Introduction
In the framework of the International Trans-Antarctic Scientific Expedition (ITASE) and Chinese Antarctic glaciological research program, glaciological studies have been conducted along the transect from Zhongshan station to Dome A. The first traverse expedition was carried out to a point 296 km inland from Zhongshan station during the 1996/97 austral summer (13th Chinese National Antarctic Research Expedition (CHINARE)). The traverse was extended southwards to 464 km during the 1997/98 (14th CHINARE) and to 1128 km during the 1998/99 (15th CHINARE) field season. The accumulation rate was recovered from stake measurements, snow pits and shallow firn cores (50–100 m). Stable-isotope and chemical records were also determined for the snow pits and firn cores. Radio-echo sounding was carried out along the 1128 km route during the 1998/99 expedition, but the results are not yet available.
The initial 294 km of the route is partly coincident with the Australian National Antarctic Research Expeditions (ANARE) traverse route surrounding the Lambert Glacier basin (LGB). In the 1993/94 and 1994/95 field seasons, the LGB oversnow traverse program covered the routes from the west to the east side of the LGB and terminated at LGB72, which is 68 km from Zhongshan station. As the main component in calculating the mass budget for the LGB drainage basin, the surface mass balance over this region was estimated by Reference AllisonAllison (1979) and Reference MclntyreMclntyre (1985) from isolated measurements and interpretation of satellite imagery respectively. Over the section from LGB72 to LGB65 (294 km from Zhongshan station), surface accumulation, snow density, 10 m firn temperature and «5180 measurements were conducted during the ANARE traverse.
Investigations of the surface mass balance over the vast ice sheet are still relatively scarce, and mass-balance studies over ice-drainage areas such as the LGB are essential for precise mass-balance calculation for the whole ice sheet. The data on surface accumulation rates and their spatial and temporal variability are important for driving ice-sheet models and testing atmospheric models. The route of the Chinese traverse is mainly located in Princess Elizabeth Land and Inaccessible Area. Up to now, there has been little glaciological investigation of the regions covered by the final 550 km of the route. In this paper, the spatial variation of snow density and the surface accumulation rate over the initial 464 km of the route are presented, and the data for the initial 294 km compared with those obtained by ANARE. Snow-chemistry analyses were conducted for snow pits and firn cores, of which only the results from the first traverse are shown.
Sampling and Experimental Procedures
Figure 1 shows the area under investigation, with the five drilling sites of the 27–102 m deep firn cores at DT001 (50 m), DT085 (52 m), DT263 (82 m), DT401 (102 m) and MGA (27 m). Two snow-pit sites, at LT921 (2.2 m) and DT001 (3.2 m) on the east side of the LGB, are also shown. The MGA firn core was extracted during the 1992/93 ANARE traverse, which included one of the authors (Reference Goodwin, Higham, Allison and JiawenGoodwin and others, 1994; Reference Renjiawen and AllisonRen and others, 1999). Snow pits were sampled every 3 cm along one of the pit walls. The firn cores at DT001 and MGA were also resampled every 3 cm in the cold storage at the Lanzhou Institute of Glaciology and Geocryology, China.

Fig. 1. Sketch map showing the route of the Chinese Antarctic inland traverses during the years 1996–99. Circles represent the locations where ice/firn cores and snow-pit samples discussed in the text, were obtained. The dashed line is the ANARE traverse route.
All samples were kept frozen until processed. Concentrations of major cations and anions were measured by Dionex-300 and Dionex-100 ion chromatography, respectively. The detection limits and precision, respectively, were 1 × l0–6ggȡ1 and ± 5% for Na+, CF and SO42, and 1 × 10–6–gg–1 and ±20% for Ca2+. The 5180 measurements were done by MAT-252 Gas Stable Isotope Ratio Mass Spectrometer, with respect to standard mean ocean water measurement, to a precision of ±0.5%o.
Isotope and Chemistry Profiles and Dating of Snow Pits and Firn Cores
Reliable chronologies are the first step for snow/ice-core studies. Various methods can be used to provide dating of depth profiles. These include stratigraphic studies, reference horizons, radioactive decay of some radionuclides and comparison with other records. In principle, numerous stratigraphical methods based on seasonal changes in the isotopic composition of the ice or in the concentration of impurities, as well as in physical properties of snow, may be used to establish year-to-year dating of ice cores.
The chemical profiles from the LT921 and DT001 snow pits, as well as the upper layers of the DT001 firn core, show that «5180, CF, Na+and NO3– have clear seasonal variations, and thus provide a relatively reliable basis for the dating of snow pits or firn cores in this region (Fig. 2a and b). Among the chemical species currently measured in polar snow layers, it appears that sodium and chloride exhibit very strong seasonal cycles. For instance, concentrations of sodium (a good tracer of sea salt) exhibit large variations (a winter to summer ratio of 5–10) in Antarctic snow layers, due to frequent advection of marine air masses in winter over the ice sheet (Reference HerronHerron, 1982). Because an additional input of hydrochloric acid takes place in summer, the seasonal variation of chloride is weaker than that of sodium. Besides these two species, acidic species like nitrate and sulfate exhibit moderate maxima in summer and spring in Antarctic snow layers. The accuracy of the dating is much increased when several parameters are considered together. It is clear from Figure 2 that the variations of NO3– are partly in phase with those of δ18O, while the variations of CF and Na+are in anti-phase with those of δ18O, except for the sodium record in Figure 2a, which shows no clear correlation. However, the seasonal variations of δ18O are gradually smoothed below 3 m (corresponding to about ten annual layers), while the seasonal variations of Cl- Na+ and NO3– are fairly consistent throughout the profile of the 50 m firn core (about 250 annual layers; Fig. 2c and d). No obvious seasonal variations of Ga2+ are found in the snow-pit profiles and the firn core.

Fig. 2. δ18O, Cl–,Na+,NO3– and Ca2+ profiles in snow pits at LT921 (a) and DT00l (b) covering the periods 1991–96and 1984–96, respectively; and in firn cores at DT001 covering depths 24–26 m (c) and36–38m (d).
Density
Snow density was measured by weighing the samples collected using a hollow steel tube with a volume of 10 cm × 10 cm × 2 cm. The density of the upper 0.2 m of snow was measured at about 8 km intervals 130–450 km along the traverse route (Fig. 3). In the section from 130 to 350 km the surface density is relatively stable (0.4–0.5 g cnF3), while from 350 to 450 km it displays considerable spatial variations, although most values are still in the range 0.4–0.5g cm–3. This is because katabatic winds dominate at 2500–3000 ma.s.L, and as a result sastrugi 30 cm high or more are widespread in this section. The surface roughness in this latitudinal range is much larger than in other sections of the route. Wind speed is a key factor for the formation of the surface roughness over the vast ice sheet. The annual mean wind speed for 1995 recorded by an automatic weather station (AWS) installed at LGB59 (2537ma.s.L), close to DT085 (2577 m a.s.L), is 9.7 msȡ1. At the coastal Zhongshan station, the annual mean for 1995 is 7.3 ms–1, although the anemometer height at Zhongshan is actually higher than at LGB59. Simultaneous observations during the traverse also indicated higher wind speeds (9–12 m s"1) in this section than in the lower or higher latitudinal ranges (6–10 ms–1). Reference Craven and AllisonCraven and Allison (1998) examined the dependence of firnification on temperature, wind and accumulation rate using two empirical models, based on firn-core data from widely spread Antarctic sites. They showed that a 5 m s"1 wind-speed increase has an effect equivalent to that of a 10°C temperature increase, or a 0.1 m a"1 accumulation-rate decrease. Strong wind has an obvious effect in increasing the firnification rate.

Fig. 3. The distribution of the upper 0.2 m snow density measured at about 8 km intervals along the initial 450 km of the traverse route (measurement starts from 130 km south of Zhongshan).
The surface density was also measured over the upper 1 m of firn, with a 2 cm sampling interval. The density profiles are quite stable, in the range 0.35–0.45g cm–3 (Fig. 4). Generally, before the density reaches 0.55g cm–3, the densification process is dominated by grain-settling, sublimation/ condensation, volume/surface diffusion as well as recyrstallization. Since the process does not involve meltwater in the interior of the Antarctic ice sheet, it is so-called ˚dry densification". Reference DaheQin (1990) classified it as cold-type densification, characterized by a primary mechanism of sintering, which occurs over the vast central regions of Antarctica (the northern limit is approximately 100 km inland from the coast) where the annual temperature is <-25°C, with a maximum of ω0°C. Wind speed plays an important role during dry densification of the surface layer of snow, while another important factor may be the annual mean temperature. The role of the overburden pressure is negligible in such shallow layers. Since strong wind over Princess Elizabeth Land runs towards the LGB all the year round, the snowfall in almost all seasons can rapidly be packed onto the surface of the ice sheet by the wind. Therefore, the seasonal variation in density is not obvious with a sampling resolution of 2 cm.

Fig. 4. Density profiles in the upper 1m of snow at six sites over the northern 464 km section of the line.
Continuous density measurement was carried out on the firn cores drilled at DT001, DT085, DT263 and DT401 (Fig. 5). Probably because of the enhanced densification in upper layers, resulting from the high wind speed, linear regressions of the density vs depth for the firn core at DT001 and DT085 are a better fit than logarithmic functions. The same finding was made over the inland region of the western LGB (Graven and Reference AllisonAllison, 1998). However, for the 80 m core at DT263 and the 100 m core at DT401, good logarithmic correlations were established (τ = 0.80 and 0.93, respectively). The depth of pore close-off (corresponding to a density of 0.83 g cm–3) is around 60 m at both DT263 and DT401. If the regression lines are extended for the density profiles of DT001 and DT085, the depth of the value 0.83 g cnT3 is also around 60 m. This means that the densification process in this large region (290–1100 km along the route), is fairly slow, as at other interior Antarctic sites.

Fig. 5. Density profiles in the Jim cores drilled at DT001, DT085,DT263andDT401.
Spatial and Temporal Variations of Accumulation Rate
Individual measurements of surface mass balance were made on bamboo canes spaced at 2 km intervals along the whole traverse route. For the section from 460 to 1128 km, only initial values of the cane height are available, so the accumulation rate cannot be calculated yet. The annual mean accumulation rate for 1997 along the coastal 460 km is plotted in Figure 6a. The seven-point-smoothed profile gives the trend of the spatial variation. It displays relatively high values (up to 200mm a–1) of snow accumulation for the initial 200 km, and then declines and remains stable (0–100 mm a"1) further south, with a few individual sites having below-zero accumulation. This spatial variation is compared with the annual accumulation measured by ANARE in 1994 along a partly coincident route (LGB72 to LT782; Reference Higham and CravenHigham and Graven, 1997). The two profiles display similar spatial trends, but with the average values for 1994 over three times higher than those for 1997, which may imply a much higher precipitation in 1994. However, in the section south of 294 km the two lines separate, leading to different spatial trends.

Fig. 6. The distribution of the annual accumulation rate in 1997 along the initial 460 km of the traverse lines on both sides of the LGB. The seven-point-smoothed profiles give the trends of spatial variations (bold lines). The value for 1994 over the eastern LGB is the 30 km unweighted mean of the measuring sites (Reference Higham and CravenHigham and Craven, 1997). (a) The two traverse lines conducted by CHINARE and AN ARE separate after 294 km; the accumulation over this section is shown by dashed profiles, (b) The start point (LGB00) lies 130 km inland of the coast.
The same study was performed by Australian scientists on the west side of the LGB (Reference Goodwin, Higham, Allison and JiawenGoodwin and others, 1994; Reference Higham, Craven, Ruddell and AllisonHigham and others, 1997). In Figure 6b, surface accumulation rate in the 460 km section from LGB00 to LGB20 (site LGB00 is 130 km inland) in the western LGB is plotted. The values are the annual accumulation rates averaged for the years 1990–93. The values over the first 150 km are roughly in the range 0–120 mm a"1 (with a few sites below zero), while in the section from 150 to 400 km they are 60–160 mm a"1, and in the section from 400 to 460 km they are 0–120 mm a"1 again. It has long been considered that the accumulation rates on the two sides of the LGB are quite different. Reference Higham, Craven, Ruddell and AllisonHigham and others (1997) believe that the difference is caused by dominant southeasterly wind fields under the combined influence of katabatic and geostrophic flows. Reference AllisonAllison (1998) suggests that the accumulation rate on the eastern LGB is up to 50% less than at the equivalent elevation on the western side due to the ˚rain-shadow" effect of the prevailing upper-level winds.
Presumably, the difference in spatial variations between the eastern and western LGB is due to the difference in the main vapor-transport paths. On the west side of the LGB, the flattest region is around the head of Amery Ice Shelf, from where the vapor could be transported most efficiently, while the steep coast near Mawson station makes for less efficient transport. This would explain the higher accumulation in the middle of the western LGB traverse line, and the lower accumulation over the initial section. On the east side, by contrast, the topography is steep in all directions inland from the LGB, and the coastal area is the most favorable direction for vapor transportation, leading to the declining trend of accumulation inland from the coast. Short-distance variation of accumulation is closely related to the micro-relief
The accumulation rates were also recovered from several snow pits along the initial 500 km of the route, giving values in the range 4.6–21 cm (46–210 kg m–2 a–1) water equivalent. The initial 500 km of the route can be divided into four subsections based on the accumulation-rate differences. The accumulation rate is highest on the section from 0 to 65 km (140–210 kgm–2a–16 ) ; still high in the section from 65 to 140 km (70–140 kg m-V1 ) ; lower from 140 to 280 km (<46kgm–2a–1); and medium from 280 to 500 km (46–70kgm–2a–1) . This pattern approximately coincides with the cane measurements along the line.
Based on the dating of the ice core at DT001 and density measurement, the history of the annual accumulation rates during the past 50 years is deduced (Fig. 7). The general trend at DT001 is slightly increasing, except for lower values in the decade 1957–67. But at MGA, western LGB, annual accumulation rates during the same period display an obviously decreasing trend. Although a decreasing accumulation rate has occasionally been reported (Reference Graf, Reinwarth, Oerter, Dyurgerov and MillerGraf and others, 1990; Reference Kameda, Nakawo, Mae, Watanabe and NaruseKameda and others, 1990; Reference Bindschadler, Vornberger and ShabtaieBindschadler and others, 1993), most studies reveal that accumulation rates have been increasing recently in Antarctica (Reference Pourchet, Pinglot and LoriusPourchet and others, 1983; Reference Peel and MulvaneyPeel and Mulvaney, 1988; Reference Morgan, Goodwin, Etheridge and WookeyMorgan and others, 1991; Reference Mosley-ThompsonMosley-Thompson and others, 1995). This suggests a complexity, either in the spatial or in the temporal sense, of the accumulation-rate variation over the continent. The different accumulation rates on the two sides of the LGB may be attributable to the reciprocal relation of focal circulation strength between the two sides.

Fig 7. Contrast of the annual accumulation rate and δ18O profiles between firm cores at DT001 (east LGB) and MGA (west LGB), both covering the past 50years. The δ18O profile for MGA is seven-point smoothed in order to give a comparable trend with that of accumulation rate. Instrumental temperature records at Davis station (east coast of LGB) and Mawson station (west coast of LGB) are illustrated, covering the period from the mid-1950s to 1996.
18 O-T Relationships and Past Climate
Figure 8 illustrates the relationships of δ18O vs mean annual temperature, T, of the two sides of the LGB. The plotted 5180 is the mean value in the upper 2 m of firn. Because of the lack of an integrated temperature series recorded by AWS, 10 m firn temperature (T10) was used as a substitute for mean annual temperature (Reference Higham and CravenHigham and Craven, 1997). Results from another coastal Antarctic transect, from Komsomolskaya to Mirny undertaken during the 1990 International Trans-Antarctic Expedition (ITAE; Reference Dahe, Petit, Jouzel and StievenardQin and others, 1994), are also illustrated in Figure 8. It is clear that the 5 1 8 0 –T gradients on both sides of the LGB are different, with 0.70%o °C–1: on the east side, and on the west side 0.93%o ˚C–1. The gradient on the west side is closer to that on the transect from Komsomolskaya to Mirny (0.90%o ˚C–1). On the whole, 5180 values in the Antarctic snow are dependent on the distance of vapor transport, but the topographic factor cannot be ignored since temperature and precipitation are different at various altitudes. Early studies have revealed that the δ18O-T relationships are different in various regions of Antarctica (Reference Dansgaard, Johnsen, Clausen and GundestrupDansgaard and others, 1973; Reference Lorius and MerlivatLorius and Merlivat, 1977; Reference RobinRobin, 1983; Reference PatersonPaterson, 1994; Reference Dahe, Petit, Jouzel and StievenardQin and others, 1994). The 5 1 8 0 –T relationship is a key function used for the recovery of past climatic history from deep ice cores. The establishment of the function in different regions is necessary for precise interpretation of paleoclimate.
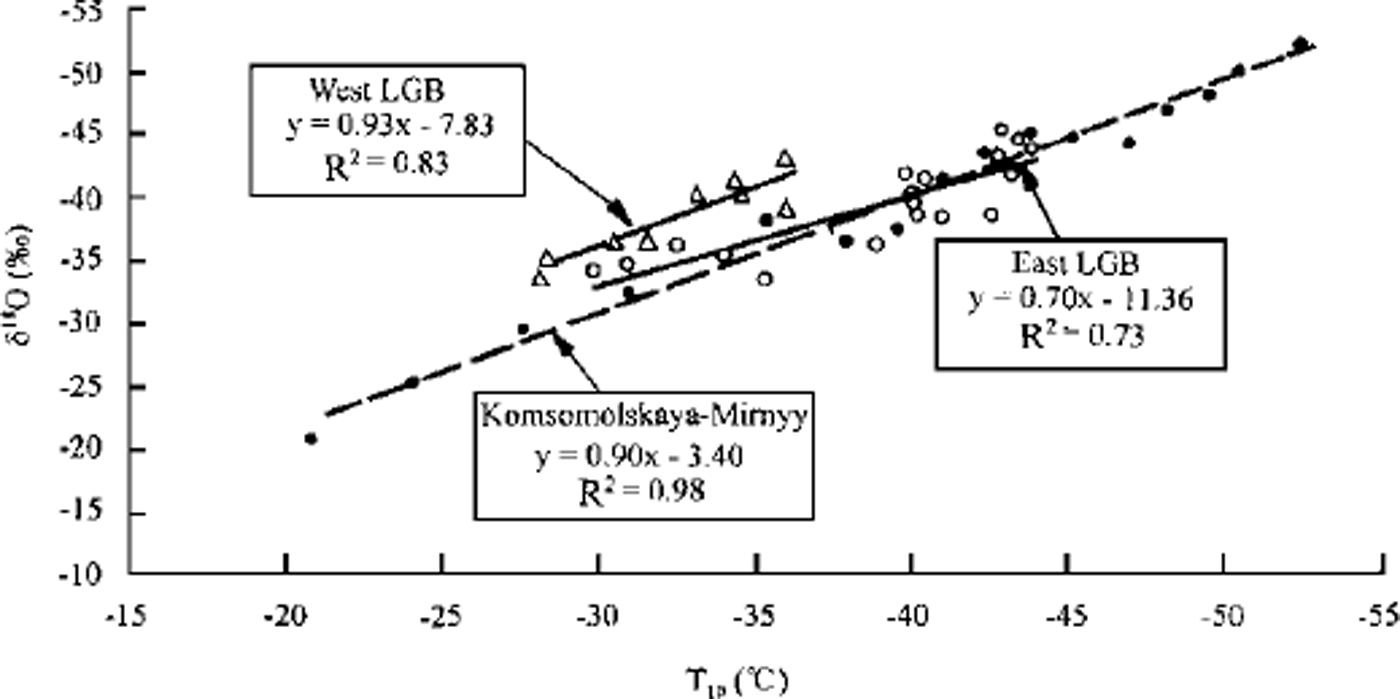
Fig. 8. Correlation of≈ vs Tw along the two sides of the LGB, compared with the results obtained between Komsomolskaya and Mirny during the 1990ITAE (Reference Dahe, Petit, Jouzel and StievenardQin and others, 1994).
The different δ18O –T relationships on the two sides of a glacier basin (LGB), an indicator of different meteorological regimes (Reference AllisonAllison, 1998), confirm the necessity for widespread investigation of the δ18O-T relationships in different areas of the Antarctic ice sheet. One must be extremely careful about using a single function for a wide area of the continent.
During the past 50 years, the δ18O profile at DT001 shows a slight increase, with a gradient of 0.025%o a–1, which may imply an increase in air temperature (Fig. 7). Also, the gradient of the accumulation rate during this period increases by about 039 kg m–2 a–1. The temperature trends, shown by the δ18O profiles in the ice cores at DT001 and MGA on opposite sides of the LGB, are different, with the east side showing an increase and the west side showing no obvious trend. This is similar to the relationship of the accumulation rates on the two sides. Temperature records from the weather stations at Mawson (west coast of LGB) and Davis (east coast of LGB) also reveal converse trends between the two stations (Reference Jacka and BuddJacka and Budd, 1998), with temperature increasing at Davis and decreasing at Mawson over recent decades. The declining tendency of temperature seems to go against the widespread acceptance that the climate has been warming over recent decades. As suggested by Reference Jacka and BuddJacka and Budd (1998), it may become possible to determine the interrelationships between the sea-ice and temperature changes by combining the archived climatic data for sea ice, atmosphere and ocean with modeling. In turn, this determination is important for understanding past climate records over decadal to centennial scales.
Concluding Remarks
Primary results of glaciological investigations from three traverses, which cover a route that now totals 1128 km from Zhongshan station to Dome A, are presented. They are compared with the results obtained by Australian scientists from both sides of the LGB. Physical properties of the firn, such as density profiles, indicate that the densification process over Princess Elizabeth Land and Inaccessible Area is fairly slow. Comparison of accumulation rates, δ18O –T relationships and δ18O profiles illustrates different relationships on either side of the LGB, both in a spatial and in a temporal sense. This may imply different climatic conditions on the two sides. However, the differences in accumulation and temperature over the past 50 years between the two sides are believed to be mainly due to the shifting of focal circulation or sea-ice extent or their interaction. The comparison study in this area shows the necessity for careful, long-term investigations of complicated drainage areas such as the LGB, not only because of their significance for the accurate estimation of the mass balance of the ice sheet, but also because of their importance in revealing the real climate regime of Antarctica. This paper includes data from the first traverse plus a small amount from the second and third traverses. Most of the laboratory analyses and interpretations are still in progress, and more complete results are expected.
Acknowledgements
The authors are grateful to the Chinese Polar Administration for logistic work for the inland traverses. This project was supported by the State Commission of Sciences and Technology of China (98–927), the Chinese Academy of Sciences (KZ951-A1-205) and the National Natural Science Foundation (49771022, 49971021).