1. Introduction
The Late Carboniferous to Triassic was marked by the assembly of a significant portion of the European crust, and these tectonic events are critical to our understanding of the amalgamation of Pangaea and its evolution. A key event in the assembly of Pangaea was the collision of Gondwana (and Gondwana-derived terranes) with Laurussia during the Acadian–Variscan–Alleghanian Orogeny in the Late Carboniferous (i.e. McCann, Reference McCann2008; Stephan et al. Reference Stephan, Kroner, Romer and Rösel2019; Franke et al. Reference Franke, Ballèvre, Cocks, Torsvik and Żelaźniewicz2020;). The Variscan Orogen is exposed in Western and Central Europe, but also occurs north of the West African Craton in Morocco and Algeria, and in the Appalachian Mountains in northeastern North America, where it is termed the Alleghanian Orogeny (Michard et al. Reference Michard, Soulaimani, Hoepffner, Ouanaimi, Baidder, Rjimati and Saddiqi2010; Stephan et al. Reference Stephan, Kroner, Romer and Rösel2019; Franke et al. Reference Franke, Ballèvre, Cocks, Torsvik and Żelaźniewicz2020). To the southeast of the Bohemian Massif in central Europe, the Variscan Belt is either overprinted by younger orogens or covered by sedimentary rocks. However, Variscan basement massifs are known from the Alps, the Carpatho-Balkanides, the Hellenides, parts of the Pontides and further to the east into the Caucasus (Sengör et al. Reference Sengör, Altiner, Cin, Ustaömer and Hsü1988; Haydoutov, Reference Haydoutov1989; Stampfli, Reference Stampfli, Bozkurt, Winchester and Piper2000; Himmerkus et al. Reference Himmerkus, Anders, Reischmann, Kostopoulos, Hatcher, McBride and Martínez Catalán2007; Gawęda & Golonka, Reference Gawęda and Golonka2011; Mayringer et al. Reference Mayringer, Treloar, Gerder, Finger and Shengelia2011; von Raumer, Reference Von Raumer2013; Zulauf et al. Reference Zulauf, Dörr, Fisher-Spurlock, Gerder, Chatzaras and Xypolias2014; Antić et al. Reference Antić, Peytcheva, Von Quadt, Kounov, Trivić, Serafimovski, Tasev, Gerdjikov and Wetzel2016; Okay & Topuz, Reference Okay and Topuz2017; Spahić & Gaudenyu, Reference Spahić and Gaudenyu2018; Franke et al. Reference Franke, Ballèvre, Cocks, Torsvik and Żelaźniewicz2020).
The Strandja Zone straddling the border between Bulgaria and Turkey forms the focus of this study. The Strandja Zone occurs between the Carpatho-Balkanides to the north and west and the Pontides to the east, and has been variously assigned to either zone (e.g. Okay et al. Reference Okay, Satur, Tüysüz, Akyüz and Chen2001; Sunal et al. Reference Sunal, Natal’in, Satir and Toraman2006; Aysal et al. Reference Aysal, Şahin, Güngör, Peytcheva and Öngen2018). It contains a series of units related to the Variscan and Alpine orogens, and is key to a better understanding of the Variscan Belt in this sector of Pangaea. The post-Variscan evolution of the Strandja Zone is interpreted by many authors as related to the subduction of the Palaeo-Tethys Ocean beneath the southern margin of Laurussia (e.g. Natal’in et al. Reference Natal’in, Sunal, Gün, Wang and Zhiqing2016; Aysal et al. Reference Aysal, Şahin, Güngör, Peytcheva and Öngen2018; Bonev et al. Reference Bonev, Filipov, Raicheva and Moritz2019a). The Palaeo-Tethys Ocean remained open to the south of the Variscan Belt until the late Palaeozoic, and then the initiation of northward subduction led to the final closure of the Palaeo-Tethys Ocean in the Middle Triassic (Sengör, Reference Sengör1979, Reference Sengör1984; Sengör et al. Reference Sengör, Altiner, Cin, Ustaömer and Hsü1988; Zulauf et al. Reference Zulauf, Dörr, Fisher-Spurlock, Gerder, Chatzaras and Xypolias2014). Furthermore, this northward subduction likely triggered rifting and the opening of back-arc basins along the Laurussian margin, e.g. the Maliac and Kure basins (e.g. Stampfli, Reference Stampfli, Bozkurt, Winchester and Piper2000; Stampfli & Kozur, Reference Stampfli, Kozur, Gee and Stephenson2006).
The Variscan Belt in Europe is characterized by an abundance of granitoids (e.g. Bonin et al. Reference Bonin, Azzouni-Sekkal, Bussy and Ferrag1998; McCann, Reference McCann2008). Many of the granitoids in the Variscan Belt of southeastern Europe remain undated and intrude into poorly characterized basement which was also affected by Alpine metamorphic overprinting of variable intensity. In this study, we present the first laser ablation – inductively coupled plasma – mass spectrometry (LA-ICP-MS) U–Pb zircon ages and geochemical results from one of the largest intrusive bodies in the Sakar Unit of the Strandja Zone: the Izvorovo Pluton. The emplacement of this pluton was interpreted by Ivanov et al. (Reference Ivanov, Gerdjikov and Kounov2001) and Gerdjikov (Reference Gerdjikov2005) as the heat source for Late Jurassic to Early Cretaceous metamorphism in the Sakar Unit of the Strandja Zone. Bonev et al. (Reference Bonev, Filipov, Raicheva and Moritz2019a), however, regarded the pluton as Carboniferous based on ages from the Ustrem Pluton. Therefore, obtaining accurate and precise U–Pb geochronological data for the Izvorovo Pluton is a critical test of this interpretation. The new ages of the Izvorovo Pluton are also coupled with petrographic and geochemical data, and integrated with a compilation of published data from the surrounding plutonic suites of the Strandja Zone. Such a data compilation allows us to demonstrate two major magmatic events in the Late Carboniferous and Permian–Triassic. It also allows for better understanding and correlation of the units within the Strandja Zone and across the region.
2. Geological setting
The Strandja (or Strandzha) Zone, also known as the Sakar–Strandja Zone (SSZ; Boyadijev & Lilov Reference Boyadjiev and Lilov1972; Ivanov, Reference Ivanov2017), Strandja (Strandzha) Massif (SM; Okay et al. Reference Okay, Satur, Tüysüz, Akyüz and Chen2001; Natal’in et al. Reference Natal’in, Sunal, Gün, Wang and Zhiqing2016 and references therein) or Istranca Massif (Bedi et al. Reference Bedi, Vasilev, Dabovski, Ergen, Okuyucu, Dogan, Kagan Tekin, Ivanova, Boncheva, Lakova, Sachanski, Kuscu, Tumcay, Gülnur Demiray, Soycan and Cemal Goncuoglu2013), is a NW–SE-trending mountain belt, located in the border area between Bulgaria and Turkey (Fig. 1). To the south, the Strandja Zone is covered by Cenozoic sedimentary rocks of the Thrace Basin. The relationship between the Rhodope Metamorphic Complex to the west and the Strandja Zone is poorly understood, and their contact is mostly covered by Cenozoic sedimentary rocks.
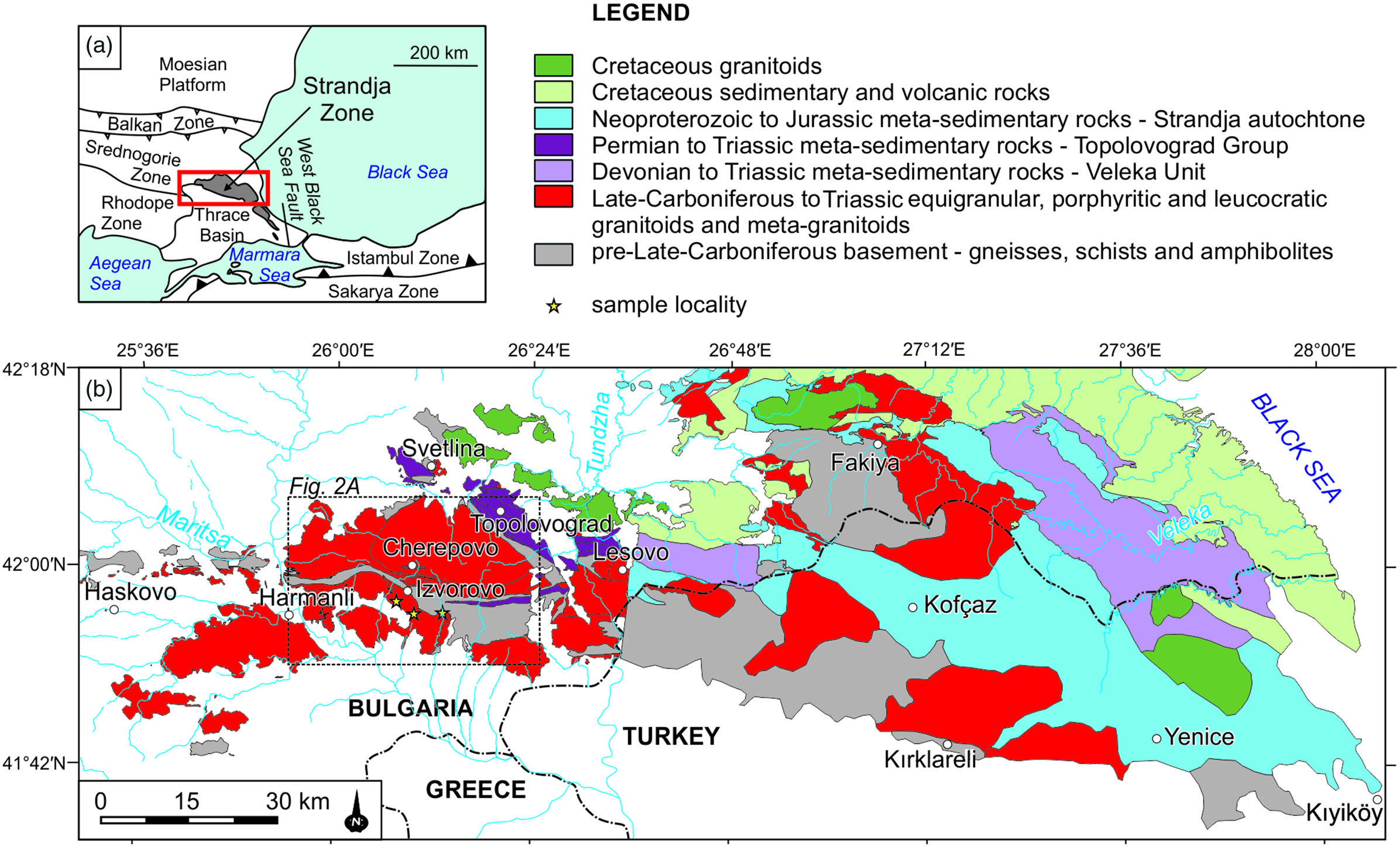
Fig. 1. Maps of the Strandja Zone along the Bulgarian–Turkish border: (a) Strandja Zone and surrounding major tectonic units; (b) geological map of the Strandja Zone with the sample localities (modified after Okay et al. Reference Okay, Satur, Tüysüz, Akyüz and Chen2001; Gerdjikov, Reference Gerdjikov2005; Natal’in et al. Reference Natal’in, Sunal, Gün, Wang and Zhiqing2016).
2.a. Units
In Bulgaria, the Strandja Zone is defined as a pre-Late Cretaceous orogen, that is covered by sedimentary rocks and intruded by plutons related to the formation of Apuseni–Banat–Timok–Sredna Gora Late Cretaceous magmatic arc (Chatalov, Reference Chatalov1990; Gallhofer et al. Reference Gallhofer, Von Quadt, Peytcheva, Schmid and Heinrich2015). A key feature of the Strandja Zone is that the main phase of deformation and metamorphism occurred during the Late Jurassic to Early Cretaceous. This event is known as the Early Alpine Orogeny in Bulgarian literature (i.e. Ivanov et al. Reference Ivanov, Gerdjikov and Kounov2001), and the Cimmerian Orogeny in Turkish literature (i.e. Cattò et al. Reference Cattò, Cavazza, Zattin and Okay2018). Based on their structural position during the Early Alpine Orogeny, the degree of metamorphism and stratigraphic characteristics, three units have been defined in the Bulgarian part of the Strandja Zone: the Sakar, Strandja and Veleka units (Chatalov, Reference Chatalov1990; Gerdjikov, Reference Gerdjikov2005).
The Sakar Unit is exposed within the Sakar Mountains, but also continues westward of the Maritsa River into the Harmanli Block west of Harmanli, as well as the Maritsa region (Fig. 1). The Strandja Unit is located to the east of the Sakar Unit, within the Strandja Mountains and Dervent Heights along the Bulgarian–Turkish border, and further to the southeast in Turkey towards the vicinity of Istanbul. The Veleka Unit is an allochthon of the Zabernovo Nappe (Chatalov, Reference Chatalov1990), that has been emplaced over the less intensely metamorphosed Strandja Unit itself (Gerdjikov, Reference Gerdjikov2005).
2.b. Evolution of the units and the granitoids
The Sakar and Strandja units share a similar geological history (e.g. Chatalov, Reference Chatalov1990). Late Carboniferous to Triassic granitoids and meta-granitoids in both units were intruded into metamorphic basement, which is composed of gneisses, schists and amphibolites. This pre-Late Carboniferous basement includes Neoproterozoic–Cambrian (Natal’in et al. Reference Natal’in, Sunal, Gün, Wang and Zhiqing2016) and Ordovician (Bonev et al. Reference Bonev, Filipov, Raicheva and Moritz2019 a) felsic magmatic rocks, and is considered to represent peri-Gondwanan terrane(s) that formed along the northern Gondwanan margin (e.g. Stampfli, Reference Stampfli, Bozkurt, Winchester and Piper2000; Okay & Topuz, Reference Okay and Topuz2017). Late Carboniferous to Triassic magmatism forms significant parts of the Strandja Zone, with the most important batholiths and plutons shown in Figure 1, along with smaller unnamed intrusions within the basement. One of the largest magmatic bodies in the Sakar Unit is the Late Carboniferous Sakar Batholith (∼450 km2, Kamenov et al. Reference Kamenov, Vergilov, Dabovski, Vergilov and Ivchinova2010; Peytcheva et al. Reference Peytcheva, Georgiev and Von Quadt2016; Bonev et al. Reference Bonev, Filipov, Raicheva and Moritz2019 a; Pristavova et al. Reference Pristavova, Tzankova, Gospodinov and Filipov2019). Neighbouring intrusions include the Late Carboniferous Ustrem Pluton and Melnitsa Complex (Bonev et al. Reference Bonev, Filipov, Raicheva and Moritz2019 a), and the Izvorovo and Levka plutons which are less extensively studied.
The Izvorovo Pluton was defined as a weakly deformed body of equigranular granites, very similar to the intrusive bodies from the Sakar Pluton (Kouzhokharov & Kouzhokharova, Reference Kozhoukharov and Kozhoukharova1973). According to the same authors, these granites, with an assumed Precambrian age, may have been emplaced into the lower part of the metamorphic basement represented by migmatitic paragneisses. Based on detailed field mapping and microfabric data, Ivanov et al. (Reference Ivanov, Gerdjikov and Kounov2001) significantly enlarged the areal extent of the Izvorovo Pluton by including strongly foliated K-feldspar porphyritic and equigranular granites, previously regarded as the local country rock. Between 1999 and 2000, the westernmost part of the Sakar Mountains and the Harmanli Block area was mapped at 1:25 000 scale. It was demonstrated by Jordanov et al. (Reference Jordanov, Sarov, Georgiev, Marinova, Dobrev, Grozdev, Balkanska and Moskovska2008) that this mapped region is almost entirely composed of metamorphosed and deformed granitoids (orthogneisses) of the Izvorovo Pluton in the Sakar area. Due to the extensive Cenozoic cover, the true extent of the Izvorovo Pluton cannot be easily ascertained, but field mapping indicates that the pluton extends westward toward the area south of Haskovo, thus allowing a crude estimate of the areal extent of the pluton at ˜500 km2. Based on these field constraints, the Harmanli Block is regarded as the western part of the Izvorovo Pluton.
In the Sakar Unit, the metamorphic basement and the Late Carboniferous to Triassic granitoids and meta-granitoids are overlain by Permian to Triassic meta-sedimentary rock of the Topolovgrad Group (Fig. 1; e.g. Chatalov, Reference Chatalov1990). All these units are penetratively deformed and metamorphosed during the Late Jurassic to Early Cretaceous Early Alpine Orogeny (Chatalov, Reference Chatalov1988, Reference Chatalov1990; Gerdjikov Reference Gerdjikov2005; Bonev et al. Reference Bonev, Spikings and Moritz2020b; Szopa et al. Reference Szopa, Sałacińska, Gumsley, Chew, Petrov, Gawęda, Zagórska, Deput, Gospodinov and Banasik2020). The highest grades (amphibolite-facies) associated with Early Alpine metamorphism are encountered in the Sakar Mountains (Chatalov, Reference Chatalov1990; Tsankova & Pristavova Reference Tzankova and Pristavova2007 a, b) and in the Harmanli Block (Jordanov et al. Reference Jordanov, Sarov, Georgiev, Marinova, Dobrev, Grozdev, Balkanska and Moskovska2008) of the Sakar Unit. Elsewhere within the Sakar Unit, metamorphism was within the greenschist facies. This spatial distribution of higher peak metamorphic temperatures compared to the rest of the Sakar Unit of the Strandja Zone led Chatalov (Reference Chatalov1990) to propose the existence of the so-called Sakar palaeothermal dome. Subsequently, Ivanov et al. (Reference Ivanov, Gerdjikov and Kounov2001) and Gerdjikov (Reference Gerdjikov2005) argued that these anomalously high temperatures were caused by syn-kinematic Late Jurassic to Early Cretaceous emplacement of large plutonic bodies (i.e. the Sakar, Izvorovo and Varnik plutons). However, new geochronological data have shown that the Sakar Batholith was emplaced in the Late Carboniferous to Early Permian (c. 305 – c. 295 Ma; Peytcheva et al. Reference Peytcheva, Georgiev and Von Quadt2016; Bonev et al. Reference Bonev, Filipov, Raicheva and Moritz2019 a; Pristavova et al. Reference Pristavova, Tzankova, Gospodinov and Filipov2019). Obtaining accurate and precise U–Pb geochronological data for the Izvorovo Pluton is a further test of this hypothesis.
3. Sample localities
The region investigated in the Izvorovo Pluton is located to the south of Izvorovo Village. The Izvorovo Pluton is separated from the Sakar Batholith by an east–west-trending belt of basement rocks (Fig. 2a). The Izvorovo Pluton consists of foliated porphyritic and equigranular meta-granites and porphyroclastic gneisses referred to as the Lesovo Orthometamorphic Complex (Kamenov et al. Reference Kamenov, Vergilov, Dabovski, Vergilov and Ivchinova2010) or described as the ‘Sakar-type’ schistose granites of the Izvorovo Dome (Dimitrov Reference Dimitrov1956, Reference Dimitrov1959; Boyanov et al. Reference Boyanov, Kozhoukharov and Savov1965; Ivanov et al. Reference Ivanov, Gerdjikov and Kounov2001). The absolute age of the Izvorovo Pluton has not been determined by isotopic dating, and there is also a lack of any other geochemical and mineralogical data from this pluton. A recent study by Bonev et al. (Reference Bonev, Filipov, Raicheva and Moritz2019 a) regarded the Izvorovo Pluton as a part of the Carboniferous Lesovo Complex (gneisses and granites), with an age of c. 306 Ma, based on U–Pb zircon dating of meta-quartz–diorite collected from the Ustrem Pluton southeast of Lesovo Village.
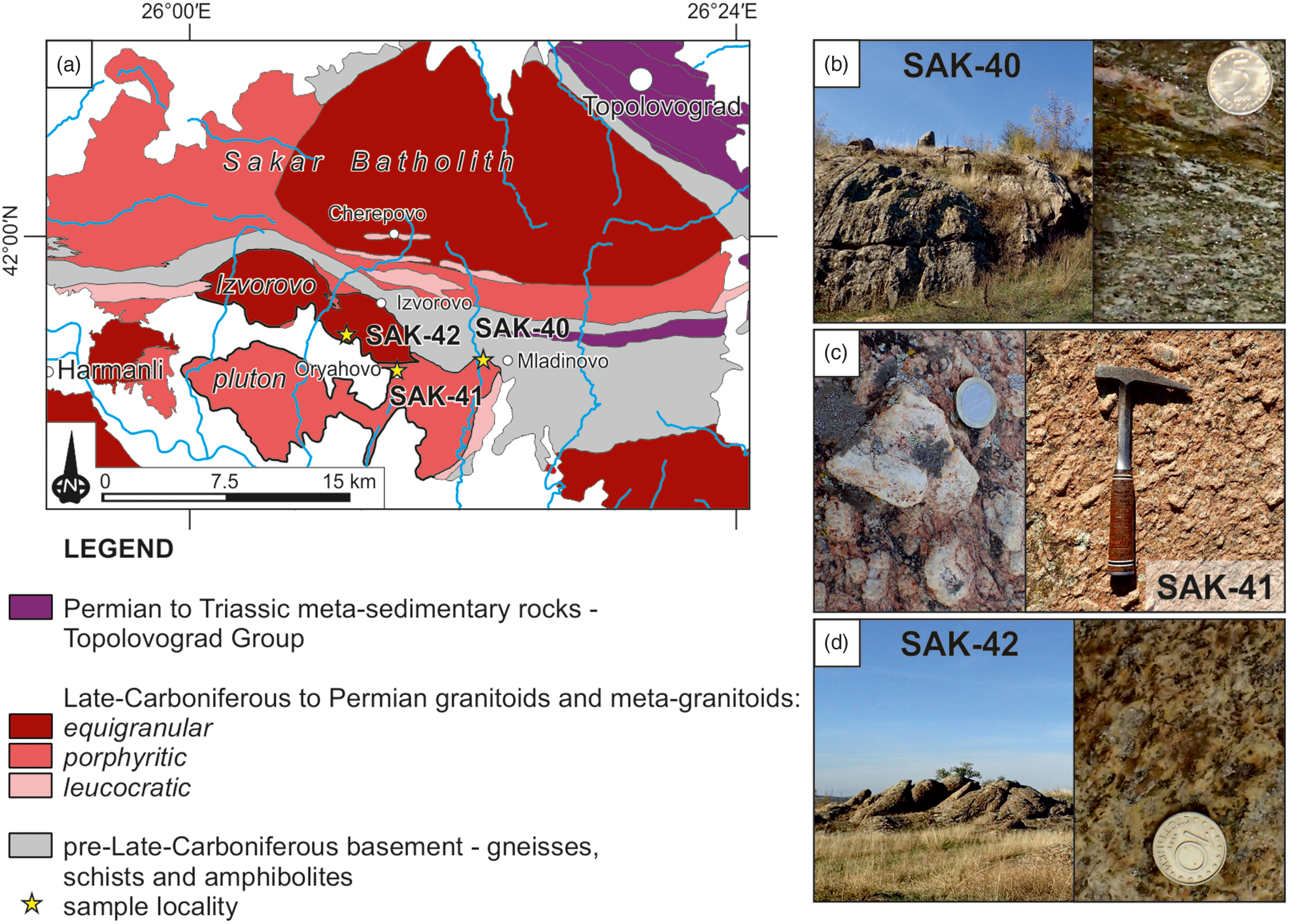
Fig. 2. Sample localities in the Izvorovo Pluton: (a) geological map of the Sakar Unit (modified after Okay et al. Reference Okay, Satur, Tüysüz, Akyüz and Chen2001; Gerdjikov, Reference Gerdjikov2005; Natal’in et al. Reference Natal’in, Sunal, Gün, Wang and Zhiqing2016); (b) strongly deformed orthogneiss with feldspar porhyroblasts (sample SAK-40), from the west of Mladinovo; (c) augen gneiss from which sample SAK-41 was collected from Oryahovo; (d) meta-granite (sample SAK-42) from southwest of Izvorovo.
In this study, three samples of orthogneiss (SAK-40), augen gneiss (SAK-41) and weakly foliated granite (SAK-42) were collected from the Izvorovo Pluton (Fig. 2). All of these samples represent different types of the Izvorovo meta-granites. The weakly foliated equigranular meta-granite sample SAK-42 is one of the variations of the Izvorovo Pluton, regarded as the ‘Lesovo-type’ pre-metamorphic granite (Kozhoukharov & Kozhoukharova, Reference Kozhoukharov and Kozhoukharova1973). The orthogneiss sample SAK-40 and augen gneiss sample SAK-41 are other types of porphyritic meta-granite within the Izvorovo Pluton. However, our field observations showed that significant strain variations occur between the massive, weakly foliated sample SAK-42 and gneiss samples SAK-40 and SAK-41, as the latter are strongly sheared due to their proximity to the country-rock contact.
Sample locations, rock types and mineral assemblages are listed in Table 1. The sample of orthogneiss with feldspar porphyroclasts (SAK-40) was collected from outcrop in a river valley c. 1 km to the west of Mladinovo (Fig. 2a, b). The contact between orthogneiss and deformed amphibolite with leucosome layers (basement rock) is observed in the vicinity of this sample locality (˜200 m to the north). The orthogneiss with feldspar porphyroclasts shows a strong resemblance to porphyritic granitoids from the Sakar Batholith, but is more deformed. The augen gneiss sample (SAK-41) was collected from a large outcrop (˜3000 m2) of pinkish felsic gneisses in the northern part of Oryahovo Village. This rock contains feldspar porphyroclasts up to 3–4 cm in length (Fig. 2a, c). Samples of meta-granite (SAK-42) were collected from an outcrop in close proximity to a dam located c. 2 km to the southwest of Izvorovo itself. This meta-granite is equigranular and coarse-grained (Fig. 2a, d). As the Sakar Mountains study area is covered by steppe, the rock outcrops are usually strongly weathered outside of quarries. However, the freshest rock samples were collected for this study.
Table 1. Sample localities and modal mineral assemblages

Major (•••), minor (••) and accessory (•) amounts of minerals. Qz, quartz; Pl, plagioclase; Afs, alkali feldspar; Bt, biotite; Ms, muscovite; Zrn, zircon; Ap, apatite; Ttn, titanite; Mnz, monazite; Fe-ox, Fe-oxides; Mag, magnetite; Ilm, ilmenite.
4. Analytical procedures
4.a. Sample preparation
Three rock samples (SAK-40, SAK-41, SAK-42) were analysed in this study. Thin-sections were made from each sample and then petrographic observations in transmitted and reflected light were made using an Olympus BX-51 optical polarizing microscope at the Institute of Earth Sciences, University of Silesia in Katowice, Poland. This was followed by scanning electron microscopy using back-scattered electron (BSE) imagery coupled to an energy-dispersive spectrometry (EDS) using a ThermoFisher Scientific Phenom XL Scanning Electron Microscope (SEM).
For whole-rock geochemistry, all weathered material was removed from the samples, and the most homogeneous parts were selected. The samples were then crushed using a jaw crusher before being pulverized in an agate ball mill to a powder. A representative aliquot was then despatched for analysis of major and trace elements at the Bureau Veritas Analytical Laboratories in Vancouver, Canada, after being coned and quartered. The lithogeochemical package selected included X-ray fluorescence (XRF) spectrometry for major elements and solution ICP-MS for trace elements, including rare earth elements (REE). Loss on ignition (LOI) on each sample was determined to obtain the volatile content. The resultant geochemical data were then plotted using the GeoChemical Data toolkit (GCDkit) of Janoušek et al. (Reference Janoušek, Moyen, Martin, Erban and Farrow2016).
Separation of zircon was undertaken at the Sample Preparation Laboratory, Institute of Geological Sciences, Polish Academy of Sciences, Kraków, Poland. These separations were made using conventional density separation techniques (i.e. crushing, sieving through a 0.315 mm mesh, washing and panning). Using a standard binocular microscope, zircon was then isolated from other accessory heavy minerals and placed into epoxy resin mounts of 25 mm diameter. The mounts were subsequently ground and the zircon grains polished to half thickness to expose their cores. Prior to U–Pb isotopic analyses, all zircon grains were imaged in transmitted and reflected light microscopy using the same Olympus BX-51 microscope, followed by scanning electron microscopy to reveal their internal textures for spot selection. Scanning electron microscopy was also undertaken with a Thermo Fischer Scientific Phenom XL SEM for BSE imagery of the zircon grains. Additionally, the zircon grains in the epoxy mounts were imaged by cathodoluminescence (CL) on a FET Phillips 30 SEM using a 15 kV accelerating voltage and a beam current of 1 nm to highlight the internal structures of the zircon grains. Prior to CL imaging, the epoxy mounts were carbon-coated. All of the respective petrographic imaging was made at the Institute of Earth Sciences, University of Silesia in Katowice. The carbon coating was then removed and the epoxy mounts were cleaned in an ultrasonic bath before U–Th–Pb isotopic measurements were undertaken.
4.b. Sample analysis
Samples SAK-40, SAK-41 and SAK-42 then underwent U–Th–Pb isotopic analyses at the Department of Geology, Trinity College Dublin, Ireland, using a Photon Machines Analyte Excite 193 nm ArF excimer laser-ablation system with a HelEx 2-volume ablation cell, which was coupled to an Agilent 7900 mass spectrometer. The NIST612 standard glass was used to tune the instruments to obtain a Th/U of unity, and produce low oxide production rates (i.e. ThO+/Th+ typically <0.15 %). A circular laser spot of 24 μm was used, with a repetition rate of 11 Hz. Helium carrier gas was fed into the laser cell at ˜0.4 L min−1, and was mixed with an aerosol of ˜0.6 L min−1 Ar make-up gas and 11 mL min−1 N2. Eight isotopes (90Zr, 202Hg, 204Pb, 206Pb, 207Pb, 208Pb, 232Th and 238U) were measured during each analysis. These analyses comprised 27.3 s of ablation (300 shots) and 12 s of washout time. The latter portions of the washout time were used for baseline measurements. The ‘VizualAge’ data reduction scheme (Petrus & Kamber, Reference Petrus and Kamber2012) in the freeware IOLITE package (Paton et al. Reference Paton, Hellstrom, Paul, Woodhead and Hergt2011) was used to undertake data reduction of the raw U–Th–Pb isotopic data. Downhole fractionation corrections to the raw data were then made to account for the long-term drift in isotopic or elemental ratios by normalizing all ratios to those of the U–Th–Pb reference materials. Conventional sample-standard bracketing was then applied. The 91500 zircon (206Pb–238U age of 1065.4 ± 0.6 Ma; Wiedenbeck et al. Reference Wiedenbeck, Alle, Corfu, Griffin, Meier, Oberli, Von Quadt, Roddick and Spiegel1995, Reference Wiedenbeck, Hanchar, Peck, Sylvester, Valley, Whitehouse, Kronz, Morishita, Nasdala, Fiebig, Franchi, Girard, Greenwood, Hinton, Kita, Mason, Norman, Ogasawara, Piccoli, Rhede, Satoh, Schulz-Dobrick, Skår, Spicuzza, Terada, Tindle, Togashi, Vennemann, Xie and Zheng2004) was used as the primary U–Pb calibration standard. The Plešovice zircon (206Pb–238U age of 337.13 ± 0.37 Ma; Sláma et al. Reference Sláma, Košler, Condon, Crowley, Gerdes, Hanchar, Horstwood, Morris, Nasdala and Norberg2008) and WRS 1348 zircon (206Pb–238U age of 526.26 ± 0.70; Pointon et al. Reference Pointon, Cliff and Chew2012) were used as secondary standards, and yielded respective ages of 336.9 ± 2.4 Ma (206Pb–238U weighted mean age, n = 13) and 526.6 ± 3.7 Ma (206Pb–238U weighted mean age, n = 17).
Following isotopic analysis, all analysed zircon grains in the epoxy mounts were again imaged by BSE, and subsequently interpreted using the pre-analysis BSE and CL images to verify any inadvertent mixtures of growth zones in the laser ablation sampling and measurements. The final geochronological calculations, Concordia diagrams as well as weighted mean diagrams were made using the Isoplot 3.75 macro for Microsoft Excel (Ludwig, Reference Ludwig2012). In Table S1 (in the Supplementary Material available online at https://doi.org/10.1017/S0016756821000650), the data are provided at the 2σ analytical uncertainty level. Weighted mean calculations and discordia intercept ages are also given at the 2σ levels and include decay constant errors. Data with absolute discordance of <5 % (206Pb/238U vs 205Pb/237U) were treated as concordant data.
5. Results
5.a. Petrographic observations and whole-rock geochemistry
Sample SAK-40 is the most deformed rock investigated in this study. It was sampled from the area located closest to the orthogneiss – country-rock contact, with the country rock composed of amphibolites and biotite paragneisses. Sample SAK-40 represents a foliated porphyroclastic gneiss (Fig. 2b), which contains quartz, plagioclase, K-feldspar and two micas (muscovite and biotite), with accessory magnetite, as well as various Fe-oxides, zircon and fluoroapatite (Fig. 3a, b). The gneiss has a well-defined foliation, composed of alternating layers of dark minerals (biotite, magnetite and Fe-oxides) and light minerals, mostly quartz and feldspars (Fig. 3a). The deformed porphyroclasts are composed of K-feldspar, are elongated and irregular in shape and are surrounded by fine-grained recrystallized quartz with an undulose extinction.

Fig. 3. Petrographic images of the gneisses and meta-granite from the Izvorovo Pluton: (a, b) orthogneiss sample SAK-40, (a) thin-section in plane-polarized light (PPL) showing foliation within the orthogneiss, (b) mineral assemblages in the BSE images; (c, d) augen gneiss sample SAK-41, (c) BSE image showing two-mica intergrowths surrounding accessory titanite, (d) thin-section image showing microcline twinning of K-feldspar porphyroclasts (under cross-polarized light); (e, f) meta-granite sample SAK-42, (e) PPL thin-section image showing the coarse-grained textures of the meta-granite, (f) BSE image showing the diverse mineral assemblage of the dark patches surrounded by coarse-grained K-feldspar and quartz. Qz, quartz; Pl, plagioclase; Afs, alkali feldspar; Bt, biotite; Ms, muscovite, Zrn, zircon; Ap, apatite; Ttn, titanite; Mnz, monazite.
Sample SAK-41 (Fig. 2c) is an augen gneiss that contains large porphyroclasts of K-feldspar within a quartz, plagioclase and biotite–muscovite matrix, with accessory fluoroapatite, zircon, titanite, monazite and ilmenite (Fig. 3c, d). The K-feldspar has characteristic microcline twinning (Fig. 3d) and is up to 3–4 cm in length. Titanite occurs within nests of co-genetic intergrowths of the two micas (Fig. 3c). The K-feldspar porphyroclasts are interpreted as phenocrysts inherited from the igneous protolith.
Sample SAK-42 is a coarse-grained, equigranular and weakly foliated meta-granite (Fig. 2d), which consists of plagioclase, quartz, K-feldspar, biotite and muscovite with accessory fluoroapatite, zircon, monazite and Fe-oxides (Fig. 3e, f). Accessory minerals occur mostly within randomly distributed, irregular patches of two-mica intergrowths, surrounded by feldspars and quartz. This meta-granite is the least deformed rock sample collected in this study.
Whole-rock major- and trace-element compositions for three samples (SAK-40, SAK-41, SAK-42), and a compilation of the published data from other Late Carboniferous to Early Triassic plutons in the Strandja Zone are presented in Table S2 (in the Supplementary Material available online at https://doi.org/10.1017/S0016756821000650). The samples from Izvorovo Pluton are silica-rich (70.4–75.4 wt % SiO2) meta-granitoids and orthogneisses, which plot in the granite field on a SiO2 vs Na2O + K2O diagram (Fig. 4a; Middlemost, Reference Middlemost1994), and are peraluminous (Fig. 4b; Shand, Reference Shand1943). The trace elements were normalized to the ocean-ridge granite (ORG) values of Pearce et al. (Reference Pearce, Harris and Tindle1984). Samples from the Izvorovo Pluton have similar trace element profiles, characterized by enrichments in K, Rb, Ba, Th and Ce and Sm relative to Ta, Nb, Hf, Zr, Y and Yb (Fig. 4c). These patterns show a resemblance to patterns from the Permian Kırklareli Pluton and Late Carboniferous to Early Permian Sakar Batholith; however, samples from Kırklareli Pluton have stronger negative Ta–Nb anomalies. The rare earth elements (REEs) were normalized to chondrite values of McDonough & Sun (Reference McDonough and Sun1995; Fig. 4b). The samples show fractionation of the light REE (LREE) relative to heavy REE (HREE), and have negative Eu anomalies (0.31–0.35). These patterns are within the range of samples from Sakar Batholith and Kırklareli Pluton.

Fig. 4. Major and trace elements diagrams for meta-granitoids and gneisses from the Strandja Zone: (a) total alkali vs silica (TAS) diagram (Middlemost, Reference Middlemost1994); (b) A/CNK vs A/NK plot of Shand (Reference Shand1943); (c) ocean ridge granite (ORG)-normalized diagram with normalization values after Pearce et al. (Reference Pearce, Harris and Tindle1984); (d) chondrite-normalized REE diagram with normalization values from McDonough & Sun (Reference McDonough and Sun1995). Data from previous studies are from: Sunal et al. (Reference Sunal, Natal’in, Satir and Toraman2006), Kamenov et al. (Reference Kamenov, Vergilov, Dabovski, Vergilov and Ivchinova2010), Machev et al. (Reference Machev, Ganev and Klain2015), Aysal et al. (Reference Aysal, Şahin, Güngör, Peytcheva and Öngen2018), Bonev et al. (Reference Bonev, Filipov, Raicheva and Moritz2019 a), and are given in Table S2 (in the Supplementary Material available online at https://doi.org/10.1017/S0016756821000650).
5.b. U–Pb zircon dating of Izvorovo meta-granitoids
LA-ICP-MS U–Th–Pb isotopic data of the analysed zircons for the samples SAK-40, SAK-41 and SAK-42 are listed in Table S1, in the Supplementary Material available online at https://doi.org/10.1017/S0016756821000650. Dates are reported as 206Pb–238U ages.
Orthogneiss sample SAK-40 from west of Mladinovo contains oscillatory zoned zircons that are euhedral to subhedral. Zircons are up to 180 µm in length with aspect ratios of 1:1 and 1:2 (Fig. 5a). Some of the zircons have thin rims (up to 8 µm in width), which were not analysed during this study. Thirty-one analyses were made on 30 zircon grains, from which 28 analyses are concordant (<5 % disc.; Table S1, in the Supplementary Material available online at https://doi.org/10.1017/S0016756821000650; Fig. 6a). From all the concordant data, one analysis is identified as of being a mixture of rim and oscillatory zoned zircon core in BSE and CL post-imaging, and is therefore excluded from further consideration in this study. The remaining data range from c. 248 Ma to c. 440 Ma, with a significant cluster on concordia between c. 248 Ma and c. 262 Ma (Fig. 6a). This cluster of 25 analyses defines a weighted mean 206Pb–238U age of 254 ± 2 Ma (MSWD = 1.5; Fig. 6b). This age is interpreted as the crystallization age of the magmatic protolith. Two older analyses come from zircon cores (Fig. 5a) and are interpreted as inherited components.

Fig. 5. BSE and CL images of representative zircons from: (a) sample SAK-40; (b) sample SAK-41; (c) sample SAK-42. Numbers represent the grain number, reported values are 206Pb/238U ages, all presented ages are concordant (<5 % disc.) except for one discordant analysis (denoted d, which is disc. >5 %), and the white circles represent the laser ablation spot.
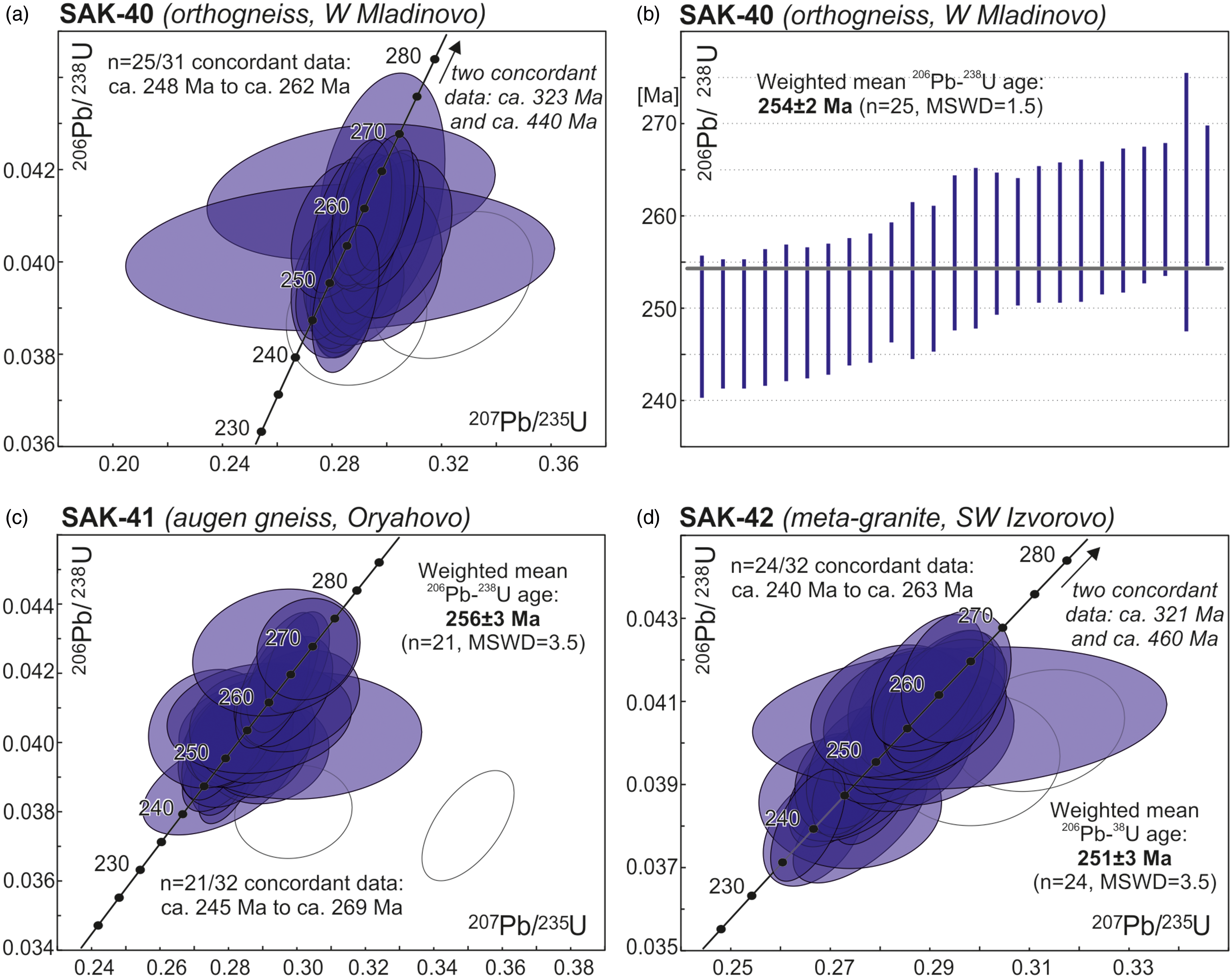
Fig. 6. LA-ICP-MS zircon U–Th–Pb analyses for sample: (a, b) SAK-40 (orthogneiss); (c) sample SAK-41 (augen gneiss); (d) sample SAK-42 (meta-granite); Wetherill Concordia diagrams (after Wetherill, Reference Wetherill1956) are shown in (a), (b) and (d); the data shown as filled ellipses are used for age calculations, and black ellipses are discordant data (>5 %); analyses of mixed domains are not shown. All data-point error ellipses and error bars are at the 2σ uncertainty level.
Augen gneiss sample SAK-41 from Oryahovo contains zircons that are elongated and euhedral to subhedral. Zircons are up to 220 µm in length, with aspect ratios of 1:1 to 4:1 (Fig. 5b). All zircons have strong oscillatory zonation. Some of the grains contain thin rims (˜5 to ˜10 µm in width), which were not analysed during this study. A total of 32 analyses were obtained from 27 grains (Table S1, in the Supplementary Material available online at https://doi.org/10.1017/S0016756821000650; Fig. 6c), of which 30 analyses were concordant (<5 % disc.). From all the concordant analyses, nine analyses were excluded from further consideration because they were obtained from mixed domains as revealed by BSE and CL post-analysis imagery. Of the remaining data, 21 data points from 19 grains with concordant 206Pb–238U ages ranging from c. 251 Ma to c. 269 Ma define a weighted mean 206Pb–238U age of 256 ± 3 Ma (MSWD = 3.5). Although these data scatter beyond analytical uncertainty more than the cluster of data points in sample SAK-40, the calculated ages are within analytical uncertainty between the two samples. Thus, c. 256 Ma is interpreted as the emplacement age of the gneiss protolith.
Meta-granite sample SAK-42 from southwest of Izvorovo contains mostly subhedral, and stubby to elongate zircons (up to 240 µm in length, with aspect ratios of 1:1 to 3:1; Fig. 5c), and exhibiting oscillatory zoning. Some of the zircons have thin rims (up to 8 µm in width), which were not analysed during this study. Thirty-two analyses from 30 grains were obtained (Table S1, in the Supplementary Material available online at https://doi.org/10.1017/S0016756821000650; Fig. 6d), of which two data points were discordant (>5 % disc.), and four were identified as mixed zones in post-analytical BSE and CL imaging, and were therefore excluded from further consideration. The remaining 26 data points range from c. 240 Ma to c. 460 Ma, of which 24 analyses scatter along concordia between c. 240 Ma and c. 263 Ma, with a weighted mean 206Pb–238U age of 251 ± 3 Ma (MSWD = 3.5). This age is within analytical error of the crystallization age of the orthogneiss igneous protolith (SAK-40), and is interpreted as the crystallization age of the meta-granite. The two older analyses come from zircon cores and are interpreted as inherited components.
6. Discussion
6.a. Interpretation of geochronology and its regional context
All three samples from the Izvorovo Pluton yielded similar U–Pb zircon ages, ranging between 251 ± 3 Ma and 256 ± 3 Ma, with a weighted mean 206Pb/238U age of 254 ± 5 Ma. The time interval between 251 ± 3 Ma and 256 ± 3 Ma is considered as the crystallization age of the Izvorovo Pluton. This shows that both varieties of equigranular and porphyritic meta-granite from the Izvorovo Pluton, which used to be regarded as two different units of meta-granite and migmatite (Kozhoukharov & Kozhoukharova, Reference Kozhoukharov and Kozhoukharova1973), are coeval. Within the zircon populations of the samples, xenocrysts were detected. Some inherited cores were analysed, and yielded concordant ages at 460–440 Ma and c. 320 Ma (Table S1, in the Supplementary Material available online at https://doi.org/10.1017/S0016756821000650). A 460–440 Ma magmatic episode within the Sakar Unit was recently described by Bonev et al. (Reference Bonev, Filipov, Raicheva and Moritz2019 a), who dated leucocratic stock meta-granites exposed to the east of the Sakar Batholith at 462 ± 3 Ma. The younger population of zircon xenocrysts is consistent with 320–310 Ma ages obtained from zircons commonly found within the Sakar Batholith (Bonev et al. Reference Bonev, Filipov, Raicheva and Moritz2019 a; Pristavova et al. Reference Pristavova, Tzankova, Gospodinov and Filipov2019). The 320–310 Ma zircons documented from the Sakar Batholith are interpreted by Bonev et al. (Reference Bonev, Filipov, Raicheva and Moritz2019 a) and Pristavova et al. (Reference Pristavova, Tzankova, Gospodinov and Filipov2019) as ‘antecrysts’, i.e. a group of crystals from an earlier pulse of magma which were incorporated by a later pulse. This suggests the occurrence of at least one earlier magmatic pulse in the Late Carboniferous within the Sakar Unit.
The crystallization age of the Izvorovo Pluton post-dates the emplacement of the Sakar Batholith (305–295 Ma; Peytcheva et al. Reference Peytcheva, Georgiev and Von Quadt2016; Bonev et al. Reference Bonev, Filipov, Raicheva and Moritz2019 a; Pristavova et al. Reference Pristavova, Tzankova, Gospodinov and Filipov2019). Additionally, the Izvorovo Pluton underwent a later Late Jurassic to Early Cretaceous deformation and metamorphic event (Chatalov, Reference Chatalov1990; Bonev et al., Reference Bonev, Spikings and Moritz2020 b), which is clearly visible in outcrop and in the petrographic data obtained from samples SAK-40 and SAK-41. The presented geochronological data invalidate the interpretation of Ivanov et al. (Reference Ivanov, Gerdjikov and Kounov2001) and Gerdjikov (Reference Gerdjikov2005) that syn-kinematic magmatism (of assumed Late Jurassic to Early Cretaceous age) was the heat source for Early Alpine metamorphism. Thus, the question about the origin of the ‘anomalous’ high-grade metamorphism in the Sakar Mountains relative to the adjacent units remains open.
The Izvorovo Pluton is the first Permian–Triassic plutonic body described within the Sakar Unit, and is clearly emplaced 40 Myr later than Late Carboniferous – Early Permian intrusives such as the Sakar Batholith and coeval intrusions (c. 305 – c. 295 Ma; Peytcheva et al. Reference Peytcheva, Georgiev and Von Quadt2016; Bonev et al. Reference Bonev, Filipov, Raicheva and Moritz2019 a; Pristavova et al. Reference Pristavova, Tzankova, Gospodinov and Filipov2019; Fig. 7; Table S3 in the Supplementary Material available online at https://doi.org/10.1017/S0016756821000650). Recently, new U–Pb ages ranging between 245 and 230 Ma were presented for the Harmanli Block Magmatic Complex (Bonev et al. Reference Bonev, Filipov, Raicheva and Moritz2019 b) and St Iliya Heights (near Svetlina; Bonev et al. Reference Bonev, Filipov, Raicheva and Moritz2020 a), located in the southwestern and northern part of the Sakar Unit (Fig. 2a), respectively. This shows that the magmatic evolution of the region is more complex than previously envisaged.
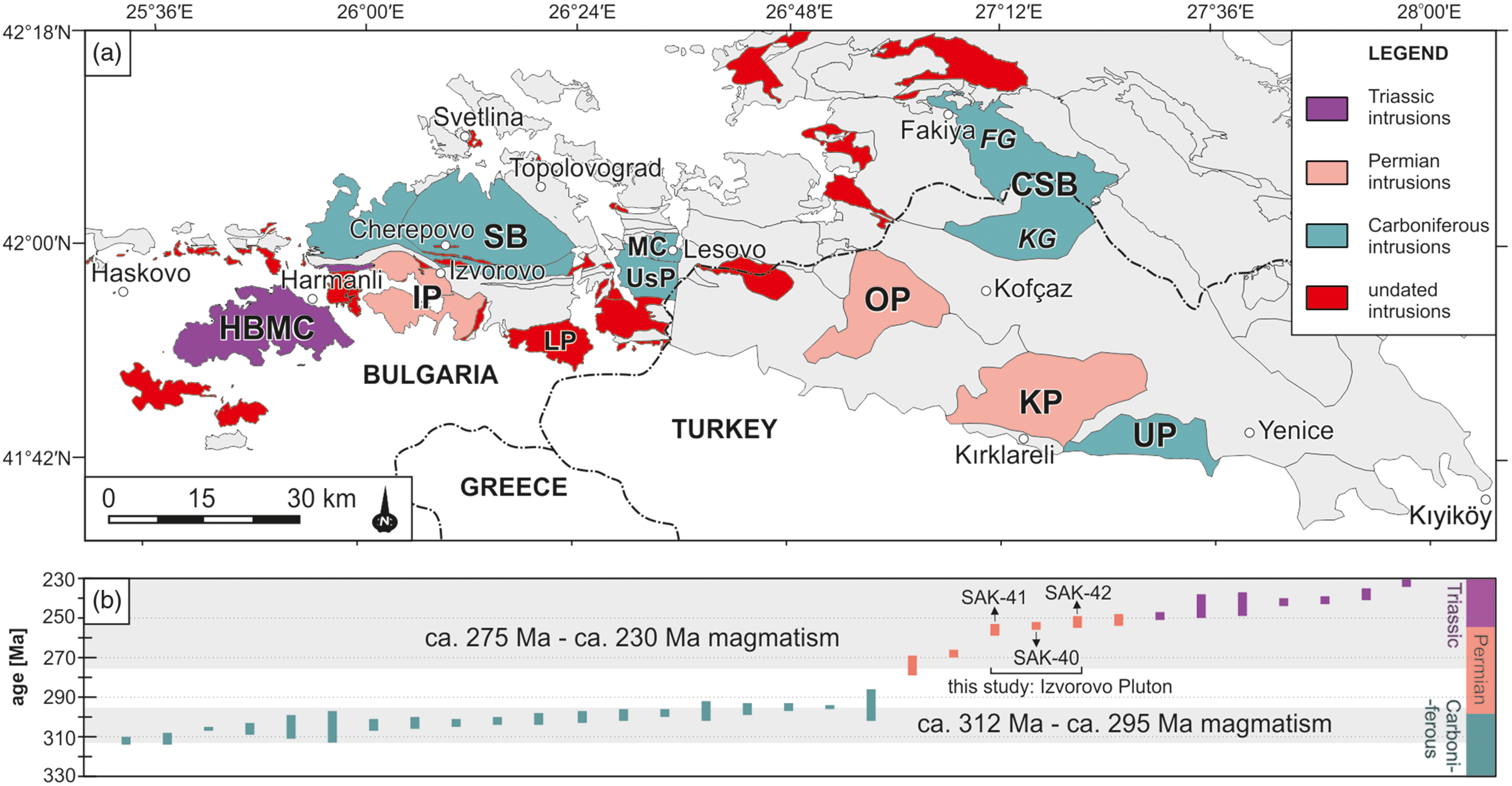
Fig. 7. Spatial and temporal distribution of the Late Carboniferous to Triassic magmatic rocks in the Strandja Zone: (a) geological map showing classification of plutonic bodies according to their age of emplacement (HBMC = Harmanli Block Magmatic Complex, IP = Izvorovo Pluton, SB = Sakar Batholith, LP = Levka Pluton, MC = Melnitsa Complex, UsP = Ustrem Pluton, OP = Ömeroba Pluton, KP = Kırklareli Pluton, UP = Üsküp Pluton, CSB = Central Strandja Batholith consisting of FG = Fakiya granite and KG = Kula granite); (b) summary of U–Pb zircon age determinations from all dated plutonic bodies within the Strandja Zone. Data sources are given in Table S3 (in the Supplementary Material available online at https://doi.org/10.1017/S0016756821000650).
Although the Izvorovo Pluton provides the first evidence of Late Permian magmatism within the Sakar Unit, other Permian and Early Triassic plutons are known from the eastern part of the Strandja Zone. These plutons include the c. 268 Ma Kırklareli Pluton (Aysal et al. Reference Aysal, Şahin, Güngör, Peytcheva and Öngen2018), the c. 252 Ma Ömeroba Pluton (Natal’in et al. Reference Natal’in, Sunal, Gün, Wang and Zhiqing2016; Fig. 7), and the c. 249 Ma Tepecik Pluton, which is located near Istanbul in the southeastern Strandja Zone (Aysal et al. Reference Aysal, Şahin, Güngör, Peytcheva and Öngen2018). For more detailed information about the published U–Pb zircon ages from Late Carboniferous to Triassic magmatic rocks in the Strandja Zone, see Table S3 in the Supplementary Material available online at https://doi.org/10.1017/S0016756821000650. Additionally, there are other published Pb–Pb ages for felsic bodies within the Strandja Unit which were obtained by the single-zircon stepwise evaporation method (Table S3, in the Supplementary Material available online at https://doi.org/10.1017/S0016756821000650; Okay et al. Reference Okay, Satur, Tüysüz, Akyüz and Chen2001; Sunal et al. Reference Sunal, Natal’in, Satir and Toraman2006); however, the inability to evaluate various factors (e.g. discordance) in such data means these ages will not be discussed further in this study.
6.b. Geochronological and geochemical arguments for two-stage magmatism in the Strandja Zone
Felsic to intermediate intrusive magmatism has played an important role in the crustal evolution of the Strandja Zone, as shown in Figure 7. The new geochronological data from this study and from the literature show that extensive magmatism began in the Late Carboniferous, and is almost continuous until the Triassic in both key components of the Strandja Zone, i.e. the Sakar and Strandja units (Table S3, in the Supplementary Material available online at https://doi.org/10.1017/S0016756821000650). The tectonic setting of the Strandja Zone is controversial and depends on the interpreted location of the zone with respect to (1) Variscan collision and (2) the subduction of the Palaeo-Tethys Ocean beneath Laurussia. The first scenario invokes Variscan collision between Gondwana and Laurussia in the granitoid formation (post-collisional granites; post-COLG) in the Strandja Zone. The peri-Gondwana continental magmatic arc, which consisted of the Balkanides, Strandja, Sakarya and Caucasus (BASSAC Block), collided with the Moesia and Istanbul Zone in the Middle Carboniferous (MOIS Block; Okay & Topuz, Reference Okay and Topuz2017). Within the Strandja Zone, Variscan metamorphism is observed in the pre-Late Carboniferous basement, which was affected by both Variscan and Early Alpine metamorphism (e.g. Okay et al. Reference Okay, Satur, Tüysüz, Akyüz and Chen2001). The exact age of Variscan metamorphism in the Strandja Zone remains unknown, but it is well established from the Balkanides (c. 340 – 330 Ma; Carrigan et al. Reference Carrigan, Mukasa, Haydoutov and Kolcheva2006; Antić et al. Reference Antić, Peytcheva, Von Quadt, Kounov, Trivić, Serafimovski, Tasev, Gerdjikov and Wetzel2016; Balkanska et al. Reference Balkanska, Gerdjikov, Georfiev, Lazarova, Dörr and Kounov2021). The second scenario assumes that subduction of the Palaeo-Tethys Ocean beneath Laurussia played a primary role, resulting in various intrusions of volcanic-arc granites (VAG) in the Strandja Zone (Okay et al. Reference Okay, Satur, Tüysüz, Akyüz and Chen2001; Natal’in et al. Reference Natal’in, Sunal, Gün, Wang and Zhiqing2016; Peytcheva et al. Reference Peytcheva, Georgiev and Von Quadt2016; Aysal et al. Reference Aysal, Şahin, Güngör, Peytcheva and Öngen2018; Bonev et al. Reference Bonev, Filipov, Raicheva and Moritz2019 a). This scenario is presented by Aysal et al. (Reference Aysal, Şahin, Güngör, Peytcheva and Öngen2018), who suggested subduction-related magmatism with a transition from Permian arc magmatism to Middle Permian – Early Triassic back-arc magmatism. Rift-related felsic magmatism has also been proposed by some authors (i.e. Okay & Nikishin, Reference Okay and Nikishin2015) for the surrounding regions (e.g. the Western Pontides). Rifting along a continental margin may be associated with back-arc basin development. The sedimentary rocks of the TopoIovgrad Group in the Sakar Unit (Chatalov, Reference Chatalov1990; Zagorchev et al. Reference Zagorchev, Dabovski and Nikolov2009) could have been deposited in this basin, which potentially initiated as a back-arc basin located in the northern part of the zone during the Permian–Triassic.
Geochemically, the Late Carboniferous to Early Triassic granitoids of the Strandja Zone are typical of either calc-alkaline volcanic-arc or post-collisional granites. The meta-granitoids from the Strandja Zone (Fig. 8a) plot within the post-collisional granite (post-COLG) field, which overlaps with the volcanic-arc granite (VAG) field on the Rb vs Y + Nb diagram (Pearce, Reference Pearce1996, after Pearce et al. Reference Pearce, Harris and Tindle1984). The Rb–Hf–Ta ternary diagram (Harris et al. Reference Harris, Pearce, Tindle, Coward and Ries1986; Fig. 8b) indicates that the Late Carboniferous to Early Permian Sakar Batholith and Late Permian Izvorovo Pluton plot within the collisional granite field, whereas data from the Permian Kırklareli Pluton, and most of the data from the Early Triassic Tepecik Pluton, lie within the VAG field. However, as was shown by Harris et al. (Reference Harris, Pearce, Tindle, Coward and Ries1986), not all post-COLG magmas demonstrate the degree of Ta enrichment defined by the boundaries in Figure 8b. The geochemical data of the Late Carboniferous to Early Triassic meta-granitoids of the Strandja Zone also do not show a systematic trend in the temporal evolution of the magma chemistry, or in the tectonic setting inferred from those geochemical data. However, this interpretation is only based on empirical assumptions from geochemical diagrams, and can additionally be disturbed by Rb mobilization due to weathering or metamorphism which affected the granitoids.

Fig. 8. Diagrams for discriminating the tectonic settings of granitic rocks: (a) the Rb–(Y + Nb) diagram (after Pearce, Reference Pearce1996, modified from Pearce et al. Reference Pearce, Harris and Tindle1984); (b) the Rb–Hf–Ta ternary diagram (after Harris et al. Reference Harris, Pearce, Tindle, Coward and Ries1986, modified from Pearce et al. Reference Pearce, Harris and Tindle1984) distinguishing volcanic-arc granite (VAG), within-plate granite (WPG), syn-collisional granite (syn-COLG) and post-collisional granite (post-COLG). Data from previous studies are from Sunal et al. (Reference Sunal, Natal’in, Satir and Toraman2006), Kamenov et al. (Reference Kamenov, Vergilov, Dabovski, Vergilov and Ivchinova2010), Machev et al. (Reference Machev, Ganev and Klain2015), Aysal et al. (Reference Aysal, Şahin, Güngör, Peytcheva and Öngen2018), Bonev et al. (Reference Bonev, Filipov, Raicheva and Moritz2019 a) and are given in Table S2 (in the Supplementary Material available online at https://doi.org/10.1017/S0016756821000650).
The Late Carboniferous to Triassic magmatism is assigned by many authors as related to the subduction of the Palaeo-Tethys Ocean beneath the southern margin of Laurussia (Sunal et al. Reference Sunal, Natal’in, Satir and Toraman2006; Natal’in et al. Reference Natal’in, Sunal, Gün, Wang and Zhiqing2016; Aysal et al. Reference Aysal, Şahin, Güngör, Peytcheva and Öngen2018; Bonev et al. Reference Bonev, Filipov, Raicheva and Moritz2019 a) with further magmatism in a back-arc setting in the Middle Triassic (Aysal et al. Reference Aysal, Şahin, Güngör, Peytcheva and Öngen2018). This interpretation is supported by published geochronological data which grouped all the intrusions as a single long-lived magmatic event. However, in this study, two stages (Late Carboniferous and Permian–Triassic) of magmatism in the Strandja Zone are now proposed. The first episode took place in the Late Carboniferous between c. 312 Ma and c. 295 Ma (Table S3, in the Supplementary Material available online at https://doi.org/10.1017/S0016756821000650; Georgiev et al. Reference Georgiev, Von Quadt, Heinrich, Peytcheva and Marchev2012; Machev et al. Reference Machev, Ganev and Klain2015; Natal’in et al. Reference Natal’in, Sunal, Gün, Wang and Zhiqing2016; Peytcheva et al. Reference Peytcheva, Georgiev and Von Quadt2016; Bonev et al. Reference Bonev, Filipov, Raicheva and Moritz2019 a; Pristavova et al. Reference Pristavova, Tzankova, Gospodinov and Filipov2019). It started with the intrusion of several small unnamed bodies described from the Kasatura and Kaletepe regions near Kıyiköy in the Strandja Unit (Fig. 1; Natal’in et al. Reference Natal’in, Sunal, Gün, Wang and Zhiqing2016), and was followed by extensive emplacement of large plutons between c. 305 Ma and 295 Ma, e.g. the Sakar and Central Strandja batholiths. However, the presence of c. 320 to c. 310 Ma zircon antecrysts within plutonic rocks of the Sakar Batholith, Ustrem Pluton and Melnitsa Complex (Bonev et al. Reference Bonev, Filipov, Raicheva and Moritz2019 a) suggests that this early-stage magmatism was voluminous within the Strandja Zone. It confirms similarities to neighbouring areas where felsic intrusions with ages of c. 320–310 Ma are widespread, e.g. the Sakarya Zone (Ustaömer et al. Reference Ustaömer, Ustaömer and Robertson2012, Reference Ustaömer, Robertson, Robertson, Ustaömer, Gerdes, Peytcheva, Robertson, Parlak and Ünlügenç2013) and the eastern Mediterranean realm (Meinhold et al. Reference Meinhold, Reischmann, Kostopoulos, Lehnert, Matukov and Sergeev2008). Following the c. 312 to c. 295 Ma magmatic activity, after c. 20 Myr of quiescence, a more extended period of magmatism began in the Permian at c. 275 Ma, and which ceased in the Triassic by c. 230 Ma. The ages of the granitic bodies from this period are well established from the Strandja Unit (Natal’in et al. Reference Natal’in, Sunal, Gün, Wang and Zhiqing2016; Aysal et al. Reference Aysal, Şahin, Güngör, Peytcheva and Öngen2018), whereas previously there was no evidence of coeval magmatism within the Sakar Unit. However, in this study, c. 251 Ma to c. 256 Ma ages for the Izvorovo Pluton are documented from the Sakar Unit. Additionally, c. 245 Ma to c. 230 Ma ages were presented for the Harmanli Block Magmatic Complex and St Iliya Heights (Bonev et al. Reference Bonev, Filipov, Raicheva and Moritz2019 b, Reference Bonev, Filipov, Raicheva and Moritz2020 a; Fig. 7).
These new data allow for the division of felsic to intermediate magmatism within the Strandja Zone into two age groups: (1) a short Late Carboniferous (c. 312 to c. 295 Ma) extensive magmatic event; and (2) Permian–Triassic magmatism (c. 275 to c. 230 Ma; Fig. 7b). These two age groups show significant differences in the characteristics of the zircon populations. The first group of Late Carboniferous granites show that within the population of typical oscillatory-zoned zircons, inherited components and antecrysts play an important role. In the c. 298 Ma albitized Sakar granitoid (Kanarata Quarry, Sakar Batholith), a considerable part of the analysed grains yielded ages between c. 330 Ma and c. 310 Ma, and were interpreted as antecrysts (Pristavova et al. Reference Pristavova, Tzankova, Gospodinov and Filipov2019). Similar results were obtained from a c. 296 Ma porphyritic granite near Planinovo in the Sakar Batholith (sample S14), where half of the concordant zircon data range from c. 340 Ma to c. 310 Ma, and were interpreted as antecrysts. There is also a minor population of xenocrysts (at c. 556 Ma and c. 636 Ma; Bonev et al. Reference Bonev, Filipov, Raicheva and Moritz2019 a). A c. 295 Ma equigranular granite (Balgarska Polyana, Sakar Batholith, sample S4), dated by Bonev et al. (Reference Bonev, Filipov, Raicheva and Moritz2019 a), also contains abundant xenocrystic material (almost half of the concordant data) within the zircon population, with a broad age spectrum between c. 600 Ma and c. 370 Ma. The dominance of 330–310 Ma antecrysts within zircon populations was also detected from a c. 306 Ma meta-quartz diorite from the Ustrem Pluton (sample S36) and a c. 300 Ma porphyritic meta-granite from the Melnitsa Complex (sample S18), where they represent ˜80 % of the concordant data (Bonev et al. Reference Bonev, Filipov, Raicheva and Moritz2019 a). Because Late Carboniferous granitoids contain a large spectrum of components inside the zircon population, with a variety of ages ranging between c. 600 Ma and 305–295 Ma (the age of intrusion), the group can be classified as inheritance-rich granitoids.
On the other hand, the second group of Permian–Triassic intrusions do not contain a significant amount of antecrysts and xenocrysts. Aysal et al. (Reference Aysal, Şahin, Güngör, Peytcheva and Öngen2018) showed that the zircon population of the c. 268 Ma Kırklareli meta-granite (sample KG-1) contained only a few inherited cores. Another study published by Natal’in et al. (Reference Natal’in, Sunal, Gün, Wang and Zhiqing2016) showed that within the zircon population of a c. 244 Ma leucocratic dyke cutting the Kırklareli Pluton (sample KI-231), all of the xenocrysts have an age directly related to the age of Kırklareli Pluton. This age range is between c. 270 Ma and c. 250 Ma, and therefore probably comes from assimilation of the host rock. A sample of c. 252 Ma porphyritic meta-granite from Ömeroba Pluton (KI-233.3) contains only two xenocrysts, with ages of c. 360 Ma and c. 330 Ma, respectively (Natal’in et al. Reference Natal’in, Sunal, Gün, Wang and Zhiqing2016). A c. 249 Ma meta-granitoid from Tepecik Pluton (sample CT-23) had no xenocrysts or antecrysts (Aysal et al. Reference Aysal, Şahin, Güngör, Peytcheva and Öngen2018). Samples from Izvorovo Pluton contain only a few xenocrysts dated at c. 440 Ma and c. 323 Ma (sample SAK-40) and at c. 460 Ma and c. 321 Ma (SAK-42), respectively. The Permian–Triassic group represents inheritance-poor granitoids.
Zircon saturation temperatures (T Zr ) calculated from bulk-rock compositions (after Watson & Harrison, Reference Watson and Harrison1983; Table S2, in the Supplementary Material available online at https://doi.org/10.1017/S0016756821000650) were used to estimate temperatures at which the magma solidified. The temperature calculated for the Sakar Batholith ranges between 717 °C and 805 °C (with average of 760 °C), and for other Late Carboniferous intrusions it is in the range 760–811 °C (with an average of 775 °C). The calculation for Permian Kırklareli Pluton and Triassic Tepecik Pluton yielded temperatures between 778 °C and 852 °C (mean of 805 °C) and 766 °C and 851 °C (mean of 810 °C, excluding one outlier of 932 °C), respectively. The zircon saturation temperatures for Permian Izvorovo Pluton from this study vary between 756 °C and 787 °C (mean of 768 °C). Therefore the Late Carboniferous granitoids with abundant inheritance yield lower T Zr than inheritance-poor Permian–Triassic rocks with the exception of Izvorovo Pluton. According to Miller et al. (Reference Miller, McDowell and Mapes2003), the zircon saturation temperature should be interpreted in different ways for inheritance-rich and inheritance-poor granitoids. Inherited zircon components present in the granitoids indicate that the source was zircon-saturated and Zr is present mainly in crystals rather than melt, and T Zr gives the upper limit on magma temperature. The lack of zircon inherited components implies that the source was undersaturated, and therefore the T Zr calculated for inheritance-poor granitoids indicates a minimum initial magma temperature (Miller et al. Reference Miller, McDowell and Mapes2003). This shows significant differences in magma temperatures between the Late-Carboniferous and Permian–Triassic intrusions in the Strandja Zone, because the maximum magma temperatures calculated for the inheritance-rich Late Carboniferous granitoids does not exceed 760 °C (for Sakar Batholith) and 775 °C (for other coeval granitoids), whereas the minimum magma temperatures for Permian–Triassic intrusions are 768 °C, 805 °C and 810 °C for the Izvorovo, Kırklareli and Tepecik plutons, respectively. Miller et al. (Reference Miller, McDowell and Mapes2003) proposed that there is a fundamentally different mechanism of melt production between inheritance-rich and -poor intrusions. Inheritance-poor granitoids are interpreted according to currently accepted modes of felsic magma generation (i.e. dehydration melting in the crust; fractionation of mantle melts, with or without crustal contamination) and without incorporation of the inherited component during transport. However, generation of ‘colder’, inheritance-rich magma requires a source of water to lower the melt temperature, as the most visible mechanism for large-scale melting at temperatures <800 °C is infiltration of a water-rich fluid phase (Miller et al. Reference Miller, McDowell and Mapes2003). Therefore, the inheritance-rich Late Carboniferous granitoids in the Strandja Zone were generated in a tectonic setting that did not reach especially high temperatures but was associated with fluid influx. The inheritance-poor Permian–Triassic intrusions were generated in a hotter, less fluid-rich environment; such settings include extensional or transtensional (Miller et al. Reference Miller, McDowell and Mapes2003). This may be attributed to a possible change in the tectonic setting across the Carboniferous–Permian boundary.
The Late Carboniferous intrusions from the Strandja Zone invite correlation with the other Variscan post-collisional granites in the Balkanides. As shown by Carrigan et al. (Reference Carrigan, Mukasa, Haydoutov and Kolcheva2005), the dominant magmatic episode in the Balkanides took place in the interval between c. 315 Ma and c. 290 Ma. These authors also highlight that the Variscan Orogen in Bulgaria and the surrounding areas does not include an older phase of c. 340 Ma to 325 Ma magmatism, which was confirmed by recent work (e.g. Balkanska et al. Reference Balkanska, Gerdjikov, Georfiev, Lazarova, Dörr and Kounov2021). This implies a similar evolution of the Balkanides and the western part of the Variscan Orogeny exposed in the Iberian Massif, where c. 340 Ma to c. 325 Ma felsic intrusions are also absent (Dias et al. Reference Dias, Leterrier, Mendes, Simões and Bertrand1998; Fernández-Suárez et al. Reference Fernández-Suárez, Dunning, Jenner and Gutiérrez-Alonso2000). A correlation between c. 315 Ma to c. 290 Ma post-collisional magmatism of the Balkanides and the Late Carboniferous meta-granitoids from Strandja Zone was proposed by Peytcheva et al. (Reference Peytcheva, Georgiev and Von Quadt2016). This interpretation has been disputed by other studies, which grouped together Late Carboniferous and Permian intrusions in the Strandja Zone into one long-lived period of subduction-related magmatism (e.g. Sunal et al. Reference Sunal, Natal’in, Satir and Toraman2006; Natalin et al. Reference Natal’in, Sunal, Gün, Wang and Zhiqing2016; Aysal et al. Reference Aysal, Şahin, Güngör, Peytcheva and Öngen2018; Bonev et al. Reference Bonev, Filipov, Raicheva and Moritz2019 a). However, in this study, it is shown that Late Carboniferous to Triassic felsic to intermediate magmatism in the Strandja Zone represents two separate temporal stages. The younger, Permian–Triassic group is attributed to either subduction-related magmatism according to earlier models proposed for the Strandja Zone (i.e. Bonev et al. Reference Bonev, Filipov, Raicheva and Moritz2019 a) or a rift-related setting, e.g. as inferred for the Western Pontides (Okay & Nikishin, 2015), or both, assuming a transition from a Permian arc setting to a Middle Permian – Early Triassic back-arc setting (Aysal et al. Reference Aysal, Şahin, Güngör, Peytcheva and Öngen2018). A rift-related environment is probably more likely as it may facilitate the high-temperature conditions required for magma generation (Miller et al. Reference Miller, McDowell and Mapes2003). A rift-related setting for Permian to Triassic granitoids in the Strandja Zone is supported by the occurrence of a Permian–Triassic sequence of sedimentary rocks in the Topolovgrad Group located in the northeastern part of Sakar Unit (Chatalov, Reference Chatalov1990; Zagorchev et al. Reference Zagorchev, Dabovski and Nikolov2009), which potentially have a back-arc basin affinity.
7. Conclusions
This study presents the first evidence of Permian magmatism in the Sakar Unit of the Strandja Zone, detected from the c. 251 to 256 Ma Izvorovo Pluton dated in this study. These ages disprove the interpretation of the Izvorovo Pluton as a possible heat source for Late Jurassic to Early Cretaceous metamorphism in the Sakar Unit proposed by Ivanov et al. (Reference Ivanov, Gerdjikov and Kounov2001) and Gerdjikov (Reference Gerdjikov2005). The Izvorovo Pluton is one of the largest magmatic bodies within the Sakar Unit, which has a complex magmatic evolution. Permian magmatism has already been well documented in the Strandja Unit, therefore this study allows for better correlation between the various magmatic units within the Strandja Zone. The results presented here highlight the significance of long-lived Permian–Triassic magmatism within the Strandja Zone (Fig. 7). The large granitic bodies intruded into pre-Late Carboniferous basement throughout the whole area from the westernmost vicinity of the Strandja Zone near Harmanli, to the southeastern part located close to Istanbul. These plutons consist of variably deformed and metamorphosed granitoids, which show evidence of post-Triassic metamorphism and deformation. However, all the presented data (i.e. field observations, petrography, geochemistry and geochronology), indicate that even the strongly sheared porphyroclastic gneisses preserve their igneous origin. Permian–Triassic magmatism was preceded by Late Carboniferous to Early Permian (c. 312 to c. 295 Ma) intrusions known from both parts of the Strandja Zone (e.g. the Sakar and Central Strandja batholiths; Table S3, in the Supplementary Material available online at https://doi.org/10.1017/S0016756821000650). Between an intensive short-lived Late Carboniferous event and a series of long-lived Permian to Triassic magmatic events, there was c. 20 Myr of magmatic quiescence. These two groups were distinguished based on geochronological data, and supported by inherited zircon populations and zircon saturation temperatures.
The two stages of felsic to intermediate magmatism during the Late Carboniferous to Triassic are attributed to changes in tectonic setting. One possible scenario is emplacement of post-collisional Late Carboniferous granitoids associated with the Variscan Orogeny. This was followed by intrusion of Permian–Triassic granitoids connected either with subduction-related or rift-related settings. The subduction-related scenario is in agreement with the interpretation of the Variscan Belt evolution in the Black Sea region (Okay & Topuz, Reference Okay and Topuz2017), whereas the rift-related scenario is consistent with models proposed for the western part of Pontides (i.e. Okay & Nikishin, 2015). This emphasizes the significance of the Strandja Zone as a link in the evolution of the Balkanides and Pontides.
Acknowledgements
We wish to thank the Editor, Peter Clift, as well as Wolfgang Dörr and two anonymous reviewers for comments which improved the manuscript. This research was supported by a Preludium Grant awarded to A.S. from the National Science Centre (Narodowe Centrum Nauki), NCN, in Poland (grant agreement no. UMO-2018/29/N/ST10/00368). D.C. is supported in part by a research grant from Science Foundation Ireland (SFI) under Grant Number 13/RC/2092 and 13/RC/2092_P2 and co-funded under the European Regional Development Fund and by PIPCO RSG and its member companies. The authors would like to thank Nikolay Gospodinov for logistical support during the field trip, and Rafał Juroszek for help with the BSE imaging, as well as Alex Kounov for scientific discussions.
Declaration of interest
The authors declare none
Supplementary material
To view supplementary material for this article, please visit https://doi.org/10.1017/S0016756821000650