Introduction
The gastrointestinal tract of humans hosts a complex ecosystem comprising diverse microorganisms, collectively called the gut microbiota (Fan et al. Reference Fan, Xia and Wang2023; Zong et al. Reference Zong, Fu and Xu2020). In the context of the gut microbiota and the host, it signifies a harmonious partnership where both the microbial community and the host organism derive advantages from each other. The gut microbiota, consisting of various microorganisms, and the host form a symbiotic relationship that goes beyond mere coexistence; it involves interdependence and cooperation, with each partner playing essential roles in maintaining overall health and functioning. Recent advancements in genomic research have improved the comprehension of these microbial communities and uncovered their remarkable impacts on human health, including complex interactions between the gut microbiota, immune system, and dietary carbohydrates. The symbiotic relationship between carbohydrate metabolism, immunity, and gut microbiota represents an important area of research, providing insights into the delicate balance of host–microbe interactions that influence overall well-being (Perlman et al. Reference Perlman, Martínez-Alvaro and Moraïs2022).
The gut microbiota, comprising fungi, viruses, bacteria, and archaea, constitutes a dynamic community that adapts to the host’s diet and lifestyle (Martino et al. Reference Martino, Dilmore and Burcham2022; Parizadeh and Arrieta Reference Parizadeh and Arrieta2023). Dietary carbohydrates play a crucial role in shaping this complex symbiosis (Dapa et al. Reference Dapa, Ramiro and Pedro2022). Carbohydrates, ranging from simple sugars to complex fibers, function as a major fuel source for the host and, when left undigested by host enzymes, a substrate for microbial fermentation. This relationship has significant implications for the immune system, as the gut provides an interface between immune cells and dietary and microbial antigens. Thus, the gut serves as a vital site for regulating immune responses by balancing host defense and tolerance (Henneke et al. Reference Henneke, Schlicht and Andreani2022; Palmer Reference Palmer2022; Pereira et al. Reference Pereira, de Lurdes Nunes Enes Dapkevicius and Borba2022).
This review delves into the intricate interplay among gut microbiota, the immune system, and carbohydrate metabolism, placing particular emphasis on nondigestible carbohydrates. It investigates the dynamic processes by which gut microbiota modulate host immune responses against carbohydrate-rich diets and, conversely, how immune factors exert influence on both the composition and functionality of gut microbiota. It further discusses the impact of specific carbohydrate types on the delicate balance within this tripartite relationship, including the complex signaling pathways, immune cell modulation, and microbial metabolic activities fundamental to this interdependence. Elucidating how carbohydrates mediate the tripartite interaction between gut microbiota and immunity not only deepens the understanding of fundamental host–microbe interactions but also paves the way for innovative therapeutic strategies. The insights derived from this exploration offer promising avenues for interventions designed to restore and maintain equilibrium in the gut ecosystem. These insights provide novel perspectives on preventive and therapeutic strategies for immune-related disorders and metabolic diseases. In the context of precision medicine, comprehending the complex relationships among gut microbiota, immunity, and carbohydrates is essential for realizing the full potential of personalized healthcare, thereby contributing to the advancement of overall health and well-being.
Carbohydrate digestion, absorption, and metabolism
Carbohydrate digestion, absorption, and metabolism collectively represent a fundamental and intricate process in the human body, essential for extracting energy and supporting various physiological functions (Sanders et al. Reference Sanders, Zhu and Wilcox2023). The journey of carbohydrates begins in the mouth, where salivary amylase initiates the breakdown of complex carbohydrates into simpler sugars. However, the primary site of carbohydrate digestion is the small intestine. In the small intestine, pancreatic amylase continues the hydrolysis of starches into oligosaccharides and maltose (Shinde and Vamkudoth Reference Shinde and Vamkudoth2022). The final steps occur at the brush border of absorptive cells, where enzymes like maltase, sucrase, and lactase break down oligosaccharides into monosaccharides such as glucose, fructose, and galactose. These monosaccharides are actively transported across the intestinal epithelium into the bloodstream, facilitating their absorption.
Once absorbed, monosaccharides play a pivotal role in metabolism. Glucose, a central monosaccharide, serves as a primary energy source for cellular activities (Remesar and Alemany Reference Remesar and Alemany2020). It can be immediately used for energy or stored as glycogen in the liver and muscles for later use. The metabolism of fructose and galactose involves conversion into glucose or intermediates that enter energy-producing pathways (Tian et al. Reference Tian, Zhao and Zhang2023). Insulin, a hormone secreted by the pancreas, plays a crucial role in regulating carbohydrate metabolism by facilitating glucose uptake into cells and promoting glycogen synthesis (Rahman et al. Reference Rahman, Hossain and Das2021). Additionally, the liver plays a key role in maintaining blood glucose levels by releasing glucose when needed and storing it as glycogen during periods of excess.
Carbohydrate metabolism is intricately linked to overall energy homeostasis, influencing not only immediate energy needs but also long-term storage and utilization. Dysregulation in carbohydrate metabolism can lead to various health issues, including metabolic disorders such as diabetes (Nagarajan et al. Reference Nagarajan, Cross and Sanna2022). Recent research on the role of gut microbiota in carbohydrate metabolism has made significant strides, uncovering intricate mechanisms and shedding light on the profound impact of microbial communities on host physiology. Studies have revealed that the gut microbiota not only participates in the fermentation of complex carbohydrates but also influences the production of metabolites like short-chain fatty acids (SCFAs) (Xie et al. Reference Xie, Alam and Marques2023), which play a crucial role in energy regulation and metabolic homeostasis. Furthermore, researchers have identified specific microbial species that exhibit distinct preferences for different types of carbohydrates, highlighting the microbial diversity involved in this intricate process (Takeuchi et al. Reference Takeuchi, Kubota and Nakanishi2023; Wardman et al. Reference Wardman, Bains and Rahfeld2022). These findings open up new avenues for understanding and potentially manipulating the gut microbiota to optimize carbohydrate metabolism, offering promising prospects for future therapeutic interventions in metabolic disorders and overall health.
In summary, carbohydrate digestion, absorption, and metabolism represent a tightly regulated and integrated process, ensuring the efficient extraction of energy and supporting the body’s diverse physiological functions.
Interply between nondigestible carbohydrates and gut microbiota
The interactions between carbohydrates and gut microbiota form a dynamic and symbiotic relationship, carrying substantial implications for the overall health of the host (Musso et al. Reference Musso, Gambino and Cassader2011). Within this complex relationship, carbohydrates function as nourishment for the extensive microbial communities in the gastrointestinal tract and crucial regulators of diverse physiological processes, such as energy metabolism, immune system modulation, and the synthesis of essential biomolecules, highlighting their pivotal role in maintaining overall health and homeostasis (Porter and Martens Reference Porter and Martens2017). This section explores the complex interaction between carbohydrates and gut microbiota, emphasizing the critical roles each plays in influencing the dynamics of the other.
Carbohydrates as microbial fuel: Nourishing the gut ecosystem
Carbohydrates, encompassing complex dietary fibers and simple sugars, are the primary energy source for the diverse microorganisms comprising the gut microbiota (Rastall et al. Reference Rastall, Diez-Municio and Forssten2022). While some carbohydrates are absorbed in the upper gastrointestinal tract, a significant portion of carbohydrates found in whole plant foods reach the colon, serving as substrates for microbial fermentation (Tiwari et al. Reference Tiwari, Mandal and Neupane2022).
The fermentation process serves as a metabolic engine, converting complex carbohydrates into metabolic byproducts, which encompass gases and beneficial SCFAs. This sustains the growth and activity of microbial communities (Rosli et al. Reference Rosli, Abd Gani and Khayat2023). This section examines the role of carbohydrates as essential fuel sources, detailing how they shape gut microbiota composition and functionality.
Carbohydrate forms: Dietary carbohydrates play a pivotal role in shaping the diversity and composition of the gut microbiota (Scott et al. Reference Scott, Gratz and Sheridan2013). Opportunistic pathobionts are known to multiply, and species that produce SCFAs are known to decrease when simple di- and monosaccharides are present (Wagenaar et al. Reference Wagenaar, van de Put and Bisschops2021). On the other hand, gut microbiota ferment undigested and microbiota-accessible carbohydrates (MACs), which increase levels of SCFAs and have beneficial health consequences (Tramontano et al. Reference Tramontano, Andrejev and Pruteanu2018). For example, Bacteroidota-related species thrive on diverse carbohydrates, whereas Firmicutes-related species specialize in fermenting specific carbohydrates and Proteobacteria-related species efficiently utilize simple sugars (Wexler Reference Wexler2007; Wexler and Goodman Reference Wexler and Goodman2017). Maintaining a balance between these microbial groups is essential for a harmonious and resilient gut ecosystem (Fig. 1).

Figure 1. Effects of different types of carbohydrates on the diversity and composition of gut microbiota. This diagram illustrates the sources, classification, and targeting of microorganisms with different types of carbohydrates.
(1). Complex carbohydrates:
Sources: Whole grains, fruits, vegetables, legumes, nuts, and seeds.
Effect: Complex non-starch polysaccharides and oligosaccharides that are considered dietary fibers can positively influence putatively health-promoting gut microbes, including Bacteroides, Akkermansia, Roseburia, Clostridium, Fecalibacterium, Prevotella, Ruminococcus, Lactobacillus, and Bifidobacterium (Zafar and Saier Reference Zafar and Saier2021). Certain MACs, like galacto-oligosaccharides (GOS) and fructo-oligosaccharides (FOS), are classified as prebiotics. Host microorganisms selectively use these prebiotic substrates to confer health benefits to the host (Zhang et al. Reference Zhang, Jin and Wang2022a). Complex carbohydrates are first broken down into oligosaccharides and simple sugars, and then into beneficial SCFAs like propionate, acetate, and butyrate. More specifically, butyrate promotes mucosal integrity, regulates inflammation, and keeps the gut lining healthy (Couto et al. Reference Couto, Gonçalves and Magro2020; Gonçalves et al. Reference Gonçalves, Araújo and Di Santo2018). Diets high in fiber help promote a healthy gut microbiome by encouraging the expansion of health-promoting bacteria such as Bifidobacterium and Lactobacillus (Cronin et al. Reference Cronin, Joyce and O’Toole2021; Kasahara et al. Reference Kasahara, Kerby and Romano2016).
(2). Resistant starch:
Sources: Legumes, unripe bananas, potatoes, and certain whole grains.
Effect: Resistant starches, evading complete digestion and absorption in the small intestine, successfully reach the colon, earning their classification as dietary fibers (Jiang et al. Reference Jiang, Du and Jiang2020). They function as substrates for bacterial fermentation in the colon, ultimately yielding the production of SCFAs. This type of carbohydrate has been associated with promoting beneficial bacteria, such as Bifidobacterium and Agathobacter, and may contribute to maintaining a healthy gut microbiota (Peredo-Lovillo et al. Reference Peredo-Lovillo, Romero-Luna and Jiménez-Fernández2020). Resistant starches also play a role in enhancing satiety and regulating blood glucose levels (Guo et al. Reference Guo, Brown and Tan2023).
(3). Simple sugars:
Sources: Caloric sweeteners, sugary snacks, and sweetened beverages.
Effect: Simple sugars are comprised of disaccharides, including lactose (glucose and galactose, found in milk), sucrose (fructose and glucose, commonly known as table sugar), and maltose (two glucose molecules), and monosaccharides, like glucose, galactose, and fructose. Diets rich in simple sugars may promote the growth of bacteria related to Proteobacteria and Firmicutes, which efficiently ferment these sugars (Jamar et al. Reference Jamar, Ribeiro and Pisani2021). This can lead to an imbalanced microbial composition, potentially promoting the overgrowth of less beneficial bacteria. For instance, certain microbial taxa have been found to predominate in specific dietary forms, like Clostridium sensu stricto within a diet rich in saturated fatty acids (short-, medium-, and long-chain SFAs like palmitic and myristic acids), Alloprevotella in a control diet, unclassified Porphyromonadaceae in a sugar-centric diet, and unclassified Ruminococcaceae in a diet rich in PUFA (n-6 polyunsaturated fatty acids like linoleic acid). Lactobacillus have been observed to influence dietary variations, particularly between PUFA- and sugar-rich diets compared to SFA-rich diets (Jamar et al. Reference Jamar, Ribeiro and Pisani2021). Moreover, consuming excessive amounts of simple sugars may lead to a reduction in microbial diversity and an undesirable shift in the gut microbiota, which is associated with dysregulated immunometabolism and the onset of chronic diseases (Arnone et al. Reference Arnone, Chabot and Heba2022).
(4). Prebiotics:
Sources: Garlic, onions, leeks, asparagus, and chicory root.
Effect: Indigestible fibers play a crucial role in fostering the development and activity of beneficial bacteria, particularly Bifidobacterium and Lactobacillus (Rezende et al. Reference Rezende, Lima and Naves2021). Since 2017, the International Scientific Association for Probiotics and Prebiotics (ISAPP) has broadened the concept of prebiotics by refining its definition to encompass “a substrate that is selectively utilized by host microorganisms, conferring a health benefit” (Swanson et al. Reference Swanson, Gibson and Hutkins2020). Therefore, in addition to FOS (fructans) and GOS, various bioactive compounds or biopolymers can also be classified as prebiotics. Prebiotics offer potential health benefits by modulating gut microbiota and promoting the release of beneficial microbial metabolites, such as SCFAs (Agus et al. Reference Agus, Planchais and Sokol2018), direct contribution to the growth and fermentation of probiotics (Blaser Reference Blaser2016), and serving as encapsulating materials for probiotics.
Regular consumption of prebiotics is associated with improved gut health, strengthened immune function, and potential protection against certain gastrointestinal disorders (Peng et al. Reference Peng, Tabashsum and Anderson2020). As an example, fructans, which consist of chains of fructose molecules, are recognized as prebiotics and have consistently shown to elevate the abundance of fecal Bifidobacterium in various clinical trials (Birkeland et al. Reference Birkeland, Gharagozlian and Birkeland2020). Inulin-type fructans stand out as one of the most frequently employed types of fructans.
Indeed, nutritional intervention with inulin has shown a heightened probability of positive outcomes in the context of metabolic disorders. Elevated abundance of genera including Arnesiella, Bilophila, Butyricimonas, Victivallis, Clostridium XIVa, Akkermansia, Raoultella, and Blautia has been observed, correlating with metabolic conditions like obesity and hepatic steatosis (Rodriguez et al. Reference Rodriguez, Hiel and Neyrinck2020). GOS, oligosaccharides similar to human milk oligosaccharides (Sangwan et al. Reference Sangwan, Tomar and Singh2011), display notable stability and solubility. They also exhibit good moisture retention and are resistant to facile hydrolysis by human digestive enzymes (Macfarlane et al. Reference Macfarlane, Steed and Macfarlane2008). Upon fermentation by colonic bacteria, GOS yield SCFAs, carbon dioxide, and hydrogen. This specific metabolic process serves to stimulate the growth of intestinal Bifidobacterium (Canfora et al. Reference Canfora, van der Beek and Hermes2017). However, sustained supplementation of GOS could simultaneously enhance the growth of these two bacterial types, thereby reshaping the ecological relationship, diminishing antagonistic effects, and reducing the fluctuation of the intestinal microecology (Ma et al. Reference Ma, Wasti and Huang2020).
In summary, the consumption of dietary carbohydrates has a remarkable impact on how the gut microbiota is structured and how it functions. Prebiotics, fibers, including resistant starches, oligosaccharides, and complex non-starch polysaccharides, as well as other bioactive substances, promote microbial diversity and the enrichment of beneficial microorganisms. It aids in the synthesis of metabolites that are beneficial to the digestive tract. Conversely, diets high in simple sugars may lead to imbalances in the gut microbiota, potentially impacting overall well-being and increasing the risk of certain chonic diseases.
Carbohydrates over proteins: Proteases break down the dietary proteins consisting of conserved peptide bonds. Gut bacteria synthesize metallo-, serine-, cysteine-, and aspartic-proteases; however, human cell-derived proteases outweigh these bacterial enzymes in most fecal samples (Vergnolle Reference Vergnolle2016). Unlike monosaccharides, the 20 proteinogenic amino acids have more interconversion stages to be integrated into metabolic pathways, so it is unusual for a single bacterial species within the gut microbial community to ferment all amino acids for energy (Lin et al. Reference Lin, Liu and Piao2017). Bypassing the need for amino acid production, the anabolic use of ambient amino acids by microbes saves greater amounts of energy compared to their catabolic usage (Portune et al. Reference Portune, Beaumont and Davila2016). Therefore, amino acids are generally less efficient as an energy source than carbohydrates for gut microbiota. This explains why the gut microbiota preferentially consumes carbohydrates over proteins, depending on availability (Geboes et al. Reference Geboes, De Hertogh and De Preter2006).
Cross-feeding dynamics: The gut microbiota, consisting of diverse microbial species, participates in a complex network of cross-feeding and communication facilitated by the availability of carbohydrates. Some bacterial species can utilize by-products of carbohydrate fermentation produced by other species (Oliphant and Allen-Vercoe Reference Oliphant and Allen-Vercoe2019; Zhang et al. Reference Zhang, Wang and Jiang2021). This dynamic interaction fosters a cooperative environment, influencing the diversity, resilience, and stability of the gut microbiota. For instance, Bacteroides thetaiotaomicron has 260 glycoside hydrolases (Xu et al. Reference Xu, Bjursell and Himrod2003), highlighting the evolutionary adaptation required to efficiently utilize various dietary fiber types. However, how the microbial community communicates through signaling molecules, responds to changes in carbohydrate availability, and orchestrates collective responses that influence host physiology still needs further investigation.
Microbial fermentation: A metabolic symphony unleashed
Microbial fermentation transforms carbohydrates into various metabolites within the anaerobic environment of the colon (Petit et al. Reference Petit, de Bruijn and Goldman2022). This process involves the production of SCFAs such as acetate, propionate, and butyrate. These SCFAs not only act as an energy source for the host but also exert immunomodulatory effects (Jasim et al. Reference Jasim, Opulencia and Ramirez-Coronel2022; Ranjbar et al. Reference Ranjbar, Vahdati and Tavakoli2021). This section examines the complex metabolic pathways involved in carbohydrate fermentation, emphasizing the diversity of microbial communities contributing to this process. The resulting metabolites are pivotal in maintaining gut homeostasis, influencing immune responses, and impacting overall host health.
(1). Metabolic pathways in carbohydrate fermentation
The fermentation of carbohydrates is a multistep biological process that often results in the production of energy for microbes via the reduction of carbohydrates to simpler molecules (Panahi et al. Reference Panahi, Dehhaghi and Guillemin2022). This complex metabolic pathway highlights the remarkable diversity of microbial communities that contribute to this essential biological function.
Various microbial groups, including bacteria, archaea, and fungi, are at the heart of carbohydrate fermentation, each possessing unique enzymatic capabilities (Zeise et al. Reference Zeise, Woods and Huffnagle2021). These microorganisms play pivotal roles in different stages of carbohydrate metabolism, allowing for the use of a wide range of substrates (Portincasa et al. Reference Portincasa, Bonfrate and Vacca2022). The diversity within these microbial communities ensures the adaptation to various environmental conditions, nutritional sources, and ecological niches (Shu and Huang Reference Shu and Huang2022).
Carbohydrates, varying from simple sugars to complex polysaccharides, undergo initial enzymatic hydrolysis by a range of glycosidases, amylases, and cellulases (Bilal and Iqbal Reference Bilal and Iqbal2020). This initial hydrolysis step paves the way for subsequent metabolic transformations. The breakdown of carbohydrates leads to the formation of intermediary metabolites like pyruvate and acetyl-CoA, which act as central nodes in the complex network of fermentation pathways (Francois et al. Reference Francois, Lachaux and Morin2019). The diversity in microbial enzymatic repertoires dictates the fate of these metabolites, resulting in various end products, including organic acids, alcohols, and gases (Van Treuren and Dodd Reference Van Treuren and Dodd2020). The metabolic pathways involved are notably diverse, including homolactic, heterolactic, alcoholic, and mixed-acid fermentations, among others (Van Treuren and Dodd Reference Van Treuren and Dodd2020).
(2). Microbial diversity and carbohydrate fermentation
Notably, the specific pathways employed during carbohydrate fermentation vary among microbial groups. For instance, lactic acid bacteria, including species of Lactobacillus and Streptococcus, specialize in producing lactic acid through the homolactic fermentation pathway (Tian et al. Reference Tian, Chen and Liu2021). Meanwhile, yeast species like Saccharomyces cerevisiae predominantly utilize the alcoholic fermentation pathway, producing ethanol (da Silva Fernandes et al. Reference da Silva Fernandes, de Souza and Carneiro2022; Maicas Reference Maicas2020).
The microbial communities involved in carbohydrate fermentation are diverse in their metabolic capabilities and exhibit a high degree of interdependence. Syntrophic relationships are common in anaerobic environments where different microbial species cooperate to accomplish a metabolic task. For example, in the anaerobic degradation of complex carbohydrates like cellulose, bacteria, and fungi work synergistically to hydrolyze, ferment, and metabolize the released sugars (Blair et al. Reference Blair, Dickson and O’Malley2021). The ecological significance of carbohydrate fermentation extends beyond microbial metabolism (Cataldo et al. Reference Cataldo, Villegas and de Giori2020). These processes profoundly impact nutrient cycling, carbon fluxes, and the overall balance of ecosystems. Understanding the complex metabolic pathways and the diversity of microbial communities involved in carbohydrate fermentation is crucial for unraveling the complexities of microbial ecology and its implications for various natural and engineered environments (Zimmerman et al. Reference Zimmerman, Howard-Varona and Needham2020).
Gut microbiota-mediated metabolism of carbohydrates in immune regulation
The microbial metabolism of dietary carbohydrates is emerging as a crucial factor in immune control, highlighting that the symbiotic interaction between the host and the gut microbiota extends beyond the realm of digestion (Daisley et al. Reference Daisley, Koenig and Engelbrecht2021; Spencer et al. Reference Spencer, Fragiadakis and Sonnenburg2019). The microbial fermentation of dietary fibers and complex carbohydrates yields SCFAs, including butyrate, propionate, and acetate. SCFAs serve as versatile signaling molecules influencing immune cells through various mechanisms (Kim Reference Kim2023). Butyrate, for example, acts as a histone deacetylase (HDAC) inhibitor, promoting an anti-inflammatory environment and enhancing regulatory T cell (Tregs) differentiation, which is pivotal in immune tolerance (Salvi and Cowles Reference Salvi and Cowles2021). This section elucidates the complex molecular interaction between gut microbiota-mediated carbohydrate metabolites and the host immune system, unveiling how these metabolites shape immune responses and maintain immune homeostasis (Fig. 2).
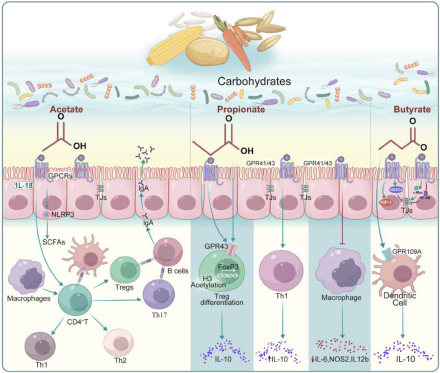
Figure 2. Regulation of the host immune function by short-chain fatty acids (SCFAs) produced by gut microbes that metabolize carbohydrates. Focusing on the three most common SCFAs (acetate, propionate, and butyrate), the figure elucidates their receptors, target cells and related signaling pathways.
Acetate
Acetate, a prevalent SCFA, functions as a nutrient for host cells and has been thoroughly investigated for its involvement in regulating mucosal immunity. Microorganisms commonly secrete acetate and other fermentation acids during growth, and it is well-established that acid accumulation in the growth medium inhibits microbial growth (Luu and Visekruna Reference Luu and Visekruna2019). Beyond serving as an energy source, acetate has been demonstrated to positively affect immune modulation.
To detect ambient acetate, intestinal enterocytes and other cell types contain G-protein-coupled receptors, which mediate most of the molecular effects of acetate (Hosmer et al. Reference Hosmer, McEwan and Kappler2023). One example is the fact that oral acetate may upregulate IFN-stimulated genes in the lung, leading to interferon-β (IFN-β) responses (Antunes et al. Reference Antunes, Fachi and de Paula2019). In mice infected with respiratory syncytial virus (RSV), these effects were correlated with a reduction in viral load and lung inflammation (Antunes et al. Reference Antunes, Fachi and de Paula2019).
The antiviral activity of acetate in pulmonary epithelial cell lines and its positive effects in RSV-infected animals were substantiated by type 1 IFN signaling through the IFN-alpha receptor (IFNAR) (Antunes et al. Reference Antunes, Fachi and de Paula2019). Increased blood acetate concentrations stimulate neutrophils in a GPR43-dependent way, promoting phagocytic receptor upregulation, oxidative burst, neutrophil chemotaxis, and cytokine release (Schlatterer et al. Reference Schlatterer, Beck and Schoppmeier2021). Indeed, acetate consistently induces the activation of the NLRP3 inflammasome in intestinal epithelial cells, a mechanism that has been shown to play a key role in protecting against colitis in nlrp3 −/− mice (Xu et al. Reference Xu, Jiang and Wang2019). Daïen et al. demonstrated that acetate directly promotes IL-10-producing regulatory B cells (B10 cells) differentiation in mice and humans (both in vivo and in vitro) (Daien et al. Reference Daien, Tan and Audo2021). They have observed that the induction of functional B10 cells by acetate effectively facilitates the differentiation of naive T cells to Tregs.
A short-term dietary intervention involving increased fiber intake in women led to elevated plasma acetate levels and a higher proportion of B10 cells, underscoring acetate’s efficacy as an inducer of B10 cells (Daien et al. Reference Daien, Tan and Audo2021). Additionally, acetate priming has been demonstrated to enhance the capability of human neutrophils to eliminate methicillin-resistant strains Staphylococcus aureus (Schlatterer et al. Reference Schlatterer, Beck and Schoppmeier2021). The immediate assimilation of acetate also augments the capacity of memory CD8+ T cells to produce IFN-gamma (IFN-γ) (Balmer et al. Reference Balmer, Ma and Thompson2020). Nevertheless, the potential impact of acetate on modulating the metabolism and function of memory CD8+ T cells during their encounter with pathogens upon re-exposure remains unexplored. Furthermore, acetate plays a role in maintaining intestinal barrier function, aiding wound healing, and effecting changes in the actin cytoskeleton, all crucial aspects of mucosal immunity (Hung et al. Reference Hung, Wu and Pao2022; Lyu et al. Reference Lyu, Pirooznia and Li2022; Nakano et al. Reference Nakano, Uchiyama and Ushiroda2020; Xiong et al. Reference Xiong, Kawagishi and Yan2018).
Propionate
Propionate, also referred to as propionic acid, is a SCFA with three carbon atoms and holds promise in immune regulation. It is produced by commensal gut microbiota within the proximal colon and cecum of mammals. This production occurs through the anaerobic fermentation of oligosaccharides and sugars that originate from enzymatically degraded fibers (Langfeld et al. Reference Langfeld, Du and Bereswill2021). In the intestinal tract of humans, propionate is predominantly produced by members of Firmicutes and Bacteroidetes phyla. These microbial groups generate propionate via the propanediol and succinate pathways, both dependent on a cofactor, vitamin B12 (Louis and Flint Reference Louis and Flint2017; Morrison and Preston Reference Morrison and Preston2016).
Various research studies have investigated the effects of propionate on immune modulation. It inhibits HDACs, influencing gene expression in immune cells. For instance, a study exploring the function of SCFAs on human basophils found that propionate induces histone acetylation in these cells, and suppression of HDAC activity mimicked propionate’s effects (Shi et al. Reference Shi, Xu and Pan2021). These findings indicated that propionate may play a complex role in regulating basophil apoptosis, activation, and degranulation via inhibiting HDAC activity (Shi et al. Reference Shi, Xu and Pan2021). Moreover, engineered propionate-producing bacteria have been shown to attenuate dextran sodium sulfate (DSS)-induced colitis in mice by modulating the immune function of resident macrophages and the production of classic inflammation-related cytokines, suggesting a potential treatment strategy for ulcerative colitis (Feng et al. Reference Feng, Wang and Kang2023). Consistently, in the gut, propionate supports intestinal epithelial integrity and barrier function (Shi et al. Reference Shi, Li and Duan2017). As a signaling molecule, increased levels of gut microbiome-derived propionate are linked to reduced sterile lung inflammation and enhanced bacterial immunity in mice (Tian et al. Reference Tian, Hellman and Horswill2019). Additionally, Du et al. discovered that gut microflora influences Th17/Treg cell differentiation in experimental autoimmune prostatitis through propionate (Du et al. Reference Du, Yue and Niu2022). Propionate also acts as a substrate for gluconeogenesis in the liver and colonic epithelium, thereby linking microbial metabolism with systemic metabolic processes that affect immune cell function (Luu and Visekruna Reference Luu and Visekruna2019). Future research is needed to elucidate the underlying molecular mechanisms further and lay the groundwork for clinical studies, potentially broadening the use of propionate as a promising option in combating infections and immune diseases related to metabolic disorders.
Butyrate
Butyrate, one of the most extensively studied SCFA, significantly affects immune regulation. Beyond its role as an epigenetic modifier, butyrate boosts the generation and function of Tregs, thereby suppressing inflammatory responses (Couto et al. Reference Couto, Gonçalves and Magro2020). Through its HDAC inhibiting function, butyrate increases Foxp3 protein acetylation, leading to elevated Foxp3 protein levels in Treg cell cultures (Arpaia et al. Reference Arpaia, Campbell and Fan2013). Kespohl et al. investigated the impact of various butyrate concentrations (0.1–1 mM in vitro and 50–200 mM in vivo via oral administration) on T cell-mediated immune responses using CD4+ T cells (Kespohl et al. Reference Kespohl, Vachharajani and Luu2017). They reported that butyrate facilitated the Tregs differentiation in vivo and in vitro at lower concentrations (0.1–0.5 mM). Conversely, butyrate induced the transcription factor T-bet expression at a higher concentration (1 mM), leading to the development of conventional T cells or IFN-γ-producing Tregs (Kespohl et al. Reference Kespohl, Vachharajani and Luu2017); however, conflicting data exist. Other studies using varying doses of butyrate observed a direct inhibitory effect on B10 cells. These studies speculate that the previously reported induction of B10 cells by butyrate might result from indirect effects mediated by the serotonin-derived metabolite 5-hydroxyindole-3-acetic acid (Daien et al. Reference Daien, Tan and Audo2021).
Butyrate plays a crucial role in reinforcing the integrity of the gut barrier, thereby preventing the translocation of microbial products that could lead to aberrant immune activation. Experimental in vivo and in vitro models have demonstrated that butyrate supports intestinal integrity. Intestinal cell models (Cresci et al. Reference Cresci, Glueck and McMullen2017; Nielsen et al. Reference Nielsen, Jensen and Theil2018) under various stress conditions, such as lipopolysaccharide (LPS) (Feng et al. Reference Feng, Wang and Wang2018) and ethanol (Cresci et al. Reference Cresci, Bush and Nagy2014), show that butyrate treatment protects against intestinal epithelial integrity disruption, as evidenced by measurements of transepithelial electrical resistance (TER) and fluorescein–isothiocyanate–dextran permeability. Several mechanisms illustrate butyrate’s direct effects on the intestinal epithelial barrier. Butyrate-induced AMP-activated protein kinase activity leads to increased tight junction reassembly and TER restoration (Eamin et al. Reference Eamin, Masclee and Dekker2013). In the presence of LPS, the inhibition of HDAC activity with butyrate not only protects against LPS-induced TER reduction and paracellular permeability but also results in reduced activation of the NLRP3 inflammasome and autophagy (Siddiqui and Cresci Reference Siddiqui and Cresci2021). Furthermore, butyrate stabilizes hypoxia-inducible factor, a transcription factor vital for managing low oxygen levels in the colonic epithelium and regulating intestinal barrier function (Kelly et al. Reference Kelly, Zheng and Campbell2015). Collectively, these findings indicate that butyrate directly benefits intestinal epithelial barrier integrity in a dose-dependent manner.
Carbohydrate-derived microbial products in immune signaling
The complex interaction between carbohydrates and the gut microbiota extends its influence beyond metabolic processes, reaching into host immunity. Carbohydrate-derived microbial products serve as ligands for various pattern recognition receptors expressed on immune cells. TLRs, nucleotide-binding oligomerization domain-containing proteins (NODs), and C-type lectin receptors recognize specific carbohydrate structures, initiating intracellular signaling cascades. This recognition is a pivotal step in immune activation, leading to the production of chemokines, cytokines, and other effector molecules. This section explores the diverse ways in which these microbial products engage in immune signaling, shaping the delicate balance between tolerance and defense (Fig. 3).

Figure 3. Carbohydrate-derived microbial products regulate host immune function. Carbohydrate-derived microbial products serve as ligands for various pattern recognition receptors, including TLRs, NODs, intracellular signaling cascades, and immune cell homeostasis.
Toll-like receptor activation
Toll-like receptors (TLRs), integral to innate immunity, play a central role in recognizing conserved microbial structures. Carbohydrate moieties, such as LPS and glycolipids, engage TLRs, activating downstream signaling pathways. This activation stimulates the expression of proinflammatory cytokines, shaping the immune response to microbial challenges and contributing to the defense against invading pathogens. For instance, one study demonstrated that pectic heteropolysaccharides selectively cluster with TLR4 during endocytosis, thereby inducing downstream signaling that leads to the phenotypic activation of macrophages (Hyun et al. Reference Hyun, Cho and Yang2023). Similarly, research utilizing a high-sugar diet model indicated that a diet rich in simple sugars promotes a proinflammatory response through alterations in gut microbiota and TLR4 signaling (Fajstova et al. Reference Fajstova, Galanova and Coufal2020). Another study investigated the modulatory effects of graminan-type fructans (GTFs) on TLRs, comparing these effects to those of inulin-type fructan I (ITFs) with different chain lengths (Fernández-Lainez et al. Reference Fernández-Lainez, Akkerman and Oerlemans2022). They found that GTFs activate nuclear factor-kappa B (NF-κB)/AP-1 via MyD88 and TRIF pathways, stimulating TLR3, 7, and 9, whereas ITFs activate TLR2 and TLR4. GTFs strongly inhibited TLR2 and TLR4, while ITFs did not inhibit any TLR (Fernández-Lainez et al. Reference Fernández-Lainez, Akkerman and Oerlemans2022). These findings highlight the immunomodulatory effects of GTFs via TLRs and the attenuation of cytokine production in dendritic cells by both GTFs and long-chain ITFs.
NOD activation
NOD1 and NOD2 are intracellular receptors that recognize peptidoglycan fragments, including carbohydrate components (Bastos et al. Reference Bastos, Wheeler and Boneca2021). NOD proteins are part of the innate immune system and are crucial in recognizing microbial components (Trindade and Chen Reference Trindade and Chen2020). These proteins are involved in signaling pathways that trigger immune responses against invading pathogens. Imbalances or dysregulation in the normal functioning of NOD-mediated signaling pathways can lead to abnormal immune responses. NOD1 is a receptor that recognizes meso-diaminopimelic acid-containing peptidoglycan, predominantly found in Gram-negative bacteria (Clarke et al. Reference Clarke, Davis and Lysenko2010). NOD2, on the other hand, serves as a receptor for peptidoglycan from both Gram-negative and Gram-positive bacteria (Danis and Mellett Reference Danis and Mellett2021; Kanneganti et al. Reference Kanneganti, Lamkanfi and Núñez2007). Activation of NOD2 induces the production of IL-17A in innate lymphoid cells (Ermann et al. Reference Ermann, Staton and Glickman2014). Furthermore, Paneth cells secrete α-defensins in a NOD2-dependent manner (Kobayashi et al. Reference Kobayashi, Chamaillard and Ogura2005). The peptidoglycan recognition proteins, including Pglyrp1, Pglyrp2, Pglyrp3, and Pglyrp4, detect peptidoglycan and exert antibacterial activity (Liu et al. Reference Liu, Gelius and Liu2000). NLRC4 functions as a receptor for intracellular flagellin, and its activation triggers caspase-1, inducing IL-1β expression (Franchi et al. Reference Franchi, Amer and Body-Malapel2006). Mice lacking NLRC4 exhibit severe disease and epithelial injury following DSS treatment (Carvalho et al. Reference Carvalho, Nalbantoglu and Aitken2012). Mortality increases in NLRC4-deficient mice infected with flagellated Salmonella, suggesting that NLRC4 enhances immune defense in the intestine. Collectively, these findings underscore the crucial role of these microbial products in influencing the immune system and maintaining immune homeostasis.
In summary, this discussion highlights the complex interplay between NOD-mediated signaling, its dysregulation, and the resultant impact on autoimmune and inflammatory disorders, alongside the role of carbohydrate-derived microbial products in maintaining immune homeostasis. Comprehending these complex relationships is vital for understanding the mechanisms driving immune-related conditions and identifying potential therapeutic approaches.
Intracellular signaling cascades
Carbohydrate-mediated immune signaling encompasses complex intracellular cascades, notably including the mitogen-activated protein kinase (MAPK) pathways and activation of NF-κB. These pathways converge to regulate gene expression, influencing the production of inflammatory mediators, antimicrobial peptides, and molecules involved in tissue repair. Microbial products like LPS, flagellin, lipoteichoic acid, and lipopeptide predominantly activate NF-κB, which is known to bind to the promoter region of mucin 2 (Paone and Cani Reference Paone and Cani2020). Similarly, several inflammatory markers such as TNF-α, interleukins, and serum amyloid A3 activate the NF-κB pathway, stimulating mucin 2 transcription (Tashiro et al. Reference Tashiro, Iwata and Yamauchi2017), while the Janus Kinase pathway activation exerts inhibitory effects (Ahn et al. Reference Ahn, Crawley and Hokari2005). A study investigating the impact of three different sucrose doses on DSS-induced colitis in mice revealed that varying sucrose consumption levels differentially affect the gut microbiota and the PPAR-γ/MAPK/NF-κB pathway (Zhang et al. Reference Zhang, Zhang and Peng2022b).
Modulation of immune homeostasis
Microbial products derived from carbohydrates directly influence immune cell functions, including phagocytosis, antigen presentation, and the differentiation of various immune cell subsets. By influencing these cellular processes, microbial products actively shape the magnitude and character of the immune response. For instance, under conditions lacking MACs, CD103+ dendritic cells demonstrated a reduced capacity to generate tolerogenic Tregs compared to conditions with high-MAC intake (Tan et al. Reference Tan, McKenzie and Vuillermin2016). In a food allergy model, mice on a MAC-free diet showed more severe clinical anaphylaxis than those on a high-MAC diet (Tan et al. Reference Tan, McKenzie and Vuillermin2016). Moreover, MACs are critical antibody response regulators in the gut and systemically. Mice on a MAC-free diet displayed impaired homeostatic and pathogen-specific antibody responses (Kim et al. Reference Kim, Qie and Park2016). Conversely, mice on a high-MAC diet showed significantly increased immunoglobulin A (IgA) production (Kim et al. Reference Kim, Qie and Park2016). High-MAC feeding may lead to an improved T follicular helper response, characterized by enhanced germinal center activities in Peyer’s patches and a higher IgA+ B cell presence in the small intestine (Tan et al. Reference Tan, McKenzie and Vuillermin2016). These effects are attributed to MAC-induced alterations in gut microbiota composition and SCFA production, which support the production of B cell antibodies through the promotion of plasma cell differentiation (Kim et al. Reference Kim, Qie and Park2016). Therefore, dietary interventions combined with beneficial bacterial strain administration could serve as a cost-effective approach to managing various noncommunicable diseases associated with Western lifestyles. Further research is required to ascertain (1) which MACs are most effective in microbiota diversification and enhancing SCFA production and (2) the optimal intake of MACs for health maintenance or for treating various inflammatory diseases.
Conclusion
In summary, this review comprehensively explores the intricate relationships between carbohydrates, gut microbiota, and host immunity, emphasizing the pivotal role of the gut microbiota as a bridge in this dynamic relationship. The interaction between carbohydrate-derived microbial products and immune signaling forms a sophisticated network central to host–microbiota interactions. Understanding the nuances of this signaling cascade provides insights into how the immune system interprets and responds to the presence of specific carbohydrates, offering potential targets for therapeutic interventions in immune-related disorders. As research in this field advances, unraveling the complexities of carbohydrate-mediated immune signaling promises to open new avenues for manipulating immune responses for health and disease, including the refinement of precision nutrition approaches and the development of targeted therapeutics based on carbohydrates.
Implications and future directions
The review concludes with a forward-looking perspective, highlighting areas for future research to elucidate the complexity of gut microbiota interactions in the context of carbohydrate metabolism and host immunity. The modulation of gut microbiota-mediated carbohydrate metabolism emerges as a potential avenue for therapeutic interventions in immune-related disorders. Precision nutrition, prebiotics, and probiotics aimed at restoring a balanced microbial community and promoting the production of beneficial metabolites hold promise for influencing immune regulation. Future research should continue to unravel the specific mechanisms by which gut microbiota-derived metabolites impact immune responses, paving the way for personalized approaches to immune modulation.
Acknowledgements
This work was supported by the National Key Research and Development Project of China (Grant/Award Nos. 2022YFD1301500, 2023YFD1301101, 2022YFD1300505); National Natural Science Foundation of China (Grant/Award Nos. 32372890, 32372889); Fundamental Research Funds for the Central Universities (Grant/Award No. K20230021).
Author contributions
X.Z. and M.J conceived the project, designed the article structure. X.Z wrote the manuscripts. All authors discussed and edited the manuscript.
Conflict(s) of interest
The authors declare that the research was conducted in the absence of any commercial or financial relationships that could be construed as a potential conflict of interest.