PUFA are important components of cell membranes. Variations in the proportions of individual PUFA can alter the cell function by modulating the fluidity of the phospholipid bilayer, thus influencing the activity of integral membrane proteins( Reference Calder, Yaqoob and Harvey 1 ). PUFA can also act as substrates for cell signalling processes. Changes in the relative proportions of individual PUFA may modify cell function by changing the nature of lipid second messengers, including eicosanoids( Reference Wada, DeLong and Hong 2 ), diacylglycerol and phosphatidic acid( Reference Heung and Postle 3 , Reference Heung and Postle 4 ). For example, activated macrophages produce 2-series PG from arachidonic acid (20 : 4n-6) through the activity of cyclo-oxygenase but synthesise less biologically potent 3-series PG from EPA (20 : 5n-3)( Reference Wada, DeLong and Hong 2 ). Protein kinase C α has been shown to be preferentially activated by 1,2-dipalmitoyldiacylglycerol (DPD) compared with 1-stearoyl, 2-docosahexaenoyldiacylglycerol (SDD). However, activation of protein kinase C γ by SDD is greater compared with DPD( Reference Kamiya, Mizuno and Komenoi 5 ). PUFA can modify cell function at the level of gene transcription by acting as ligands for PPAR( Reference Forman, Chen and Evans 6 ), and recent findings suggest that PUFA can modify epigenetic processes( Reference Burdge and Lillycrop 7 ). Thus, maintenance of the phospholipid composition of cell membranes and, consequently, normal cell activity requires an adequate, timely supply of specific PUFA. These can be obtained pre-formed from the diet via the bloodstream. However, this may be a precarious strategy for maintaining tissue function because dietary choice and temporal variation in intake may limit the capacity of the diet to supply individual PUFA against changing demands; for example, during an immune response to infection.
Mammals can convert the essential fatty acids (EFA) linoleic acid (18 : 2n-6) and α-linolenic acid (18 : 3n-3) to longer-chain, more unsaturated fatty acids that are required for cell function. The purpose of this review is to assess whether hepatic and extra-hepatic PUFA syntheses are important for meeting demands for PUFA, primarily in humans.
Hepatic PUFA synthesis in mammals other than humans
Mammalian cell membranes contain C18, C20 and C22 PUFA, which are classified as n-6, n-3 or n-9 depending on the position of the first double bond from the methyl end of the hydrocarbon chain. The MUFA oleic acid (18 : 1n-9) can be synthesised de novo from non-lipid precursors by the activity of the fatty acid synthase complex followed by insertion of a double bond at Δ9 position by stearoyl-CoA desaturase( Reference Ichi, Kono and Arita 8 ). During dietary EFA deficiency, 18 : 1n-9 can be converted to Mead acid (20 : 3n-3) by the sequential activities of Δ6 desaturase, elongase 5 and Δ5 desaturase( Reference Ichi, Kono and Arita 8 ) (Fig. 1). EFA deficiency and 20 : 3n-9 synthesis are rare in humans and occur primarily in patients receiving artificial nutrition( Reference Barr, Dunn and Brennan 9 ). However, the precise biological role of conversion of 18 : 1n-9 to 20 : 3n-9 is not known. One possibility is that 20 : 3n-9 may, at least in part, compensate for decreased membrane fluidity due to reduced availability of PUFA for incorporation into the phospholipid bilayer. Alternatively, synthesis of 20 : 3n-9 involves, in part, enzymes that catalyse PUFA synthesis (Fig. 1) and so conversion of 18 : 1n-9 to 20 : 3n-9 may be an artefact of reduced flux of EFA through the PUFA synthesis pathway.
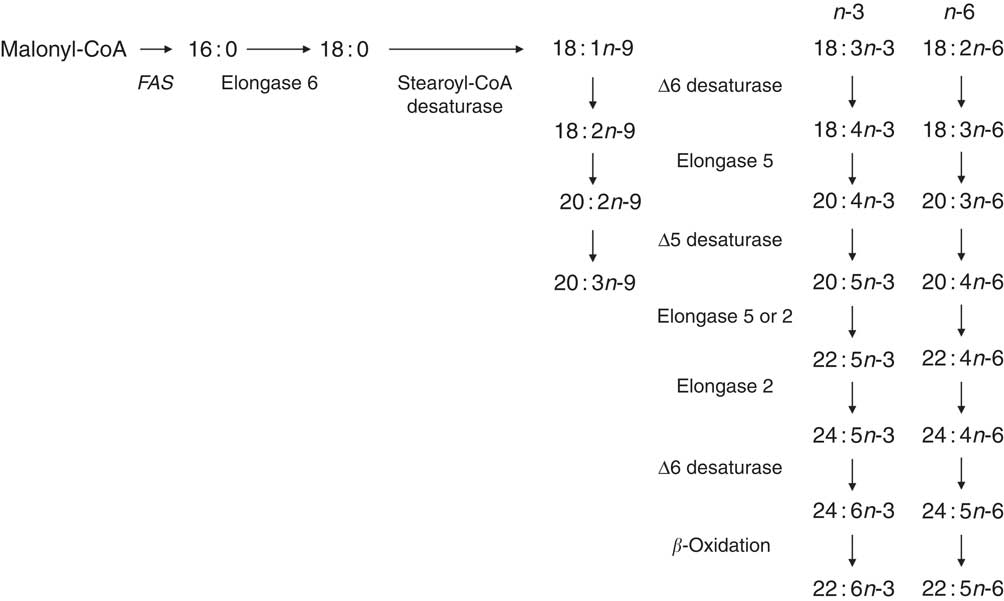
Fig. 1 The pathway for synthesis of longer-chain n-9 fatty acids overlaps in part with metabolic reactions involved in n-3 and n-6 PUFA biosynthesis. FAS, fatty acid synthase.
Mammals do not express Δ12 or Δ15 desaturases which catalyse the conversion of 18 : 1n-9 to 18 : 2n-6 and 18 : 2n-6 to 18 : 3n-3, respectively. Consequently mammals are dependent upon either consumption of pre-formed ≥20 carbon PUFA in their diet or conversion of 18 : 2n-6 and 18 : 3n-3 to longer-chain, more unsaturated fatty acids to meet their PUFA requirements. The general pathway for conversion of 18 : 2n-6 and 18 : 3n-3 to longer-chain, more unsaturated PUFA was elucidated in the 1960s by studies carried out using rodent liver( Reference Klenk and Mohrhauer 10 , Reference Mead 11 ) (Fig. 1). The first desaturation reaction, catalysed by Δ6 desaturase, is followed by malonyl-CoA-dependent carbon chain elongation by elongase 5 and then Δ5 desaturation to form 20 : 4n-6 and 20 : 5n-3 from 18 : 2n-6 and 18 : 3n-3, respectively (Fig. 1); 20 : 4n-6 can be converted to 22 : 4n-6 and 20 : 5n-3 to 22 : 5n-3 by the addition of two carbons by either elongase 5 or elongase 2 (Fig. 1). Although the first reaction catalysed by Δ6 desaturase is generally assumed to be rate limiting, the chain elongation reactions that follow the formation of 22 : 5n-3 and 22 : 4n-6 have also been proposed as the metabolic control points( Reference Gregory, Gibson and Cook-Johnson 12 ). Synthesis of 22 : 5n-6 and 22 : 6n-3 was originally proposed to involve Δ4 desaturation( Reference Innis 13 ). However, this view was superseded because of the apparent absence in rat liver microsomes of an enzyme with Δ4 desaturase activity and the demonstration that synthesis of 22 : 5n-6 and 22 : 6n-3 involves further chain elongation by elongase 2 to form the intermediates 24 : 4n-6 and 24 : 5n-3, followed by Δ6 desaturation and subsequent translocation of the products, 24 : 5n-6 and 24 : 6n-3, to peroxisomes. 22 : 5n-6 and 22 : 6n-3 are formed by the removal of two carbons by single cycle of β-oxidation( Reference Voss and Sprecher 14 – Reference Voss, Reinhart and Sankarappa 16 ). However, Park et al.( Reference Park, Park and Kothapalli 17 ) have shown that the MCF7 human breast cancer cell line, which does not express Δ6 desaturase activity, expresses Δ4 desaturase activity when transfected with fatty acid desaturase (FADS) 2 and FADS1, which encodes Δ6 and Δ5 desaturases, respectively. However, Δ4 desaturase activity remains to be demonstrated in wild-type, untransformed primary cells.
Hepatic PUFA biosynthesis in humans
In marked contrast to rodents, humans have limited capacity for conversion of EFA to longer-chain PUFA( Reference Plourde and Cunnane 18 , Reference Burdge 19 ). Studies using stable isotope tracer technology to assess whole body, in essence hepatic, interconversion of 18 : 3n-3 in men or mixed groups of men and women have shown consistently that conversion to 20 : 5n-3 is limited to <10% of the administered labelled 18 : 3n-3, and that only trace amounts of 22 : 6n-3 are formed (<0·05 % of ingested 18 : 3n-3)( Reference Plourde and Cunnane 18 – Reference Hussein, Ah-Sing and Wilkinson 20 ). Moreover, conversion of [13C]18 : 3n-3 to 22 : 6n-3 has been shown to be independent of relative intakes of 18 : 3n-3 and 18 : 2n-6( Reference Hussein, Ah-Sing and Wilkinson 20 ). Thus, the contribution of PUFA synthesis to 22 : 6n-3 formation appears to be negligible, although one study in men estimated conversion of 18 : 3n-3 to 22 : 6n-3 to be approximately 4 % of the administered dose( Reference Emken, Adlof and Gulley 21 ). It is not known why 22 : 6n-3 synthesis was greater in this study compared with others. Conversion of [13C]18 : 2n-6 to 20 : 4n-6 in men has also been shown to be extremely limited (approximately <0·2 % of ingested labelled fatty acid)( Reference Hussein, Ah-Sing and Wilkinson 20 ).
There are major challenges in interpreting the concentrations of stable isotope-labelled fatty acids in blood in terms of EFA interconversion, which have been reviewed elsewhere( Reference Burdge 19 ). However, such estimates are consistent with the effect of increased 18 : 3n-3 intake (between 4 and 20 g/d) on the proportions of 20 : 5n-3, 22 : 5n-3 and 22 : 6n-3 in blood and cell lipids in men or in mixed groups of men and postmenopausal women( Reference Baker, Miles and Burdge 22 , Reference Burdge and Calder 23 ). These studies showed that greater 18 : 3n-3 intake was associated with increased proportions of 20 : 5n-3 and 22 : 5n-3. Based on data summarised in Burdge & Calder( Reference Burdge and Calder 23 ), 18 : 3n-3 intake predicted 66% of the variation in the proportion of 20 : 5n-3 in plasma phospholipids such that doubling 18 : 3n-3 intake approximately doubled the change in 20 : 5n-3 status. However, there was no significant increase in the proportion of 22 : 6n-3 and in four of nine studies 22 : 6n-3 status decreased during 18 : 3n-3 supplementation to below the baseline level( Reference Burdge and Calder 23 ). In addition, dietary supplementation with 18 : 3n-3, 18 : 4n-3 or 20 : 5n-3 increased the proportions of 20 : 5n-3 and 22 : 5n-3 in blood but was accompanied by a statistically non-significant decrease in 22 : 6n-3 status( Reference James, Ursin and Cleland 24 ). One possible explanation for the reduction in 22 : 6n-3 status is that the rate of 22 : 6n-3 synthesis was less than the rate of turnover.
Women and females of other species appear to have greater capacity for synthesis and higher 22 : 6n-3 status than their male counterparts. Conversion of [13C]18 : 3n-3 to 20 : 5n-3 in women of reproductive age has been estimated to be 21% (compared with <10 % in men) and conversion to 22 : 6n-3, 9% (compared with <0·05 % in men)( Reference Burdge and Wootton 25 , Reference Burdge, Jones and Wootton 26 ). This observation is supported by the findings of a systematic review of fifty-one observational studies that showed the proportions of 20 : 4n-6 and 22 : 6n-3 in blood phospholipids were typically 20 % higher in women than in men( Reference Lohner, Fekete and Marosvolgyi 27 ) and also by a recent kinetic modelling study in overweight women( Reference Lin, Hibbeln and Domenichiello 28 ). Female rodents( Reference Burdge, Slater-Jefferies and Grant 29 , Reference Childs, Romeu-Nadal and Burdge 30 ) and great tits (Parus major)( Reference Isaksson, Hanson and Burdge 31 ) also have higher 22 : 6n-3 status compared with males, which suggests this difference in PUFA status and metabolism between sexes may have been conserved during evolution. Oestrogen is an agonist for PUFA synthesis( Reference Burdge and Wootton 25 , Reference Giltay, Gooren and Toorians 32 ) and hence may be responsible for greater PUFA synthesis in females than in males. However, studies in postmenopausal women, in primary human hepatocytes and in HepG2 hepatocarcinoma cells, suggest that progesterone may also be involved( Reference Giltay, Duschek and Katan 33 , Reference Sibbons, Brenna and Lawrence 34 ).
Dietary supplementation with 9·5 g/d 18 : 3n-3 induced a greater increase in the proportion of 20 : 5n-3 in women (age 53·5 years) compared with men (age 50·5 years), but there was no difference between sexes in the proportion of 22 : 6n-3 at the end of the study( Reference Childs, Kew and Finnegan 35 ). Furthermore, the change in plasma 20 : 5n-3 status was associated negatively with age in women but not in men( Reference Childs, Kew and Finnegan 35 ). These findings suggest that, at least in women of post-reproductive age, the contribution of PUFA biosynthesis to plasma PUFA status is similar to men. Whether this pathway is more important for meeting the demands for PUFA of younger women has yet to be determined.
Hepatic PUFA biosynthesis in pregnancy
Pregnancy involves increased demands on the mother for PUFA, to meet both her requirements and those of her fetus. The human fetus accumulates 22 : 6n-3, beginning in the second trimester, particularly into the central nervous system( Reference Innis 13 , Reference Kuipers, Luxwolda and Offringa 36 ) and deficits in 22 : 6n-3 incorporation into developing brain have been associated with cognitive deficits in non-human primates( Reference Reisbick, Neuringer and Hasnain 37 ). The concentration of 22 : 6n-3, but not 20 : 4n-6, has been shown to increase in plasma phosphatidylcholine (PC) during the second trimester of pregnancy, specifically an increase in PC16:0/22:6( Reference Postle, Al and Burdge 38 ), which precedes the period of prenatal 22 : 6n-3 accumulation into fetal brain( Reference Kuipers, Luxwolda and Offringa 36 ). One interpretation is that such adaptation of maternal liver lipid metabolism may facilitate adequate supply of 22 : 6n-3 to the fetal brain( Reference Postle, Burdge and Al 39 ). Total plasma 22 : 6n-3 concentration has also been shown to increase between 18 and 29 d post-conception in women undergoing assisted reproduction( Reference Meyer, Onyiaodike and Brown 40 ). This change in plasma PC composition has been shown in rats to involve changes in the composition of the maternal hepatic diacylglycerol pool destined for PC synthesis and decreased acyl remodelling of sn-1 16 : 0 to sn-1 18 : 0 PC molecular species( Reference Burdge, Hunt and Postle 41 ) and to be accompanied by increased liver Fads1 and Fads2 mRNA expression( Reference Burdge, Slater-Jefferies and Grant 29 , Reference Childs, Hoile and Burdge 42 ), possibly by the action of progesterone( Reference Childs, Hoile and Burdge 42 ).
Despite evidence of coordinated changes in maternal hepatic PUFA metabolism, the contribution, if any, of such adaptations to meeting maternal and fetal demands for PUFA is not known. In pregnant women, maternal plasma PC 22 : 6n-3 concentration tracks during gestation such that the level in early pregnancy (11–17 weeks) predicts 21 % of the variation in late pregnancy (35 weeks)( Reference Crozier, Sibbons and Fisk 43 ). Therefore, it may be expected that position in the rank order of maternal 22 : 6n-3 status, which is a proxy for 22 : 6n-3 availability to the developing fetus, would influence the cognitive function of the child. However, maternal plasma 22 : 6n-3 concentration during pregnancy has not been associated with intelligence quotient (IQ) or markers of executive cognitive function at ages 4 or 6–7 years( Reference Crozier, Sibbons and Fisk 43 , Reference Brouwer-Brolsma, van de Rest and Godschalk 44 ), cognition at 4 years( Reference Ghys, Bakker and Hornstra 45 ) or 7 years( Reference Bakker, Ghys and Kester 46 ), nor with problem behaviour at 7 years( Reference Krabbendam, Bakker and Hornstra 47 ). Moreover, 22 : 6n-3 concentration has been shown to be approximately 32 % lower in umbilical cord blood and 62 % lower in breast milk from vegetarian pregnancies compared with omnivores( Reference Sanders and Reddy 48 ) which suggests that metabolic adaptations that increase 22 : 6n-3 concentration in maternal blood during pregnancy are insufficient to compensate for low intake of 22 : 6n-3 in vegetarians( Reference Burdge, Tan and Henry 49 ). One possibility is that maternal adaptations to hepatic PUFA metabolism may be involved in the adjustment of the mother’s tissues to pregnancy rather than contributing directly to the PUFA supply and development of the fetus. For example, in the rat increased maternal plasma PC16:0/22:6 concentration occurs in late pregnancy and may facilitate supply of 22 : 6n-3 for incorporation into milk( Reference Burdge, Hunt and Postle 41 ).
Extra-hepatic PUFA biosynthesis
Tissues can acquire pre-formed ≥C20 PUFA from blood which are derived from the diet or from hepatic synthesis. These PUFA are assimilated primarily by hydrolysis of TAG and uptake of fatty acids at the surface of the vascular endothelium via the actions of lipoprotein lipase and CD36 or uptake via CD36 from the NEFA pool( Reference Goldberg, Eckel and Abumrad 50 ). The Na-dependent transporter Mfsd2a has also been shown to transport lysoPC containing 22 : 6n-3 or 18 : 1n-9 selectively to the brain( Reference Guemez-Gamboa, Nguyen and Yang 51 , Reference Nguyen, Ma and Shui 52 ), skin fibroblasts( Reference Moore, Hurt and Yoder 53 ), retina( Reference Wong, Chan and Cazenave-Gassiot 54 ) and placenta( Reference Prieto-Sanchez, Ruiz-Palacios and Blanco-Carnero 55 ). At least some extra-hepatic tissues express key genes involved in PUFA synthesis or have been shown to convert EFA to longer-chain PUFA. One possible explanation for local intracellular PUFA synthesis is that the pool of pre-formed ≥20 carbon PUFA in blood is insufficient to meet the demands of specific tissues, possibly in terms of quantity, composition and/or timing and that obtaining specific PUFA is closely associated with fundamental processes in the function of those cells.
FADS2 mRNA expression has been detected in human heart, brain, lung, liver, skeletal muscle, kidney, pancreas and placenta, while FADS1 was found to be expressed primarily in liver, lung, brain and heart with only trace expression in pancreas, kidney, skeletal muscle and placenta( Reference Cho, Nakamura and Clarke 56 ). However, the relative expression of FADS2 to other genes involved in the pathway differs between reports( Reference Cho, Nakamura and Clarke 56 , Reference Cho, Nakamura and Clarke 57 ). In general, human brain and liver expressed the highest level of FADS2 mRNA, followed by heart>placenta≡lung>kidney>skeletal muscle>spleen but was essentially undetectable in pancreas( Reference Cho, Nakamura and Clarke 56 , Reference Cho, Nakamura and Clarke 57 ). FADS1, FADS2, ELOVL5 and ELOVL4 have also been shown to be expressed in peripheral blood mononuclear cells (PBMC)( Reference Chisaguano, Montes and Perez-Berezo 58 , Reference Sibbons, Irvine and Pérez-Mojica 59 ). Moreover, PUFA biosynthesis has been reported in femoral artery( Reference Kelsall, Hoile and Irvine 60 ), vascular smooth muscle (VSM) cells( Reference Irvine, Lillycrop and Fielding 61 ), testis( Reference Albert, Rhamy and Coniglio 62 ), umbilical vein( Reference Rosenthal, Garcia and Jones 63 ), leucocytes( Reference Sibbons, Irvine and Pérez-Mojica 59 , Reference Chapkin, Miller and Somers 64 – Reference Chapkin and Coble 66 ) and a number of neoplastic and non-cancerous mammary epithelial cell lines( Reference Grammatikos, Subbaiah and Victor 67 , Reference Grammatikos, Subbaiah and Victor 68 ). There is no evidence that extra-hepatic cells secrete newly synthesised PUFA. Thus, the expression of genes involved in PUFA synthesis and/or PUFA synthesis in extra-hepatic tissues suggests that capacity for EFA interconversion may serve a different purpose to that in the liver. However, relatively few studies have investigated the role of PUFA synthesis in cell function. Nevertheless, emerging findings discussed below suggest that local PUFA synthesis is required for the normal function of at least some cell types.
PUFA biosynthesis in leucocytes
Leucocytes can convert 18 : 3n-3 and 18 : 2n-6 to longer-chain, more unsaturated PUFA, although the extent of such conversion appears to differ between types of immune cells and with the activation state of the cells. Radiolabelled 18 : 2n-6 can be converted to 20 : 2n-6 in quiescent murine macrophages without detectable further desaturation or elongation( Reference Chapkin, Somers and Erickson 69 ). Incubation of murine peritoneal macrophages with the calcium ionophore A23187, phorbol myristate acetate, or zymosan did not induce further interconversion of 20 : 2n-6( Reference Chapkin, Somers and Erickson 69 ). Furthermore, [14C]18 : 3n-6 can be converted to 20 : 3n-6, but not to 20 : 4n-6, in stimulated murine macrophages( Reference Chapkin and Coble 66 ). Absence of detectable 20 : 4n-6 suggests that murine macrophages lack Δ5 desaturase activity.
In contrast to macrophages, mitogen stimulation increased the proportions of 18 : 1n-9, 22 : 5n-3 and 22 : 6n-3 and decreased the proportion of n-6 PUFA in T-cell membranes( Reference Anel, Naval and Gonzalez 70 ). This suggests remodelling of membrane phospholipids and/ or increased synthesis or uptake of specific fatty acids including longer-chain n-3 PUFA. Mitogen stimulation of human PBMC increased Δ9, Δ6 and Δ5 desaturase activities, which was associated with increased interconversion of [14C]18 : 0 to 18 : 1n-9 and of [14C]18 : 2n-6 and [14C]18 : 3n-3 to triene and tetriene PUFA( Reference Anel, Naval and Gonzalez 71 ). The authors noted that it was uncertain whether such capacity was sufficient to account for the changes in membrane composition that accompany T-cell activation. Moreover, incubation of human PBMC with 18 : 2n-6 or 18 : 3n-3 followed by mitogen stimulation increased the incorporation of these fatty acids into the cells( Reference Anel, Naval and Gonzalez 71 ). Incubation of mitogen-stimulated rat lymph node lymphocytes with 18 : 3n-3 increased the proportions of 18 : 3n-3, 20 : 5n-3 and 22 : 5n-3 but not 22 : 6n-3 in membrane phospholipids( Reference Calder, Yaqoob and Harvey 72 ) which suggests that at least some of the changes in membrane composition in activated T cells may involve endogenous PUFA synthesis, while other, such as increased 22 : 6n-3 content, may represent selective uptake from their environment. Although incubation with 18 : 2n-6 increased the proportion of this fatty acid in T-cell phospholipids, there was only a small, non-significant increase in the 20 : 4n-6 content( Reference Calder, Yaqoob and Harvey 72 ). The apparent inconsistency in the conversion of 18 : 3n-3 and 18 : 2n-6 was not explained by the authors. These findings suggest that, in contrast to macrophages, human and rat lymphocytes express Δ6 and Δ5 desaturase, and that elongase expression is up-regulated in activated cells. Increased membrane fluidity induced by increasing the proportion of unsaturated fatty acids in membrane phospholipids has been suggested a possible mechanism by which unsaturated fatty acids could inhibit immune cell function by modifying the membrane protein activity( Reference Calder, Yaqoob and Harvey 72 ). Moreover, increasing the synthesis of PUFA substrates may facilitate the production of pro-resolving mediators and hence attenuate the immune response( Reference Halade, Black and Verma 73 ).
A recent study of PUFA biosynthesis in human PBMC confirmed that mitogen stimulation induced increased incorporation of [13C]18 : 3n-3 into cell lipids and conversion to longer-chain, more unsaturated fatty acids( Reference Sibbons, Irvine and Pérez-Mojica 59 ). This was accompanied by marked up-regulation of FADS2, FADS1, ELOVL5 and ELOVL4 mRNA expression( Reference Sibbons, Irvine and Pérez-Mojica 59 ). The first two reactions were chain elongation of 18 : 3n-3 to 20 : 3n-3 by an unidentified elongase followed by Δ8 desaturation of 20 : 3n-3 to 20 : 4n-3, essentially the reverse of the initial reactions of the pathway reported in liver( Reference Voss and Sprecher 14 , Reference Sibbons, Brenna and Lawrence 34 ) (Fig. 2). The first Δ6 desaturation in liver has been shown to be rate limiting( Reference Rodriguez, Sarda and Nessmann 74 ) and to preferentially catalyse conversion of 18 : 3n-3 compared with 18 : 2n-6( Reference Rodriguez, Sarda and Nessmann 74 ). Thus, one implication of the apparent substitution of the initial Δ6 desaturation with elongase activity is that the regulation of the PUFA synthesis pathway in PBMC may differ from that of other tissues. For example, elongases have been shown to differ in substrate preference in terms of chain length and level of unsaturation such that elongase 5 catalyses elongation of 18- and 20-carbon PUFA, while elongase 2 exhibits marked preference of 20- and 22-carbon PUFA( Reference Gregory, Gibson and Cook-Johnson 12 ). Both of these enzymes preferentially catalyse conversion of n-3 compared with equivalent n-6 PUFA( Reference Gregory, Gibson and Cook-Johnson 12 ). If so, this may have implications for understanding the influence of dietary fatty acids on immune function. The protein product of the baboon Fads2 gene has been associated with Δ8 desaturase activity when transfected into yeast cells, although Δ6 desaturase activity predominated( Reference Park, Kothapalli and Lawrence 75 ). Thus, it is possible that Δ8 desaturase activity in PBMC was also associated with the protein encoded by the FADS2 gene, although no Δ6 desaturase activity was detected( Reference Sibbons, Irvine and Pérez-Mojica 59 ). Moreover, Δ4-desaturase activity has been demonstrated in MCF7 breast cancer cells transfected with FADS2 that do not normally express this gene( Reference Park, Park and Kothapalli 17 ). Thus, the desaturase activity of the FADS2 protein product may depend upon the cellular environment in which it is expressed. ELOVL2 was not expressed in PBMC and the PUFA synthesis pathway was truncated after synthesis of 22 : 5n-3( Reference Sibbons, Irvine and Pérez-Mojica 59 ).

Fig. 2 The proposed pathway for PUFA biosynthesis linked to proliferation of T lymphocytes( Reference Cho, Nakamura and Clarke 57 ). Dashed arrows indicate putative reactions. VLCPUFA, very long-chain PUFA.
ELOVL4 catalyses the conversion of ≥C20 PUFA, but not 22 : 6n-3, to PUFA with chain length C24–C38( Reference Agbaga, Brush and Mandal 76 – Reference Suh and Clandinin 79 ), which are involved in the formation and structure of lipid rafts required for T-cell signalling( Reference Iwabuchi, Nakayama and Iwahara 80 ). Thus, it has been suggested that truncation of the PUFA synthesis pathway after 22 : 5n-3 and up-regulation of ELOVL4 may provide very long-chain PUFA required for T-cell activation( Reference Sibbons, Irvine and Pérez-Mojica 59 ). Moreover, a lipoxygenase-derived metabolite of 20 : 3n-9 can modulate leukotriene B4 synthesis, and hence neutrophil activation, via inhibition of leukotriene A hydrolase( Reference Cleland, James and Proudman 81 ). It is not known whether 20 : 3n-3 can also be converted into bioactive metabolites but demonstration of such activity may suggest an additional mechanism to link Δ8 desaturation to immune function. Of potential importance to understanding the role of PUFA synthesis in stimulated T cells, pharmacological inhibition of the FADS2 protein reduced mitogen-induced T-cell proliferation( Reference Sibbons, Irvine and Pérez-Mojica 59 ). Thus, PUFA synthesis appears to be involved in the regulation of T-cell proliferation possibly by providing substrates for the assembly of lipid microdomains and/or through the formation of novel lipid mediators (Fig. 2).
PUFA synthesis in cancer cells
PUFA synthesis has been described in several cancer cell types. One recent study compared PUFA synthesis in Jurkat T-cell leukaemia cells with PBMC( Reference Sibbons, Irvine and Pérez-Mojica 59 ). The findings showed that interconversion of [13C]18 : 3n-3 was constitutive and 17-fold greater in Jurkat cells than in mitogen-stimulated PBMC. FADS2, FADS1, ELOVL4 and ELOVL5 expression was also up to 17-fold greater in Jurkat cells than in stimulated PBMC, and ELOVL2 was expressed which is consistent with the conversion of 18 : 3n-3 to 22 : 6 in Jurkat cells. These findings suggest that oncogenic transformation may disrupt PUFA synthesis at the level of transcription at several points in the pathway. The major product of PUFA synthesis in Jurkat cells was 22 : 5n-3 rather than 20 : 3n-3, which was the major fatty acid synthesised from 18 : 3n-3 in PBMC. Jurkat cells expressed both Δ8 and Δ6 desaturase activities, while PBMC only expressed Δ8 desaturase activity. Furthermore, inhibition of the FADS2 protein did not significantly alter Jurkat cell proliferation( Reference Sibbons, Irvine and Pérez-Mojica 59 ). Stimulation of PBMC induced hypermethylation of a 600-bp region of the FADS2 promoter. In contrast, this putative regulatory region was hypomethylated in Jurkat cells( Reference Sibbons, Irvine and Pérez-Mojica 59 ). Together, these findings suggest that PUFA synthesis and its role in regulating lymphocyte cell proliferation is disrupted in Jurkat cells.
The activity of Δ6 desaturase and the expression of FADS2 mRNA were shown to be substantially greater in murine xenografts of B16 melanoma and Lewis lung carcinoma (LLC) cells compared with the normal tissue( Reference He, Qu and Wan 82 ). FADS2 RNAi knockdown inhibited proliferation and reduced tumour size in both B16 and LLC tumours( Reference He, Qu and Wan 82 ) which suggests that PUFA synthesis may be involved in the regulation of mitosis in these cells. The ratio of the sum of n-6 PUFA to 18 : 2n-6 in primary breast cancer tissue, a proxy marker of PUFA synthesis, was greater than that in uninvolved tissues( Reference Pender-Cudlip, Krag and Martini 83 ) and higher in more aggressive oestrogen receptor-negative (ER–) tumours compared with ER+ tumours( Reference Pender-Cudlip, Krag and Martini 83 ).
The absence of FADS2 expression in MCF7 breast carcinoma cells results in the synthesis by Δ5 desaturase of 5,11,14–20:3 and 5,11,14,17–20:4 which lack the 8–9 double bond of their eicosanoid substrate counterparts 20 : 4n-6 and 20 : 5n-3( Reference Park, Kothapalli and Lawrence 84 ). This suggests a mechanism by which deletion of FADS2 during malignant transformation may lead to disruption of cellular signalling and the production of novel second messengers( Reference Park, Kothapalli and Lawrence 84 ). Furthermore, activation of the c-Ha-ras oncogene, but not the c-Ha-ras pro-oncogene, in spontaneously immortalised MCF10A mammary epithelial cells resulted in the loss of capacity for Δ6 and Δ4 desaturation( Reference Grammatikos, Harvey and Subbaiah 85 ).
Together these studies suggest that altered regulation of PUFA synthesis is a characteristic of at least some types of cancer, which may be linked to their proliferative capacity. This is consistent with the findings of studies that show reduction in proliferation and/or induction of apoptosis of cancer cells treated with 22 : 6n-3( Reference Liu and Ma 86 – Reference Burdge, Rodway and Kohler 89 ).
PUFA synthesis in spermatogenesis
Human sperm is highly enriched in 22 : 6n-3. Morphologically normal sperm cells contain approximately 35 % 22 : 6n-3, while the proportion of 20 : 4n-6 is approximately 10·5 %( Reference Lenzi, Picardo and Gandini 90 ). The presence of 22 : 6n-3 in sperm membrane phospholipids has been associated with cell motility, membrane fusion and the synthesis of second messengers and is consistent with the presence of high levels of antioxidant defence mechanisms( Reference Lenzi, Picardo and Gandini 90 ). Experimental EFA deficiency in rats can induce degeneration of seminiferous tubules and reduced sperm formation and fertility( Reference Leath, Northop and Harrison 91 ). Moreover, feeding male Fads2 null mice a diet containing 18 : 3n-3 and 18 : 2n-6, without longer-chain PUFA, induced 74 % reduction in the proportion of 22 : 6n-3 and 92 % decrease in the relative amount of 20 : 4n-6 (92%) in testis( Reference Roqueta-Rivera, Stroud and Haschek 92 ). This was accompanied by arrested spermatogenesis and degradation of seminiferous tissue( Reference Roqueta-Rivera, Stroud and Haschek 92 ). Dietary supplementation of Fads2 null mice with 22 : 6n-3 restored spermatogenesis and fertility to levels equivalent to wild-type mice( Reference Roqueta-Rivera, Stroud and Haschek 92 ). This suggests that PUFA synthesis is important for fertility in mice fed a diet without longer-chain PUFA. However, because the knockout was not tissue-specific, it is not possible to conclude whether the activity of the PUFA synthesis pathway in testis is involved. However, there is some evidence for local PUFA synthesis in testis. Microsomes from human testis can convert [14C]18 : 2n-6 to 20 : 3n-6 (88 %) and 20 : 4n-6 (12 %) but not 18 : 3n-6( Reference Albert, Rhamy and Coniglio 62 ), indicating that the initial reactions were elongation of 18 : 2n-6 to 20 : 2n-6, followed by Δ8 desaturation to form 20 : 3n-6 followed by Δ5 desaturation to 20 : 4n-6. This pathway has also been reported in rat testis( Reference Albert and Coniglio 93 ). Moreover, the proportion of 22 : 6n-3 in testis from humans and from Rhesus macaques increases during puberty and remains essentially unchanged during adulthood( Reference Coniglio, Grogan and Rhamy 94 , Reference Connor, Lin and Neuringer 95 ). One possible mechanism is that testicular 22 : 6n-3 synthesis increases during puberty to meet the requirements for 22 : 6n-3 during spermatogenesis. If so, this suggests that regulation of PUFA synthesis in testis differs from hepatocytes in which testosterone has no significant effect on 22 : 6n-3 synthesis( Reference Childs, Kew and Finnegan 35 ). However, the contribution of testicular PUFA synthesis to male fertility has yet to be determined.
PUFA synthesis in arteries
Bovine aortic endothelial cells have been shown to retroconvert 22 : 6n-3 to 22 : 5n-3 and 20 : 5n-3( Reference Hadjiagapiou and Spector 96 ) and 22 : 4n-6 to 20 : 4n-6( Reference Mann, Kaduce and Figard 97 ). Elongation of [14C]20 : 5n-3 to 22 : 5n-3 and of [14C]20 : 4n-6 to 22 : 4n-6 has also been shown in human vascular endothelial cells( Reference Rosenthal, Garcia and Jones 63 ), although the role of PUFA synthesis in endothelial cell function has not been reported. Phenylephrine stimulation of isolated rat aortae increased Fads1 and Fads2 mRNA expression compared with unstimulated vessels( Reference Kelsall, Hoile and Irvine 60 ). Moreover, treatment of isolated rat aortae or human femoral artery with the FADS2 protein inhibitor SC26196 or rat aortae with the Δ5 desaturase inhibitor sesamin reduced phenylephrine-induced vasoconstriction and secretion by rat aortae of pro-constriction eicosanoids PGF2α , PGE2 and thromboxane A2( Reference Kelsall, Hoile and Irvine 60 ). Thus, PUFA synthesis, which was localised to VSM rather than the endothelium( Reference Kelsall, Hoile and Irvine 60 ), appears to be involved in regulating vasoconstriction. Mouse aortae, immortalised murine VSM (MOVAS) cells and human primary aortic VSM cells express Fads1, Fads2 and Elovl5 but not Elovl2 ( Reference Irvine, Lillycrop and Fielding 61 ). This is consistent with the apparent absence of conversion of 22 : 4n-6 to 22 : 5n-6, in MOVAS cells( Reference Irvine, Lillycrop and Fielding 61 ). Since Elovl2 was also not expressed in PBMC, it is possible that suppression of Elovl2 transcription is a characteristic of at least some excitable tissues although the precise function may differ. The absence of Elovl2 expression did not appear to involve hypermethylation of the promoter( Reference Irvine, Lillycrop and Fielding 61 ). Inhibition of Δ6 or Δ5 desaturase significantly reduced phenylephrine-mediated Ca release and secretion of PGE2 and PGF2α in MOVAS cells( Reference Cho, Nakamura and Clarke 57 ). The findings suggest that α 1-adrenergic receptor-mediated vasoconstriction involves activation of PUFA synthesis acting via regulation of endogenous Ca2+ release and secretion of specific vaso-active eicosanoids. One possible mechanism that has been suggested to explain the link between PUFA synthesis and vasoconstriction is activation of protein kinase C ζ and inhibition of myosin light chain phosphorylase by newly synthesised 20 : 4n-6( Reference Irvine, Lillycrop and Fielding 61 ).
Polymorphisms in genes involved in PUFA biosynthesis
The region of human chromosome 11 that contains the FADS1 and FADS2 genes in a head-to-head orientation (11q12.2–q13.1), together with FADS3( Reference Schaeffer, Gohlke and Muller 98 ), contains 4391 variants, of which 217 can result in amino acid changes( Reference Minihane 99 ) and hence potentially alter enzyme activity. This region has been identified as a cancer ‘hotspot locus’( Reference Park, Kothapalli and Lawrence 84 , Reference Marquardt, Stohr and White 100 ). Several genome–wide association studies have reported associations between polymorphisms in genes associated with PUFA synthesis and the concentrations of PUFA in blood. A meta-analysis of 8866 individuals from five cohorts showed that minor alleles of SNP in FADS2 and FADS1 were associated with a higher level of 18 : 3n-3 and a lower level of 20 : 5n-3, 22 : 5n-3 and 22 : 6n-3, while minor alleles of ELOVL2 were associated with higher proportions of 20 : 5n-3 and 22 : 5n-3 and a lower proportion of 22 : 6n-3( Reference Lemaitre, Tanaka and Tang 101 ). The variation in the proportions of n-6 PUFA in plasma phospholipids explained by eleven locus haplotypes has been shown to be 18 : 2n-6, 9·2 %; 18 : 3n-6, 7·9 %; 20 : 2n-6, 12·3 %; 20 : 3n-6, 10·8 % and 20 : 4n-6, 28·5 %( Reference Mann, Kaduce and Figard 97 ). In contrast, there were only limited associations with n-3 PUFA (18 : 3n-3, 5·4 %; 20 : 5n-3, 6·9 %; 22 : 5n-3, 5·1 %; 22 : 6n-3, 2·9 %)( Reference Schaeffer, Gohlke and Muller 98 ). However, whether these polymorphisms alter tissue function is not known. Moreover, interpretation of such associations may be confounded by contributions of hepatic phospholipid biosynthesis by the cytosine diphosphate choline and phosphatidylethanolamine (PE) N-methylation pathways to the fatty acid composition of plasma phospholipids because human( Reference Pynn, Henderson and Clark 102 ) and rat( Reference Burdge, Hunt and Postle 41 ) liver selectively synthesise 20 : 4n-6- and 22 : 6n-3-containing PC by PE N-methylation. One possible explanation for the greater effect of FADS polymorphisms on the proportions of n-6 PUFA for n-3 PUFA is the typically greater intake of 18 : 2n-6 than 18 : 3n-3( Reference Burdge and Calder 103 ) and consequently greater flux of n-6 PUFA through the PUFA synthesis pathway than 18 : 3n-3. Women carrying FADS1 and FADS2 minor alleles had lower proportions of 20 : 4n-6 and 22 : 5n-3 in their plasma and in breast milk, although the effect on the development of their infants and children was not assessed( Reference Xie and Innis 104 ). Thus, mutations in genes involved in PUFA synthesis are associated with variations in plasma PUFA status, which suggests that hepatic PUFA synthesis can contribute to the levels of circulating PUFA. However, these findings have not been used to estimate the magnitude of this contribution. Carriers of the minor allele (deletion) of a 22 bp FADS2 insertion–deletion mutation had lower expression of FADS1 in lymphocytes( Reference Reardon, Zhang and Kothapalli 105 ) and lower 20 : 4n-6 status compared with the major insertion allele( Reference Kothapalli, Ye and Gadgil 106 ). The frequency of the insertion/insertion allele was greater in populations with a tradition of vegetarian diet compared with Western omnivorous populations who had a higher frequency of the deletion/deletion genotype. This has been suggested as a possible adaption to low intakes of pre-formed longer-chain PUFA( Reference Kothapalli, Ye and Gadgil 106 ).
To date, the effect of polymorphisms in genes involved in PUFA synthesis on tissue function has not been investigated directly, although such studies may provide novel insights into tissue function and disease aetiology. However, there is some evidence that individuals carrying minor FADS1–FADS2 alleles that were associated with lower 20 : 4n-6 status have a lower prevalence of allergic disease which may be attributable to a reduction in the availability of 20 : 4n-6 for the synthesis of pro-inflammatory eicosanoids( Reference Schaeffer, Gohlke and Muller 98 ). However, since PUFA synthesis can regulate T-cell activation and proliferation( Reference Sibbons, Irvine and Pérez-Mojica 59 ), it is also possible that reduced desaturase activity may constrain the activity of the PUFA synthesis pathway, thus limiting cell proliferation and the immune response.
Conclusions
Humans have limited capacity for hepatic conversion of EFA to longer-chain, more unsaturated fatty acids( Reference Plourde and Cunnane 18 , Reference Burdge 19 ). Current knowledge about the contribution of EFA interconversion to meeting demands for PUFA in humans is based primarily on the results of whole-body tracer studies that are difficult to interpret in terms of net contribution of PUFA status and on dietary intervention trials that use amounts of EFA that exceed those found in typical western omnivorous diets( Reference Plourde and Cunnane 18 , Reference Burdge 19 ). The relatively few studies that report PUFA status in individuals consuming vegetarian diets that exclude pre-formed ≥C20 PUFA suggest that hepatic PUFA synthesis is unable to supply sufficient longer-chain PUFA to maintain status equivalent to that achieved by consuming an omnivorous diet( Reference Burdge, Tan and Henry 49 ). However, whether the levels of PUFA found in omnivores are optimal or whether they exceed requirements for maintaining health is uncertain. It is possible that humans can function adequately with PUFA status found in vegetarians, which primarily reflects PUFA synthesis from EFA, as may be implied by the health benefits of such diets( Reference Burdge, Tan and Henry 49 ). If so, it may be appropriate to question whether recommendations for intake of n-3 PUFA are higher than needed for optimal tissue function as opposed to pharmacological intakes that may prevent specific lifestyle-associated diseases. Overall, the contribution of PUFA synthesis to meeting the demands for ≥C20 PUFA of individuals is uncertain and requires further consideration.
A number of extra-hepatic tissues express genes involved in PUFA synthesis or have been shown to be capable of converting EFA to longer-chain PUFA. The details of such processes differ between cell types that suggest extra-hepatic PUFA synthesis is involved in and tailored to a particular cell phenotype. However, there are important gaps in knowledge regarding the precise role of PUFA synthesis in each cell type. Nevertheless, the findings of experiments that have attempted to address this problem suggest PUFA synthesis can be involved in key regulatory processes such as cell proliferation and that oncogenic transformation may result in disruption of the link between PUFA synthesis and cell function.
In conclusion, the biological importance of extra-hepatic PUFA biosynthesis remains unclear and there are major gaps in knowledge about PUFA synthesis in humans. Moreover, it may be that the primary role of PUFA biosynthesis within specific tissues lies in local intracellular provision of specific PUFA in a timely manner to meet the demands of specific cell functions.
Acknowledgements
Thank you to Dr Elizabeth Miles for her helpful comments and suggestions during the preparation of this manuscript.
G. C. B. has received research funding from Nestlé, Abbott Nutrition and Danone. He is a member of the Scientific Advisory Board of BASF and a member of the BASF Asia-Pacific Grant Award Panel. At the time the manuscript was submitted, he was the editor-in-chief of the British Journal of Nutrition but was excluded completely from the peer-review process.
G. C. B. declares no conflicts of interest with the content of this article.