Implications
The implications of this paper are for animal scientists and policy makers. In the context of sustaining global food security, the use of potentially human-edible feeds as supplements to forage feeds in ruminant diets should be restricted to the rectification of dietary imbalances in higher-producing livestock. The potential of all-forage diets should be demonstrated for a wide range of ruminant production systems to deliver high-quality milk and meat, control input costs, and at the same time utilise land not suitable for high-yielding arable crop cultivation.
Introduction
The nutrition of ruminant livestock is dominated globally by locally grown forage feeds, that is whole plants, either consumed in situ by grazing animals at pasture, or consumed as silage or hay when pasture is limiting or unavailable due to adverse weather. However, within the ruminant livestock sector there is a wide range in types of feed inputs, especially the proportion of forages making up the total diet (Council for Agricultural Science and Technology (CAST), 1999).
Of concern to global human food security, defined as an adequate annual supply of human-edible food to meet the annual demand of the human population, is the use in livestock diets of potentially human-edible foods. It has been estimated that a third of the annual global cereal grain harvest is used for livestock feed rather than directly as human food (Alexandratos and Bruinsma, Reference Alexandratos and Bruinsma2012; Eisler et al., Reference Eisler, Lee, Tarlton, Martin, Beddington, Dungait, Greathead, Liu, Mathew, Miller, Misselbrook, Murray, Vinod, Van Saun and Winter2014). CAST (1999) estimated that between 1993 and 2020 the growth in cereal grain use as livestock feed would be 1.4% per annum, comprising annual growth rates of 2.7% in developing countries and 0.7% in developed countries. With global livestock numbers expected to exceed 35 billion chickens, 2.5 billion cattle (all bovines); 2.5 billion sheep and goats; 1 billion pigs and 25 million camels (Thornton, Reference Thornton2010), by 2050 the quantity of arable crops given to livestock might exceed that used by humans (Bailey et al., Reference Bailey, Froggatt and Wellesley2014).
Typically, monogastric livestock (pigs and poultry) diets are comprised predominantly of wheat (Triticum spp.) and maize (Zea mays) grain, with soyabean meal (Glycine max) as the major source of supplementary protein. CAST (1999) and Wilkinson (Reference Wilkinson2011) found that ruminants converted potentially human-edible feeds to animal product with similar efficiency to monogastric livestock, mainly because human-edible feeds comprised a low proportion of the total feed input to the system (Table 1). In the context of typical Northern European systems of livestock production, input of human-edible CP ranged from 0.03 of total diet CP for lamb production to 0.71 of total diet CP for poultry meat (broiler) production (Table 1). However, despite large differences between systems in human-edible protein input, the range in protein efficiency (output/input) between systems was much smaller, tending to be higher for ruminant than for monogastric systems and >1.0 for upland beef and grass-based milk production (Table 1).
Table 1 Proportion of potentially human-edible feed in the total feed input and ratio of animal protein output to human-edible protein input for a range of livestock systems (from Wilkinson, Reference Wilkinson2011)

DM=dry matter.
There is also concern that the increasing global scale of livestock units may constitute a threat to potable water quality as a result of the leaching of nitrate into ground water and rivers, and to air quality as a result of emissions of ammonia to the atmosphere, notwithstanding concerns over greenhouse gas (GHG) emissions, which are significantly higher per unit of product from ruminant systems than from monogastric systems due to methane from enteric fermentation. Although livestock manure is a valuable source of recycled fertilizer N, livestock systems are substantially less efficient than crop production in terms of N use efficiency (NUE), defined as N in product as a proportion of total N input (Audsley and Wilkinson, Reference Audsley and Wilkinson2014).
Further, ruminant livestock production is less efficient in terms of NUE than monogastric systems of production due to the nature of rumen fermentation, which relies on a balance between fermentable energy and degradable protein and also the relatively lower digestibility of forages in ruminant diets compared with cereal grains and soyabean meal – the predominant feeds in diets for pigs and poultry. Dijkstra et al. (Reference Dijkstra, France, Ellis, Strathe, Kebreab and Bannink2013) calculated a theoretical maximum ruminant NUE of 0.45, but more typically this is <0.30, especially in high-forage systems where fermentable energy and degradable protein are not balanced in the rumen (Lee et al., Reference Lee, Merry, Moorby, Humphreys, Theodorou, Macrae and Scollan2003).
In this paper, the inputs of potentially human-edible animal feed to different ruminant livestock systems are outlined in relation to output of animal product. The characteristics of forages that might limit output and efficiency are discussed in relation to potential levels of livestock output to identify systems where the use of potentially human-edible feeds or by-products might be justified as supplements to forages to meet animal nutritional requirements. Examples are given to justify the use of potentially human-edible feeds. Finally, the scope for replacing potentially human-edible feeds in all-forage ruminant systems is explored.
Material and methods
Literature sources were used to provide evidence of the extent of potentially human-edible feed use in ruminant livestock systems and to generate specific examples of systems, or parts of systems where the use of potentially human-edible feeds might be justified. Examples were drawn from the literature of the extent to which forages and human-inedible by-product feeds might be used to replace potentially human-edible feeds in high-yielding systems of ruminant livestock production.
Results and discussion
Human-edible feed use in milk production
Examples of the range of potentially human-edible feed use in different systems of bovine milk production are given in Table 2. At one extreme, milk production is reliant almost entirely on grazed pasture with limited inputs of silage and either grain or by-products such as extracted palm (Elaeis guineensis) kernel meal to rectify seasonal deficiencies in pasture availability. However, daily milk output in the grass-based system is restricted by limits to grazed pasture intake. In order to achieve higher levels of daily milk yield, concentrate inputs are required to achieve higher daily intakes. Thus, a diet based on grass silage or straw plus by-products is capable of supporting a higher average daily output of milk per cow because the input of potentially human-edible and inedible by-product feeds from the human food and drink industry is reflected in higher daily dry matter (DM) intake.
Table 2 Human-edible feed input and nitrogen use efficiency (NUE) in different systems of milk production

DM=dry matter.
1 Fat+protein; 35 g protein/kg milk for grazed pasture, 31 g protein/kg milk for other diets.
2 Human-edible proportions from Wilkinson (Reference Wilkinson2011).
3 NUE; milk N as proportion of total N intake.
4 Autumn calving, seasonally variable diet.
Higher levels of potentially human-edible feed inputs are typical of total mixed rations (TMR) comprising silage and concentrates. Daily milk output from animals kept in this type of production system is relatively high, as is the input of concentrate feeds, including a higher proportion of human-edible feeds than in diets based on grass silage diet or on by-product feeds (Table 2). It is notable that NUE is directly related to milk solids output, reflecting a closer balance between total N intake and animal net protein requirement for housed systems compared with the pasture or grass silage diets.
Edible protein output per unit human-edible feed protein input was high (>30) for the pasture-based system because total input of pasture supplement was severely restricted to only 64 kg DM/head over the total lactation period (Clement et al., Reference Clement, Dalley, Chapman, Edwards and Bryant2016). Protein efficiency was >1.0 for the grass silage and by-product diets but was <1.0 for the TMR based on grain and maize silage diet (Table 2).
A further feature of higher milk production systems is that they are typically based around heavier Holstein cows which produce a ‘lower quality’ milk in terms of milk solids (<4% fat and 3% protein) compared with more grazing systems based on Jersey (~4.5% fat and 4% protein) or Friesians (~4% fat and 3.5% protein) (Dobson et al., Reference Dobson, Smith, Royal, Knight and Sheldon2007). These differences need to be taken into consideration when comparing milk volumes from different production systems. Nevertheless, it is important to recognise that in an industry where the financial margin between profit and loss is small, farmers must consider greater reliance on pasture to improve resource use efficiency. The profitability of dairy farms is driven by control of input costs over and above milk price (Agriculture and Horticulture Development Board, 2012a). In producing milk from pasture, the most efficient approach is to achieve maximum intake of pasture combined with strategic supplementation to balance input costs against income (Agriculture and Horticulture Development Board, 2012b).
Although bovine milk makes up the majority of global production (~703 Mt/year) with 83% from cattle and 13% from buffalo, small ruminants make an important contribution to milk production (~15 Mt goat milk/year and ~9 Mt sheep milk/year, Food and Agriculture Organisation of the United Nations (FAO), 2010). Traditionally, milk production from small ruminants is from high-forage systems, usually scrub grazing or mountain pasture providing vital nutrition for subsistence farmers, or high-value niche products. The small ruminant industry in developed countries is becoming increasingly intensive with higher-yielding animals being offered rations containing relatively high proportions of concentrate (Giger-Reverdin et al., Reference Giger-Reverdin, Rigalma, Desnoyers, Sauvant and Duvaux-Ponter2014), even ‘non-forage’ diets (Bava et al., Reference Bava, Rapetti, Crovetto, Tamburini, Sandrucci, Galassi and Succi2001). Bava et al. (Reference Bava, Rapetti, Crovetto, Tamburini, Sandrucci, Galassi and Succi2001) reported the ability of goats to adapt to relatively low rumen pH driven by high concentrate rations, with little adverse effect of feeding non-forage-based diets during lactation. However, a recent case report on lameness and ruminal acidosis in intensive goat dairies indicated causative nutritional factors driven by low forage intake (Groenevelt et al., Reference Groenevelt, Anzuino, Smith, Phythian, Lee and Grogono-Thomas2015).
Human-edible feed use in beef production
There is also a wide range in potentially human-edible feed use in beef production. The cereal beef system, in which male calves from the dairy herd are reared from weaning to slaughter on a grain-based diet and slaughtered at 11 to 13 months of age, has a much higher potentially human-edible proportion than pasture-based beef systems (Table 1). Although this system is traditionally less common than suckler beef systems, it is rapidly becoming a major contributor to the European beef market with the removal of the EU milk quota in 2015 and an increasing supply of male calves from the dairy herd. These animals, depending on the male sire, have a lower musculature and propensity to finish off grass than more traditional beef breeds. Dairy–beef animals therefore require a higher energy density diet to reach finish for market, increasing the demand for cereal and human-edible feed. In any case notwithstanding dairy–beef, cereal-based rations represent the final finishing period in feedlots of weaned suckled calves from grazed cow–calf operations (CAST, 1999; Corona et al., Reference Corona, Rodriguez, Ware and Zinn2005).
Example diets were given by CAST (1999) to illustrate the large differences in human-edible feed use and in efficiency of animal edible protein output per unit of human-edible protein input between systems of milk and beef production in the United States and South Korea (Table 3). Although there have been developments since that time associated with intensification of milk and beef production in South-East Asia, it is likely that significant differences remain between the two regions due to local economic circumstances.
Table 3 Example diets for dairy cows and beef finishing in the United States and South Korea (CAST, 1999)

DM=dry matter.
1 Sunflower, soyabean and cottonseed meals and whole cottonseed.
Trends in human-edible feed use
In 1990/92 worldwide use of cereal grains in livestock feeds amounted to 600 million tonnes, of which 31% was used in developing countries (Hendy, Reference Hendy, Kleih, Grashaw and Phillips1995). By 2005 total cereal use for livestock had risen to 742 million tonnes, of which 38% was used in developing countries (FAO, 2010). As an example of a developed country, concentrate feed use by dairy cattle increased steadily in Great Britain in the period 1990 to 2013 (Figure 1). The graph illustrates a general trend in many other regions of the world, indicating that most of the increase in annual milk production per cow has been achieved through increased input of concentrate feeds containing significant proportions of potentially human-edible cereal grain and soyabean meal. However, the pattern post-2005 for further increases in compound feed use for moderate gain in milk yield signifies an over-reliance on concentrates which needs to be addressed, especially in relation to the control of feed input costs.
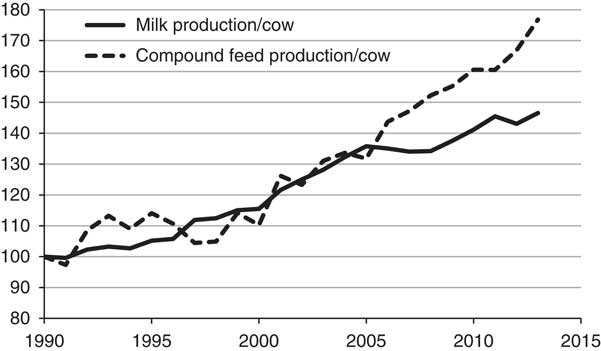
Figure 1 Trends in annual milk production and concentrate feed production per cow in Great Britain (1990=100). From Wilkinson and Allen (Reference Wilkinson and Allen2015).
Reasons for human-edible feed use
The use of concentrates may be justified on nutritional grounds in terms of meeting animal requirement for energy, especially in late pregnancy (sheep) early lactation (dairy cows) and the final period of growth (beef cattle). Moreover, there are specific situations (e.g. the high-yielding dairy cow) in which the requirement for metabolisable protein cannot be met by microbial protein synthesis in the rumen and an additional supply of undegraded dietary protein and/or essential amino acids is required. This is especially the case for methionine and lysine where protected supplementation has been shown to increase milk yield (Nichols et al., Reference Nichols, Schingoethe, Maiga, Briuk and Piepenbrink1998), whereas on high-forage diets histidine is often first limiting due to the greater reliance on microbial protein (Lee et al., Reference Lee, Theobald, Gordon, Leyland, Tweed, Fychan and Scollan2014).
Apart from the issue of competition between livestock and humans for land and food, concentrate feeding is associated with several negative aspects including higher input costs, animal health issues (sub-acute rumen acidosis, acute acidosis, ruminal parakeratotic hyperkeratosis) and the substitution effect. Very few energy supplements have a purely additive effect on forage intake as starch-based concentrates tend to reduce pH and fibre digestion with detrimental effects on intake of forage. The decrease in forage intake per kilogram increase in concentrate intake is dependent on the nature of both the forage and concentrate with a greater impact observed with higher digestibility forages (Conrad et al., Reference Conrad, Pratt and Hibbs1966).
Although the feeding of concentrates that contain potentially human-edible feeds often includes intercontinental movement of commodities with subsequent impact on carbon footprint (CFP) of land-use change and air/ship miles, there are environmental benefits to ruminants of potentially human-edible feeds as supplements to forage-based diets. First, methane production from enteric fermentation is lower per unit of DM intake when concentrates are included in the ration than when forage is the sole feed (Harper et al., Reference Harper, Denmead, Freney and Byers1999). Methane production in the rumen is a by-product of the removal of hydrogen produced during enteric fermentation. Forages contain higher proportions of fibre (cellulose) than concentrates, which favour the formation of acetate as a by-product of fibrolytic bacterial fermentation, but for every mole of acetate produced four moles of hydrogen are formed. Whereas high-starch concentrate supplements favour amylolytic fermentation with formation of propionate, which utilises two moles of hydrogen for every mole formed in the rumen (McDonald et al., Reference McDonald, Edwards, Greenhalgh, Morgan, Sinclair and Wilkinson2010). Further, concentrate-based rations are more digestible, have a greater rumen flow rate and reduced potential methane production, notwithstanding the higher protein content of many supplementary feeds which have been shown to reduce methane formation in the rumen (Ramin and Huhtanen, Reference Ramin and Huhtanen2015).
Second, there is often an imbalance between readily available energy and rapidly degraded N in the rumen on pasture-based diets, reflecting a relatively high total N intake. This imbalance decreases NUE as it is inversely related to total N intake (Ledgard et al., Reference Ledgard, Schils, Eriksen and Luo2009). For intensive grazing systems, higher-sugar grasses potentially offer a better balance between rumen-degradable protein and fermentable carbohydrates within the grass, resulting in greater NUE (Miller et al., Reference Miller, Moorby, Davies, Humphreys, Scollan, Macrae and Theodorou2001; Lee et al., Reference Lee, Merry, Moorby, Humphreys, Theodorou, Macrae and Scollan2003). Alternatively, supplementation with fermentable carbohydrates (e.g. grain or sugar beet (Beta vulgaris) pulp) is an effective strategy to increase capture of excess protein and increase microbial protein synthesis; this strategy is more effective at increasing NUE than altering the CP of the overall diet (Broderick, Reference Broderick2003; Sinclair et al., Reference Sinclair, Garnsworthy, Mann and Sinclair2014).
As N excretion is directly related to N intake (Castillo et al., Reference Castillo, Kebreab, Beever, Barbi, Sutton, Kirby and France2001), it follows that a reduction in daily N intake in ruminants grazing high-protein pasture is desirable from the point of view of increasing NUE and reducing nitrate leaching and gaseous emissions of nitrous oxide and ammonia to the atmosphere. One possible approach to reducing N intake is by providing a lower protein supplementary feed. Chaves et al. (Reference Chaves, Woodward, Waghorn, Brookes, Holmes and Laboyrie2002) emphasised the need to match composition of the supplement to composition of the pasture. Oilseed by-products such as palm kernel meal or soyabean meal are inappropriate in this situation because their concentrations of CP are too high. Alternatively, pastures can be used with reduced protein solubility, for example, red clover (Trifolium pratense) through the action of polyphenol oxidase (see below; Lee, Reference Lee2014).
Soyabean meal
Soyabean meal is a human-edible feed that fulfils a role as a source of high-quality protein and energy in diets for poultry, pigs and high-yielding dairy cows, but its use has been criticised on environmental grounds and alternatives have been evaluated (e.g. lupins for poultry diets; Lee et al., Reference Lee, Parkinson, Fleming, Theobald, Leemans and Burgess2016). In a study of the potential environmental impact of a range of diet formulations for dairy cows yielding 40 kg milk/day, Wilkinson and Garnsworthy (Reference Wilkinson and Garnsworthy2017, unpublished data) found the diet with the lowest feasible concentrate CFP included soyabean meal, which might seem counter-intuitive given the relatively high CFP of soyabean meal compared with human-inedible alternatives such as wheat distillers’ dried grains or rapeseed meal. Replacing soyabean meal by other by-products increased the CFP of the whole diet and decreased NUE because soyabean meal has a more favourable ratio of digestible undegraded protein to CFP than other feeds. Soyabean production in North America has lower GHG associated with its production than winter oilseed rape grown in Europe (Audsley and Wilkinson, Reference Audsley and Wilkinson2014) because soyabeans are leguminous and do not require fertiliser N. Lehuger et al. (Reference Lehuger, Gabrielle and Gagnaire2009) found a dairy cow diet containing Brazilian soyabean was more environmentally efficient than one containing European rapeseed meal when land-use change was excluded from the analysis. Huhtanen et al. (Reference Huhtanen, Hetta and Swensson2011) in a meta-analysis of supplementary proteins reported that rapeseed meal can be substituted successfully for soybean meal on an isonitrogenous basis and that most feed evaluation systems overestimate metabolisable protein concentration of soybean relative to rapeseed.
Land-use change, especially rain forest destruction, has been cited as a major reason for not using soyabean meal, but the issue is not straightforward. For a detailed review of land-use change in soyabean production see Opio et al. (Reference Opio, Gerber, Mottet, Falcucci, Tempio, Macleod, Vellinga, Henderson and Steinfeld2013). The trend to more soyabean meal being produced from land in arable cultivation for more than 20 years will help to sustain soyabean meal as a suitable raw material for inclusion in low CFP diets because of its high concentration of both CP and metabolisable energy (ME) in addition to its superior amino acid profile. But in terms of competition for arable land for food production, human-inedible alternatives to soyabean meal such as rapeseed meal are to be preferred as sources of supplementary protein in diets for ruminants.
Characteristics of forages that limit intake and efficiency of feed use
Forage crops have not been ‘designed’ to contain a perfect balance of nutrients for ruminant production. Ruminants have evolved to utilise their low energy density and excess N through slower growth rates and the return of N to the soil to fertilise subsequent pasture growth. However, issues arise when slow growth rates, moderate milk yields and low NUE do not meet production demands. For all-forage diets DM intake and consequently energy intake is predominately driven by physical distension of the rumen (Conrad et al., Reference Conrad, Pratt and Hibbs1966), although other negative feedbacks such as acetate and ammonia may also be involved (Moorby and Theobald, Reference Moorby and Theobald1999). Figure 2 shows the energy demands of a dairy cow yielding different volumes of milk and due to the limitations of DM intake the maximum energy intake from three pastures: low ME (10 MJ), median ME (11.6 MJ) and the theoretical maximum ME of 13.6 MJ/kg DM calculated from constituents of forage by Waghorn (Reference Waghorn2007). For the highest daily milk yield of 45 l no forage diet could provide the energy demand of the cow. Even at 35 l/day the median ME could not provide the energy demand. Therefore, for modern high-yielding dairy cows an all-forage diet is simply not able to provide the energy needed for lactation and therefore the need for strategic supplementation. Future development of high-lipid grasses may provide a solution for higher energy but these are many years away from commercial use (Hegarty et al., Reference Hegarty, Yadav, Lee, Armstead, Scollan, Powell and Skot2013).

Figure 2 Energy demand for variable milk yields (3.2% protein; 3.5% fat) for a 650 kg mid-lactation dairy cow v. the energy intake predicted from a low metabolisable energy (ME) forage (10 MJ/kg dry matter (DM)), median ME (11.6 MJ/kg DM) and the theoretical maximum ME from forage (13.6 MJ/kg DM; Waghorn, Reference Waghorn2007) predicted using Agricultural and Food Research Council (1995).
The concentration of CP in grass pre-grazing typically contains >200 g/kg DM (Holmes et al., Reference Holmes, Brookes, Garrick, Mackenzie, Parkinson and Wilson2002; Wilkinson et al., Reference Wilkinson, Allen, Tunnicliffe, Smith and Garnsworthy2014), excessively high in relation to animal requirement. Although there is a marked decline in CP from about 330 g CP/kg DM at the three-leaf stage of growth to about 70 g CP/kg DM at full flowering (Beever et al., Reference Beever, Offer and Gill2000), the problem of excess N intake (and excretion) is compounded by the grazing selection differential. Selection of leaf in preference to stem results in the grazing animal consuming herbage of higher quality than the average for the whole sward. The grazing selection differential for CP has been quantified at between 1.1 and 1.5, depending on efficiency of pasture utilisation (Stockdale and Dellow, Reference Stockdale and Dellow1995; Jacobs et al., Reference Jacobs, McKenzie and Ward1999). Thus, at a relatively high efficiency of pasture utilisation (e.g. 75%), which would be a reasonable target under well-managed grazing systems, the grazing animal can consume herbage about 10% higher in CP concentration than the average, that is, 220 g CP/kg DM in the herbage DM intake when the average for the pre-grazed pasture allowance is 200 g CP/kg DM. With more mature herbage on offer and/or higher quantities of residual herbage (and lower efficiency of utilisation) the grazing animal effectively negates any reduction in overall pasture CP concentration by rejecting stem and mature leaf of below-average CP. Forage breeding has improved the balance of readily available energy and rumen-degradable protein. Grasses with higher levels of water-soluble carbohydrate, as already mentioned, have been used to increase the supply of readily available energy to increase NUE (Lee et al., Reference Lee, Merry, Moorby, Humphreys, Theodorou, Macrae and Scollan2003), milk yield (Miller et al., Reference Miller, Moorby, Davies, Humphreys, Scollan, Macrae and Theodorou2001) and animal growth rate (Lee et al., Reference Lee, Jones, Humphreys, Moorby, Theodorou, Macrae and Scollan2001). On the other side of the imbalance an enzyme system in red clover (polyphenol oxidase) has been shown to slow down protein degradation in the rumen and thus improve NUE through improved balance with energy release (Lee, Reference Lee2014).
Future outlook and potential ruminant production from all-forage diets
The drive to increase output per animal has led to excessive use of potentially human-edible feeds in the diets of ruminants, especially in developed countries. Use of human-inedible by-product feeds in concentrate formulations is significant in regions where there is a large human population and an ample supply from the human food and drinks industries (Wilkinson, Reference Wilkinson2013). However, the supply of human-inedible raw materials is finite and future increases in supply should be used in diets for monogastric livestock that cannot use grazed pasture and forage feeds.
Land use
The relative use of different types of land by different classes of livestock is often overlooked when discussing the future of livestock production practices, as part of food security, with emphasis placed on carbon emissions and water use (Eshel et al., Reference Eshel, Shepon, Mokov and Milo2014). Although emissions must be considered and improvements made to practices to mitigate and control them (Bryngelsson et al., Reference Bryngelsson, Wirsenius, Hedenus and Sonesson2016), the vital role in delivering high-quality food by rain-fed, pasture-based, ruminant livestock kept on land not suitable for alternative cultivation must be fully recognised (Eisler et al., Reference Eisler, Lee, Tarlton, Martin, Beddington, Dungait, Greathead, Liu, Mathew, Miller, Misselbrook, Murray, Vinod, Van Saun and Winter2014; Van Zanten et al., 2016).
Wilkinson et al. (2017, unpublished data) estimated that it takes three times as much arable (cultivated) land to produce the human-edible feeds (mainly cereal grain and soyabean meal) used in the production of a unit of edible animal protein in typical Northern European systems of pig meat, poultry meat and egg production compared with typical Northern European forage-based systems of beef and lamb production (Table 4). In contrast, relatively large areas of grassland are required per unit of animal protein output in the case of typical ruminant livestock systems, though there is considerable variation in land requirement within ruminant systems reflecting differences in type of product (milk v. meat), livestock species (cattle v. sheep) and type of diet (cereal beef v. grass-fed dairy beef). Further, the inverse relationship between efficiency of feed conversion (output per unit of input), land area required and GHG emissions per unit of animal protein output (Bryngelsson et al., Reference Bryngelsson, Wirsenius, Hedenus and Sonesson2016) focusses attention on type of land, climate and potential crop yield in determining the most efficient use of this scarce resource for human food production.
Table 4 Land required per tonne of animal protein output for a range of livestock systems (from Wilkinson et al., 2017, unpublished data)

Alternative approaches to ruminant livestock production are essential for future global food security. One approach is to produce milk, beef and lamb from grassland using forages as the sole dietary ingredient. However, some grassland, especially that in lowland regions, might be used more efficiently for human food production through arable cropping than by growing forage crops since ruminants are particularly relevant to add value to biomass produced on marginal grassland. To determine whether or not a net gain in output might accrue from the use of a particular type of land by ruminants rather than through arable cropping, the land use ratio (LUR) concept developed by Van Zanten et al. (2016) may be used. The LUR is defined as the maximum amount of human-digestible protein (HDP) produced from food crops grown on the land used to produce a kilogram of animal product divided by the amount of HDP in a kilogram of animal product. A LUR value >1.0 indicates that the land would be better used for the production of arable crops, whereas a value <1.0 implies that the optimal use of that land would be for the production of ruminant milk or meat. For example, Van Zanten et al. (2016) calculated that the LUR for dairy cows was 2.10 when the animals were kept on sandy soils and 0.67 when kept on peat soils. The LUR was lower for cows on peat soils than for sandy soils because the peat soils were unsuitable for direct production of food crops. Using this approach identifies those types of land on which ruminant livestock are more efficient converters of plant biomass than other classes of livestock or arable cropping for direct production of human food.
A further consideration, relevant to future human health and well-being, is that all-forage-based diets produce ruminant meat and milk with a more beneficial composition of fatty acids and a greater concentration of certain vitamins (A and E) whose antioxidant capacity also improves the shelf life of the product, reducing waste (Warren et al., Reference Warren, Scollan, Enser, Hughes, Richardson and Wood2008; Daley et al., Reference Daley, Abbott, Doyle, Nader and Larson2010).
Milk from all-forage diets
The potential of an all-forage diet to support milk production from cows and heifers in the United Kingdom was investigated by Rae et al. (Reference Rae, Thomas, Reeve, Golightly, Hodson and Baker1987). High-digestibility ryegrass (Lolium spp.) silage was given to the cows from calving in late winter to the start of the grazing season. Thereafter, the animals received grazed pasture as the sole feed until the autumn when the cows were housed and given lower digestibility silage for the remainder of the lactation and during the dry period. Whole lactation milk yields averaged 4680 kg for cows and 4006 kg for heifers at 3.94% fat and 3.14% protein. Animal health and fertility were satisfactory.
In a study of small organic dairy farms, Ertl et al. (Reference Ertl, Knaus and Steinwidder2014) described the characteristics of eight farms in which no concentrate feeds were given to the animals over a 2-year period. The results of the study revealed that the potential of an all-forage diet was 5093 kg milk/cow per annum at 4.07% fat and 3.27% protein. It is notable that five of the eight farms used no silage at all, relying on hay as the conserved forage feed. Calving interval was higher but veterinary costs were lower on the zero concentrate farms than on 49 comparable organic farms where typical levels of concentrate feeds were used and where milk production per cow was higher (1657 kg concentrate/cow per year and 6824 kg milk/cow per year). Critically, there was no evidence that a zero concentrate strategy was reflected in reduced profitability.
In a review by Fulkerson and Trevaskis (Reference Fulkerson and Trevaskis1997) they concluded that a milk yield of 20 to 25 l per day from Friesian cows was achievable from pasture as a sole feed agreeing with the predicted requirements in Figure 2. Animal genetic merit, availability of pasture and pasture species all influence the actual level of production but responses could be modest. For example, in high producing cows they showed that available pasture (DM on offer) must increase by 27 kg DM/cow per day to increase milk yield by 2.6 l/day. The report also concluded that C4 grasses typically yielded 5 l/day less than C3 grasses, whereas clover may give 3.5 l/day more than grass alone, although these studies were based on relatively low-producing animals. The potential exists to increase milk production from pasture by improving the protein: carbohydrate ratio, as discussed above. One strategy commonly being used in high-grazing regions is to ensure a high level of non-structural carbohydrates in the pasture by adjusting time of grazing with Miller et al. (Reference Miller, Moorby, Davies, Humphreys, Scollan, Macrae and Theodorou2001) reporting an increase in pasture water-soluble carbohydrate concentration from 150 g/kg DM at 0600 h to >200 g/kg DM at 1800 h (Miller et al., Reference Miller, Moorby, Davies, Humphreys, Scollan, Macrae and Theodorou2001).
Meat from all-forage diets
For ruminant meat production from all-forage diets, lamb production systems (Table 1) currently utilise little supplementary feed (trace minerals and concentrates) for a short period in late pregnancy and early lactation. For beef, as already discussed, there is an increasing reliance on concentrates in finishing rations. However, high levels of production are achievable from pasture and high ME silage. Warren et al. (Reference Warren, Scollan, Enser, Hughes, Richardson and Wood2008) reported the finishing of Holstein-Friesian and Aberdeen Angus steers in 741 and 755 days at 614 and 686 kg, respectively, off grass silage ad libitum with no supplemental feed. Lee et al. (Reference Lee, Theobald, Tweed, Winters and Scollan2009) finished dairy cull cows on grass and red clover silage ad libitum with average daily live weight gains of 1.3 kg. Both studies indicate that feeding high-quality silage can result in acceptable live weight gains.
Future research
The complex interactions between land use capability, livestock production system, environmental impact, product quality and consumer demand require further detailed multi-disciplinary research so that policy makers and producers can make informed judgements about allocating limited resources and financial investment to different livestock sectors, including appropriate genetic research relevant to both the animals themselves and their feed inputs.
Total land required per unit of animal protein output is considerably greater for ruminant systems than for monogastric systems, especially suckler beef and lamb production which involve feeding a breeding female throughout the production cycle (Table 4). This illustrates the need to consider soil quality and climate in assessing land capability as an essential component in research analyses of the relative efficiencies of livestock production systems. Priorities for future research should include identifying appropriate ways of utilising marginal grassland for ruminant milk and meat production, assessing agricultural systems to deliver optimum nutrient provision (micro- and macro-nutrients) for human nutrition per area of land, and establishing the limits to the use of human-inedible by-product feeds in diets for pig and poultry systems.
As an example, research at the North Wyke Farm Platform is determining the potential of all-forage beef finishing systems using Life Cycle Analysis (www.rothamstedresearch/farmplatform). The approach will elucidate the true impact and value potential of three pasture management systems (permanent pasture; clover and grass swards; and reseeded pasture) through mapping animal performance and product quality, environmental impact, labour cost and economic returns using primary data sets. Latest findings indicate that live weight gain solely from pasture from weaning to finish averaged 1.0 kg/day for all treatments, however, CFP was lowest on the clover and grass system as a result of lower fertiliser N requirement (Thompson et al., Reference Thompson, Orr, Dungait, Murray and Lee2014; McAuliffe et al., Reference McAuliffe, Takahashi, Eisler, Harris, Orr and Lee2016). Achieving lifetime cattle growth rates of >1 kg/day live weight gain on pasture through good pasture management to finish at <20 months avoids a second winter where maintenance feed requirements are higher than in summer and risk of damage to pastures from treading is increased. Increasing cattle growth rates on pasture will usually require lower stocking rates (1.5 LU/ha), but if this is associated with higher daily live weight gain per animal, the reduced stocking rate is balanced to ensure no reduction of overall profitability, with the added benefit of significantly lower cost of feed inputs.
Conclusions
Grazed pasture, the single most important forage feed for ruminants due to its low unit cost and widespread global availability, will continue to sustain the profitability of ruminant livestock production systems. Potentially human-edible feeds have vital roles to play in complementing grazed pasture and conserved forages, to increase total diet DM intake and rectify nutritional imbalances, especially for high-yielding dairy cows. By-product feeds can replace potentially human-edible feeds as supplements to pasture and forage feeds, but limited availability may restrict their use in some regions of the world.
Acknowledgement
This paper is an invited contribution following the First Global Farm Platform conference (12 to 15 January 2016, Bristol, UK). The Global Farm Platform is an international initiative linking research farms around the globe to develop solutions for sustainable ruminant livestock production (www.globalfarmplatform.org).