere and appeared in each subsequent atmosphere. Compounds containing three carbons or more carbons were detected in the gas mixture corresponding to 80% N2, 10% CH4 and 10% CO2. Figure 2 shows a chromatogram from the 88% N2, 10% CH4 and 2% CO2 mixture after 1 h of irradiation.
Table 8. Simulated atmospheres for the second set of experiments and the products obtained after irradiation


Figure 2. Above: Chromatogram (scan mode) of the irradiation products of the 88% N2, 10% CH4 and 2% CO2 atmosphere, where HCN is separated from the other hydrocarbons produced (peaks: (1) ethylene; (2) ethane; (3) propene; (4) propane; (5) propyne; (6) hydrogen cyanide; (7) propyne; (8) 1-butene; (9) 1-buten-3-yne; (10) 1-butyne; (11) 1,3-butadiyne; (12) benzene). Below: CG-MS spectra of the analysed hydrogen cyanide.
Chemical analysis of the organic polymers
We collected the yellowish solid produced after irradiation and obtained the ATR-FT-IR and Raman spectra of the sample (Fig. 3). Both spectra show that compounds with the expected functional groups are present. The results are consistent with previous analyses of tholins (Mutsukura and Akita, Reference Mutsukura and Akita1999; Imanaka et al., Reference Imanaka, Khare, Elsila, Bakes, McKay, Cruikshank, Sugita, Matsui and Zare2004; Quirico et al., Reference Quirico, Montagnac, Lees, McMillan, Szopa, Cernogora, Rouzaud, Simon, Bernard, Coll, Fray, Minard, Raulin, Reynard and Schmitt2008; Mendoza-Téllez, Reference Mendoza-Téllez2022) but include additional bands corresponding to C=O or C–OH bands due to the CO2 present in the original gas mixture.

Figure 3. Above: Raman spectrum of the remnant solid after the irradiations. Below: Infrared spectrum of the same sample. The solid sample was obtained after the irradiation (1 h) of the 85% N2, 10% CH4 and 5% CO2 mixture.
Elemental analysis shows that low amounts of nitrogen are present in the solid sample obtained after the irradiation of the 85% N2, 10% CH4 and 5% CO2 atmosphere (Table 9). The total elemental percentage is far from 100%. This discrepancy may be attributed to the presence of oxygenated compounds. Given that CO2 was included in the gas mixture, the formation of oxygenated compounds in the precipitate post-irradiation is plausible. Previous research (Mendoza-Téllez, Reference Mendoza-Téllez2022) has established through pyrolysis and gas chromatography that the principal constituents of the yellowish precipitate—referred to as tholins, which emerge following the irradiation of N2, CH4 and CO2—encompass aromatic hydrocarbons, nitrogenous compounds (e.g. acetonitrile, cyanoacetylene) and oxygen-containing compounds (e.g. methanol, ketones). These results also match the C=O and C–O–H bands in Fig. 3. A potential explanation for the emergence of oxygenated compounds within the precipitates is the thermal dissociation of CO2, yielding CO and O2 (Navarro-González et al., Reference Navarro-González, McKay and Mvondo2001). Consequently, oxygen may become available for the synthesis of organic compounds, such as ketones. A comprehensive analysis and elucidation of the mechanisms underlying tholin production are beyond the scope of this work.
Table 9. Elemental analysis of the solid sample (obtained after 1 h irradiation of 85% N2, 10% CH4 and 5% CO2 mixture)

Results are represented as percentages by weight. The instrument's limit of quantification was 1% for C, H, N and S.
Production of HCN in relation to the simulated energy from shockwaves
The 85% N2, 10% CH4 and 5% CO2 atmosphere was selected to study the production of HCN at different laser irradiation times (between 0 and 6 h). The exposure time of the simulated early Earth atmosphere was converted into dissipated energy by multiplying the exposure time by the pulse energy (300 mJ) of the laser irradiation to obtain the HCN formation rate due to the energetic discharge of the laser in molecules per unit of energy. Figure 4 shows the HCN production relative to the dissipated energy. At the longest irradiation time (6 h, corresponding to 64 800 J) a low abundance of cyanoacetylene was observed among the irradiation products.

Figure 4. Effect of the duration of irradiation on a simulated primitive Earth atmosphere. The sample conditions were: 300 mJ per pulse, 1000 mbar and a frequency of 10 Hz.
According to Fig. 4, the number of HCN molecules produced per J increases until it reaches a maximum at 10 000 J; after that, the number of molecules decreases and then remains almost constant for larger energies. Considering the most optimistic value (6 × 1018 molecules J−1), 1.5 × 1047–3.6 × 1047 molecules of HCN could have been formed by the release of shock energy into the atmosphere, if we consider the totals in Tables 2 and 3, or 1.3 × 1046–3.1 × 1046 molecules of HCN if we consider our more restrictive values. This implies that, in the first case, between 6.6 × 1024 and 1.6 × 1025 g of HCN could have been formed or, in the second case, between 5.7 × 1023 g and 1.4 × 1024 g of HCN could have been formed. The mass of the Earth is 5.97 × 1027 g; thus, the estimated amount of HCN that could be produced is 0.26% of the mass of the Earth at most.
Discussion
The results obtained for the atmospheres proposed in the first set of experiments (section ‘Atmospheres without a nitrogen source’) show that hydrocarbons can be formed due to irradiation. Although these compounds do not have great relevance for prebiotic chemistry, it is assumed that the formation of other possibly relevant compounds is inhibited due to the presence of carbon dioxide. Other authors (Bar-Nun and Shaviv, Reference Bar-Nun and Shaviv1975; Scattergood et al., Reference Scattergood, McKay, Borucki, Giver, van Ghyseghem, Parris and Miller1989; Sugisaki et al., Reference Sugisaki, Mimura and Kato1994; McKay and Borucki, Reference McKay and Borucki1997; Ferus et al., Reference Ferus, Rimmer, Cassone, Knízek, Civiš, Šponer, Ivanek, Šponer, Saeidfirozeh, Kubelík, Dudzák, Petera, Juha, Pastorek, Křivková and Krůs2020) have observed the possible effects of impacts on the atmosphere in the production of organic compounds, and have also produced hydrocarbons and HCN, despite starting with different gas mixtures of similar composition.
Although the atmospheric composition of the early Earth is still a matter of debate, one of several proposals is that the early atmosphere resembled the current atmospheric conditions of Titan (Clarke and Ferris, Reference Clarke and Ferris1997; Trainer et al., Reference Trainer, Pavlov, DeWitt, Jimenez, McKay, Toon and Tolbert2006), for example, their similar isotopic ratios of H, C, N and O suggest a possible cometary origin of Earth atmosphere (Trigo-Rodriguez and Martín-Torres, Reference Trigo-Rodriguez and Martín-Torres2012). The presence of an organic haze would have contributed to protecting photo-dissociable compounds, such as methane and ammonia (Sagan and Chyba, Reference Sagan and Chyba1997). If the atmosphere of the primitive Earth were dense, organic haze and meteoric metals would have acted as catalysts for forming complex organic molecules from methane and CO2. Therefore, the atmosphere of Titan is a system we can consider understanding possible prebiotic pathways for forming relevant organic compounds on early Earth (Trigo-Rodriguez and Martín-Torres, Reference Trigo-Rodriguez and Martín-Torres2012). The atmospheres chosen in this work (sections ‘CO2, CH4 and N2 atmospheres’ and ‘Production of HCN in relation to the simulated energy from shockwaves’) are similar to that of Titan.
The results show that as the percentage of CO2 in the mixtures decreases, the production of organic compounds is favoured, and even HCN is produced. HCN is essential for chemical evolution because it can be a precursor (through other prebiotic reactions) to molecules such as RNA and early proteins. Another compound of interest for prebiotic chemistry is cyanoacetylene; this molecule was obtained at the maximum irradiation of the 85% N2, 10% CH4 and 5% CO2 gas mixture. This compound is a common electric discharge product on a methane–nitrogen mixture. It is also relevant to prebiotic chemistry because it can be a precursor of compounds such as cytosine, aspartic acid and asparagine (Orgel, Reference Orgel1998).
Conclusions
The results of subjecting these samples of possible primitive atmospheres to a simulation of the energy released by the shock waves produced by impactors of asteroidal and cometary material show that if the oxidizing character of the atmosphere is dominant, the production of organic compounds is diminished. Even so, they were produced in the atmospheres (Table 7) containing ≤10% CO2.
The energy released into the atmosphere by the impactors that hit the Earth between 4.2 and 3.8 Ga before the present could have provided the energy to produce organic compounds that could have played an important role in the chemical evolution period, such as HCN and NO. Although the estimates made in this work are upper limits and the atmospheres considered were favourable to their formation, the possible quantity of these compounds, which is equivalent to a few per cent of the mass of the Earth, should be considered. In the future, evaluating the dynamics of the reaction of the different compounds produced could be important.
While the Young Sun paradox seems to indicate that due to the lower luminosity of the Sun in its early years promoting very low temperatures on the early Earth, geological evidence, such as the dating of zircons mentioned above, indicates that the Earth had favourable conditions for chemical evolution and the subsequent origin of life. For this to be possible, greenhouse gases, such as CO2 and CH4, are necessary; compounds like CH4 and NH3 can be rapidly photolysed and would not accumulate in high enough concentrations to prevent freezing ocean water (Bada, Reference Bada2004). Furthermore, there were no apparent sources of methane on the primitive Earth. However, this work shows that large impacts would be major contributors to the presence of this compound and cause transient reduced atmospheres. Around 546 cosmic objects with diameters >115 km could have impacted our planet during its first 700 Ma of history, providing multiple opportunities for these conditions. The energy released to the atmosphere by the entry of such a cosmic body could have produced between 5.7 × 1023 and 1.6 × 1025 g of HCN.
Acknowledgements
The authors are most grateful to Chris McKay for his comments and suggestions, which helped to improve this manuscript. L. R.-V. acknowledges the DGAPA-UNAM Fellowship for Postdoctoral Researchers, Instituto de Geofísica UNAM and Instituto de Ciencias Nucleares UNAM, especially to the Laboratorio de Evolución Química and the ‘Unidad de Laboratorios Dr Rafael Navarro González’ for the support and the use of the facilities for this research. The authors are grateful to MSc P. Molina-Sevilla, C. Camargo-Raya, M. Ham-Reyes and MSc C. A. Fuentes-Carreón for their technical support and MSc V. H. Lemus-Neri from the Unidad de Servicios de Apoyo a la Investigación y a la Industria (USAII-UNAM) for their help with the elemental analysis. The PAPIIT grant IN114122 supported this work. The internal project P-107 from Instituto de Geofísica also supported this work.
Author contributions
G. C.-T. derived the theory and L. R.-V. and J. d. l. R. performed the simulations. All authors contributed equally to analysing data, writing the manuscript and reaching conclusions.
Competing interest
None.