Introduction
Mountain glaciers are a relatively reliable indicator of climate change, and their recent decline across the world has been linked to global warming (Reference BarryBarry, 2006). Arguably the most serious consequence of their shrinkage is the cumulative impact on global sea-level rise (e.g. Reference Rignot, Rivera and CasassaRignot and others, 2003), but the retreat of mountain glaciers has more localized consequences, affecting the geomorphology and hydrology of the glacial valleys which they occupy (Reference Kaser, Juen, Georges, Gomez and TamayoKaser and others, 2003; Reference Fischer, Kääb, Huggel and NoetzliFischer and others, 2006).
In this paper, we explore the local impacts of glacier retreat in the Caucasus Mountains, Russia, focusing specifically on the changes in supraglacial debris cover and the development of supra-/proglacial lakes. Several studies indicate that these phenomena are intimately linked with glacier retreat (Reference AgetaAgeta and others, 2000). It is also known that glacial lakes can cause locally higher rates of ablation on debris-covered glaciers and that this can result in thermokarst processes and can lead to further glacier retreat (Reference Benn, Wiseman and WarrenBenn and others, 2000). Moreover, glacial lakes, and particularly those that are dammed by ice-cored moraine, are a potentially serious threat to human life because such dams can be prone to catastrophic failure (cf. Reference Richardson and ReynoldsRichardson and Reynolds, 2000).
Monitoring ongoing changes in glacier retreat, supraglacial debris cover and glacial lake development is therefore an important requirement for informed predictions of their future response to climatic forcing and geohazard prediction (Reference KääbKääb and others, 2005). This is particularly relevant in the Caucasus Mountains, where numerous glaciers exist with varying degrees of debris cover and the vast majority of which are known to be retreating (cf. Reference Stokes, Gurney, Shahgedanova and PopovninStokes and others, 2006). Fatal glacier-related debris slides have also been reported in this region (Reference HaeberliHaeberli and others, 2004).
Recent Glacier Change in the Caucasus Mountains
The Caucasus Mountains mark the boundary between Russia and Georgia, extending west-northwest to east-southeast from the Black Sea to the Caspian Sea (see Fig. 1). The mountain range extends for around 1300 km and contains several peaks above 4000 m, the highest of which is Elbrus (5642 m). Estimates place the total glaciated area in the Caucasus Mountains at 1400–1805 km2 (Reference Bedford and BarryBedford and Barry, 1995), with an average ice thickness of ~75m (Reference Bazhev and BadenkovBazhev, 1989).
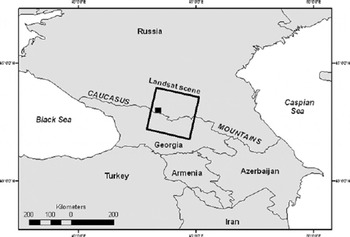
Fig. 1. Location map of the Caucasus Mountains showing the coverage of the Landsat satellite scene (path 131, row 70) and the location of our detailed study area in the Adylsu valley (black rectangle).
In total, there are over 2000 glaciers in the Caucasus, of which around 250 are temperate valley glaciers. Reference SolominaSolomina (2000) reported evidence for widespread glacier retreat since the end of the Little Ice Age (LIA), although the timing of the most recent maximum is somewhat complex, with moraine lichenometry indicating maximum positions around AD 1300, 1700 and 1850 (Reference Serebryannyy, Golodkovskaya, Orlov, Mal-yasova and Il’vesSerebryannyy and others, 1984).
During the 1960s, the number of glaciers in retreat declined from ~80% to ~65%, but the 1970s saw an increasing number of glaciers in retreat, through to the early 1980s (Reference Bedford and BarryBedford and Barry, 1995). More recently, Reference Stokes, Gurney, Shahgedanova and PopovninStokes and others (2006) compared glacier terminus positions on satellite imagery of the Caucasus from 1985 and 2000. Of the 113 glaciers measured, ~95% had retreated. Analysis of mass-balance measurements from Djankuat glacier and local and synoptic climate trends indicated that the recent retreat of Caucasus glaciers is being driven by an increase in summer air temperatures, with no compensating increase in winter precipitation (Reference Shahgedanova, Stokes, Gurney and PopovninShahgedanova and others, 2005).
Reference Stokes, Gurney, Shahgedanova and PopovninStokes and others (2006) also reported a ~10% decrease in the surface area of debris-free ice between 1985 and 2000. They did not directly measure the spatial extent of debris cover on individual glaciers but speculated that it was increasing (as a percentage of glacier area) in tandem with glacier retreat. This assertion is supported by earlier field measurements on Djankuat glacier which indicated that the total area of debris cover increased from 3% to 10% of the entire glacier area between 1968 and 1999 (Reference Popovnin and RozovaPopovnin and Rozova, 2002). Reference Popovnin and RozovaPopovnin and Rozova (2002) also detected a general pattern whereby the more negative the mass balance, the higher the rate of debris accumulation on the glacier surface. Given that the vast majority of glaciers are in retreat in this region, this might imply that supraglacial debris cover is becoming more extensive elsewhere. To date, no data exist to verify or refute this.
In this paper, we build on previous work using satellite imagery (Reference Stokes, Gurney, Shahgedanova and PopovninStokes and others, 2006) and produce detailed maps of debris cover on six glaciers in the Adylsu valley, including Djankuat and Bashkara glaciers, where field investigation has been targeted by the Geographical Faculty of Moscow State University. We also map supra- and proglacial lake coverage across a larger area and compare the satellite image interpretations with the detailed field measurements.
Methods
Three images of our study area were obtained, all from cloud-free scenes towards the end of the ablation season, and dating from 3 August 1985 (Landsat Thematic Mapper (TM)), 28 September 1991 (Landsat TM) and 5 September 2000 (Landsat Enhanced TM Plus (ETM+)). These images were geo-referenced (without correction for topographic distortion) and co-registered using ERDAS Imagine 8.7 software. The glacier outline and areal extent of debris cover on each glacier were manually digitized using a false-colour composite image of TM bands 5, 4, 3 (red, green, blue), as recommended by Reference Paul, Huggel, Kääb, Kellenberger and MaischPaul and others (2003). Although semi-automated techniques to map supraglacial debris cover have been developed recently (e.g. Reference Paul, Huggel and KääbPaul and others, 2004), manual on-screen digitizing can be more accurate, especially where only small numbers of glaciers are being studied, as in this case. Error analysis of the digitizing at a scale of 1 : 30 000 found the accuracy to be of the order of ±25m (i.e. the same glacier/debris-cover outline was independently digitized 20 times and the maximum distance between any two digitized positions was <25 m). Once digitized, the image-processing software was used to measure the surface area of the glacier and the surface area of supraglacial debris cover.
We acknowledge that delineating the boundary between bare ice and debris-covered ice using imagery of this resolution (25 m) is difficult. It may be impossible, for example, to detect small areas of debris cover surrounded by bare glacier ice. Moreover, debris-covered glaciers often show a gradational transition from debris-free ice to debris-covered ice and this makes it difficult to define the margins of the debris cover (it should be remembered, however, that field-surveying of debris cover will also be hindered by this problem). As such, our mapping is essentially detecting contiguous areas of generally thick debris cover which may contain small areas of exposed ice within it. The advantage of this, however, is that we are capturing the main changes in the extent of major areas of debris cover on the glaciers. Thus, although the mapping is unlikely to be entirely robust, it does reveal general patterns in the evolution of debris cover from which we can compare to field measurement. Additionally, we acknowledge that supraglacial debris is likely to fluctuate over much shorter timescales than our 6–9 year repeat measurements can detect, influenced by interannual variations in accumulation and ablation and non-climatic factors (e.g. rockfalls).
Proglacial lakes were readily identifiable on the satellite imagery because their spectral signature is in marked contrast to the glacier ice or debris cover that surrounds them. Rather than focus solely on the Adylsu valley, we broadened our mapping to the area covered by the whole Landsat scene from path 171, row 030.
In addition to our remote-sensing approach, detailed field mapping of supraglacial debris cover has been made on Djankuat glacier. The distribution of supraglacial debris cover has been surveyed at the end of the ablation season, and Reference Popovnin and RozovaPopovnin and Rozova (2002) present incremental data from 1968, 1974, 1984, 1992, 1996 and 1999. We compare our results on the satellite imagery to the detailed data from Djankuat glacier, due to be updated in September 2006. Recently, detailed measurements have also been taken of proglacial lakes impounded against Bashkara glacier, one of the glaciers covered by our detailed mapping. These field data are a very useful way of verifying the accuracy of the satellite image interpretations.
Results
Glacier retreat and evolution of supraglacial debris cover
The six glaciers in the Adylsu valley which were investigated are shown in Figure 2, which also shows their terminus positions in 1985 (outermost limit) and 2000 (innermost limit). It can be seen from Figure 2 that each of the six glaciers in the Adylsu valley has retreated, although at varying rates and distances. This information is detailed in Table 1, which shows the retreat distance and retreat rate for each glacier during each time interval.

Fig. 2. Landsat ETM+ image of Adylsu valley from 2000, showing the location of the six glaciers investigated in this study. The glacier terminus positions in 1985 (outer limit) and 2000 (inner limit) are also shown. For clarity, the terminus position in 1991 is not shown. Note proglacial lake adjacent to the right flank of Bashkara glacier (see also Figs 4 and 5).
Table 1. Retreat distance and rates of six glaciers in the Adylsu valley, central Caucasus

It can be seen from Table 1 that a general pattern emerges whereby the larger glaciers have retreated a greater distance.
This would be expected given that the larger glaciers extend to lower elevations within their respective valleys. In association with glacier retreat, supraglacial debris cover appears to be evolving to cover an ever-increasing area of the glaciers’ surface. Our measurements indicate that between 1985 and 2000 supraglacial debris cover has increased as a percentage of the glacier surface area on all but one of the glaciers (Bzhedukh glacier). These results are shown in Figure 3, which plots the percentage of each glacier covered by supraglacial debris cover at each time increment.

Fig. 3. Plot showing the general increase in debris cover of the six glaciers in the Adylsu valley, as a percentage of the glacier area.
On individual glaciers, supraglacial debris cover ranges from just a few per cent (e.g. Bzhedukh) to over 25% (e.g. Shkhelda), but for all glaciers it generally increases 3–6% between 1985 and 2000. On Djankuat glacier, for example, supraglacial debris cover increased from 6% to 9% between 1985 and 2000. We note that detailed field measurement of debris cover on this glacier by Reference Popovnin and RozovaPopovnin and Rozova (2002) found an increase from 3% to 10% between 1968 and 1999, which is broadly consistent with our remote-sensing data. Indeed, the discrepancy may be explained by the fact that the detailed field surveying would have picked out smaller islands of debris cover which were beyond the resolution of the satellite imagery.
The increase in debris cover on each glacier is characterized by a progressive migration up-glacier. This can be clearly seen in Figure 4, which shows debris cover on the surface of Bashkara glacier at each time-step of the study period.

Fig. 4. Incremental changes in glacier surface area and supraglacial debris cover on Bashkara glacier. Also note the emergence and growth of proglacial lakes adjacent to the glacier terminus and shaded in light grey, shown in Figure 5.
Changes in proglacial/supraglacial lake coverage
It can be seen in Figure 4 that glacier retreat and the increase in supraglacial debris cover on Baskhara glacier has also been accompanied by the emergence and growth of proglacial lakes. The remote mapping clearly indicates the growth of these lakes since 1985 as a recent phenomenon. Indeed, detailed measurements of these lakes indicate that they have progressively increased in size and depth during the last two decades. Figure 5a shows the areal extent of Lapa lake, adjacent to the terminus of Bashkara glacier, and Figure 5b is a photograph of the lake from 2005 (see Fig. 4 for location).

Fig. 5. (a) Recent (2002, 2003, 2004) expansion and joining of two lakes (Lapa and Mizinets) at the terminus of Bashkara glacier, shown in (b). These lakes did not exist in 1985 and continue to enlarge (see Fig. 4 for location).
We also mapped the occurrence of proglacial and supraglacial lakes across the whole area covered by the Landsat scene (path 171, row 30). A synoptic assessment across this region has never been undertaken before, but our results indicate that the growth of lakes in the Adylsu valley follows a regional trend. Table 2 shows the number and areal extent of lakes in 1985 compared to 2000.
Table 2. Comparison between the number and size of proglacial lakes on Landsat scene path 171, row 030 in 1985 and 2000

The data in Table 2 indicate that there were sixteen lakes in 1985, but by 2000 two of these had disappeared, six had decreased in size and eight had increased in size. Moreover, a further ten new lakes had appeared in 2000 that did not exist in 1985. In summary, the total lake area in 1985 was 0.2423 km2 and this increased to 0.3815 km2 in 2000, representing a 57% increase in lake surface area in the study region.
Discussion
With one exception (Bzhedukh glacier), mapping of six neighbouring glaciers indicates that glacier retreat is being accompanied by an increase in the proportion of each glacier covered by supraglacial debris. In general, our results indicate a 3–6% increase in debris cover over the period 1985–2000.
The presence of supraglacial debris can have a profound influence on ablation rates (e.g. Reference Nakawo and RanaNakawo and Rana, 1999). A thin layer of debris (generally <1–2 cm) will lower the albedo of the glacier surface, causing it to absorb more shortwave radiation. This results in an increase in ablation of the underlying and adjacent ice, compared to ‘cleaner’ ice nearby. Thicker debris layers (>5–10 mm), however, have the effect of shielding the underlying ice from incoming solar radiation and this can greatly reduce ablation, compared to cleaner ice nearby (cf. Reference ØstremØstrem, 1959; Reference Bozhinskiy, Krass and PopovninBozhinskiy and others, 1986; Reference Benn and EvansBenn and Evans, 1998). Thus, the highest ablation rates are likely to be focused in areas of thin, patchy debris and/or at the up-glacier limit of the supraglacial debris cover.
The evolution of the debris cover on the glaciers in our study area is characterized by a progressive up-glacier migration of pre-existing coverage (see Fig. 4). This process, accompanied by glacier retreat, is described as ‘backwasting’ (Reference Benn and EvansBenn and Evans, 1998) and occurs when ablation at the snout is greatly reduced by the presence of the debris cover and melting is focused on the debris-free ice on the steeper slopes and at the up-glacier limit of the debris cover. The expansion of supraglacial debris, therefore, is primarily driven by the thinning of the glacier and the progressive exposure of englacial debris at the up-glacier limits of the existing debris cover, where ablation is most intense due to the influence of thinner, patchy debris cover. A similar pattern of up-glacier migration and a change in the locus of maximum ablation has also been detected on Tasman Glacier, New Zealand (Reference Kirkbride and WarrenKirkbride and Warren, 1999). Reference Kirkbride and WarrenKirkbride and Warren (1999) also report the development of an ice-contact proglacial lake adjacent to this glacier, resulting from thermokarst processes.
The process of glacier thinning can lead to other positive feedbacks whereby surface lowering of the lateral margins of the glacier, including in the accumulation area, can lead to slope instability. Additionally, a warming climate can result in permafrost melting which induces instabilities in the high-altitude rock faces. Indeed, examples of this have recently been observed on Djankuat glacier in 2001 and 2003 (see Fig. 6). The 2003 rock avalanche stretched across the whole accumulation area of the glacier and dramatically increased supraglacial debris coverage. This additional supply of supraglacial debris may also play a role in increasing the overall coverage on the Caucasus glaciers.

Fig. 6. Photographs of two rock avalanches on Djankaut glacier in 2001 (a) and 2003 (b), thought to be induced by glacier thinning in the accumulation area and permafrost melting which induce instabilities in the high-altitude rock face.
Towards the lower limits of the glacier where debris cover is at its thickest, ablation can be greatly reduced and the presence of a thick debris mantle may decouple the glacier from climatic forcing such that debris-covered glaciers respond more slowly to climatic changes (Reference Benn and EvansBenn and Evans, 1998; Reference MattsonMattson, 2000; Reference Thompson, Kirkbride and BrockThompson and others, 2000). It would appear, however, that the glaciers in the Adylsu valley are continuing to respond to the recently observed warming trend in the region (Reference Shahgedanova, Stokes, Gurney and PopovninShahgedanova and others, 2005) because glacier retreat is continuing, i.e. the shielding effect of the increased supraglacial debris at the glacier snouts is not enough to offset the retreat trend. It may be that the debris cover is preventing what would otherwise be an even more rapid retreat because supraglacial debris cover can impart an important control on ice flow and glacier dynamics. In reducing melting during the ablation season, debris cover can reduce the infiltration of surface meltwater to the bed and thereby modify the subglacial hydrology and glacier flow. Reference MattsonMattson (2000), for example, found that debris cover on Dome Glacier in the Canadian Rocky Mountains apparently acts to subdue subglacial hydrology and stream-flow. Annual variances in volumetric meltwater discharge from this glacier were only around 1% during the study period, compared to 24% for the neighbouring and largely debris-free Athabasca Glacier. As a result, Reference MattsonMattson (2000) speculated that debris-covered glaciers may not be as sensitive to climate change as debris-free glaciers, and a similar conclusion was reached by Reference Thompson, Kirkbride and BrockThompson and others (2000), who found that the debris-covered Ghiacciaio del Miage in the Italian Alps had retreated less than debris-free glaciers in the same region. Exploration of the relationship between debris-cover thickness and extent, mass balance and frontal variations is clearly warranted, and field measurement of the surface energy balance on and adjacent to debris-covered ice should prove fruitful.
The retreat of the Caucasus glaciers and the increase in their supraglacial debris cover is also linked to the observed increase in the number and areal extent of supra- and proglacial lakes. Debris-covered snouts may become separated from more rapidly ablating ice further up-glacier and eventually stagnate. Ice cored (and non-ice-cored) moraine can then serve as an effective barrier to meltwater runoff and can lead to the development of proglacial and supraglacial lakes (cf. Reference Richardson and ReynoldsRichardson and Reynolds, 2000; Reference Chikita, Jha and YamadaChikita and others, 2001). The potential instability of such lakes may pose a serious threat to human activity downstream (Reference KonradKonrad, 1998; Reference Richardson and ReynoldsRichardson and Reynolds, 2000).
Our analysis indicates that both the total number of lakes and their surface area have increased dramatically in this region since 1985 (see Fig. 5; Table 2). This is similar to the situation reported by Reference AgetaAgeta and others (2000) who found that both supraglacial and proglacial lakes had expanded in the Bhutan Himalaya between the 1950s and 1998. Detailed field measurements of the lakes adjacent to Bashkara glacier (Fig. 5) confirm the regional trend, showing a progressive enlargement and deepening. Many of the lakes, as in the case of Bashkara glacier, lie immediately adjacent to debris-covered ice, either at the terminus of the glaciers or perched adjacent to their lateral margins. Thus, the recent thinning and retreat of the glaciers is leading to ponding of meltwater against relatively recent terminal and lateral moraines. Given that the mid-1980s to early 1990s were generally more favourable for Caucasus glaciers (i.e. less negative mass balance than current trends), it might be that many of the lakes have formed since the early 1990s during a period of more rapid retreat.
Continuation of this trend is therefore likely to lead to more lakes emerging in the region, and the progressive enlargement and filling of existing lakes. Once such lakes have developed, their thermal properties ensure that progressive expansion is achieved through the melting and subsidence of the dead ice beneath them (thermokarst) and the melting of adjacent ice, as well as thermally induced calving (Reference Chikita, Jha and YamadaChikita and others, 1999; Reference Sakai, Takeuchi, Fujita and NakawoSakai and others, 2000; Reference Kääb and HaeberliKääb and Haeberli, 2001). We suggest that it is these thermokarst processes that are leading to the observed expansion of the lakes adjacent to Bashkara glacier (Fig. 4). Additionally, supraglacial lakes may form at the up-glacier limit of the debris cover because this is where ablation is focused and may create a surface depression on the glacier surface (Reference KonradKonrad, 1998; Reference Naito, Nakawo, Kadota and RaymondNaito and others, 2000). The development of such lakes is also determined by the overall surface gradient of the glacier, with low surface slopes (<10˚) more favourable for lake development (Reference ReynoldsReynolds, 2000).
Given that many of the glacial catchments in the Caucasus feed directly into larger valleys which house small settlements and towns, this is a concern. Indeed, recently there has been a glacier-related rock/mudslide which led to major loss of life in this region (Haerberli and others, 2004). Although this was not attributable to a supra-/proglacial lake outburst, it emphasizes the close proximity of human activities to these glacial systems and their vulnerability to glacier-related hazards.
Conclusions
In this paper, we report local changes on six neighbouring glaciers undergoing retreat in the Adylsu valley, focusing specifically on the evolution of supraglacial debris cover and the evolution of supra- and proglacial lakes. We also extend our investigation of supra- and proglacial lakes beyond this valley to a much larger area of the central Caucasus. Mapping from satellite imagery (covering the period 1985– 2005) and field surveys (e.g. Reference Popovnin and RozovaPopovnin and Rozova, 2002) leads us to the following conclusions:
Glacier retreat and thinning is accompanied by a progressive and consistent increase in the percentage of supraglacial debris cover on each glacier, with the exception of one very small glacier where accurate mapping is more difficult
In general, supraglacial debris cover has increased 3–6% on each glacier and is characterized by a gradual migration of existing debris cover up-glacier
Glaciers in the Caucasus mountains appear to be undergoing a process of backwasting whereby the debris-covered snouts are largely stagnant, albeit in retreat, but ablation and thinning is focused at the up-glacier limit of the debris cover, rather than the terminus
Glacier thinning and shrinkage (in addition to permafrost melt) increases the likelihood of slope failure immediately adjacent to the glacier (including the accumulation area), leading to the addition of further debris on to glacier surfaces
The recent increase in glacier retreat and thinning has led to the ponding of meltwater in lakes impounded by recent (late 1980s?) terminal and lateral moraines. The total number of supra- and proglacial lakes in our wider study area has increased from 16 to 24, representing a 57% increase in total lake area coverage
The progressive increase in debris cover and glacial lake coverage is likely to exert a profound influence on the future response of these glaciers to climate change (Reference Sakai, Takeuchi, Fujita and NakawoSakai and others, 2000; Reference Thompson, Kirkbride and BrockThompson and others, 2000). Detailed measurement of the interactions between debris-cover thickness and extent and the surface energy balance is required to elucidate the links between debris cover and glacier mass balance. In addition, continued monitoring of supra- and proglacial lakes would appear to be a prudent precaution against potential glacier-related hazards (Reference Richardson and ReynoldsRichardson and Reynolds, 2000).
Acknowledgements
This work was funded by the School of Human and Environmental Science at The University of Reading and the Royal Society Joint Project Scheme (2005/R2-JP/H3088300). The ongoing field monitoring of Djankuat and Bashkara glaciers is supported by the Russian Foundation for Basic Research (06-05-64094a). We thank C. Huggel, an anonymous reviewer and the scientific editor, A. Kääb, for instructive comments on the manuscript.