INTRODUCTION
In recent years, paleopedology has shifted from a largely qualitative discipline to an increasingly quantitative endeavor (Sheldon and Tabor Reference Sheldon and Tabor2009). A good example is the study of paleosols in coastal sedimentary sequences. Coastal paleosols form during protracted sea level lowstands associated with glacial stadials, such as during the last glacial maximum (LGM). Paleosols often form relatively thick, solitary units that can be used as basin-wide stratigraphic markers of sea level lowering. Examples include Late Quaternary paleosols from the Changjiang Delta (Chen et al. Reference Chen, Sun and Li1996, Reference Chen, Li, Li, Liu and Sun2008; Li and Wang Reference Li and Wang1998); Yellow Sea, western coast of Korea (Lim and Park Reference Lim and Park2003; Choi Reference Choi2005); Mississippi River Delta (Fisk Reference Fisk1944; Aslin and Autin Reference Aslan and Autin1998; Autin and Aslan Reference Autin and Aslan2001), and Po River coastal plain on the Adriatic Sea (Amorosi et al. Reference Amorosi, Bruno, Cleveland, Morelli and Hong2016; Bruno et al. Reference Bruno, Amorosi, Severi and Costagli2017; Morelli et al. Reference Morelli, Bruno, Cleveland, Drexler and Amorosi2017). Paleosols provide information about changes in sediment deposition, subsidence, erosion and pedogenesis (Kraus Reference Kraus1999). In a deltaic setting, the thickness and extent of weathering of a particular paleosol reflects the lateral variability associated with basin topography, and site-specific sedimentation. Coastal paleosols can serve as key stratigraphic markers in reconstructing the glacial-deglacial sequence stratigraphy of a deltaic system, and their relationship to sea level.
Here, we extend the work of Shang et al. (Reference Shang, Fan, Yin, Burr, Zhang and Wang2018), who studied the sedimentological history and paleoclimate of the Oujiang Delta since MIS3, based on the lithological, palynological, and microfossil characteristics of cored sediments. In this paper we focus on two paleosols from the Oujiang Delta. These coastal paleosols formed when sea level dropped, and place constraints on former sea level. We verify that these deposits are paleosols based on lithological characteristics, clay mineralogy, major element chemistry, and Rb/Sr content. Radiocarbon (14C) dates from charcoal and plant material contained in the paleosols show that one paleosol formed during a period of changing sea level in the Early Holocene, and the other paleosol formed during a time of sea level lowering, near the end of Marine Isotope Stage 3 (MIS 3). Our goal in this article is to is understand the relationship between the paleosols and eustatic sea level change.
The Oujiang Delta
The Oujiang Delta was selected for study because it is a relatively straightforward system, fed by sediments carried by a single river. It is the second largest river in Zhejiang Province and drains an area of about 18,000 km2 from low-lying coastal mountains to the south and west into the East China Sea (Figure 1). The mean annual water discharge of the Oujiang River is 1.67×1010 m3 and the mean annual sediment discharge is 2.22×106 tons. The river originates at Baishanzu Mountain (peak elevation of 1857 m) in Southwest Zhejiang Province, and winds 388 km through low mountainous terrain before debouching into Yueqing Bay, east of Wenzhou (Figure 1). The bay follows the coast towards the northeast, where a number of rocky islands effectively separate it from the open ocean.

Figure 1 Map of the Oujiang River in Zhejiang Province, China.
The Oujiang drainage basin experiences a subtropical monsoon climate, and is subject to typhoons in summer. The annual precipitation in the region varies from 1100 to 2200 mm. Most of the rainfall is concentrated in summer (April to September), and summertime river runoff accounts for 78% of the annual total (Xie et al. Reference Xie, Li, Xia, Li, Van Weering and Berger1994; Zhang et al. Reference Zhang, Fan, Wu, Shang and Chen2012). Heavy rainfall induced by typhoons can produce severe flood events in the region, with hugely disproportionate flooding, as compared with typhoon-induced flooding on large rivers, such as the Changjiang River. This is because even a small typhoon 200 km across (~30,000 km2) can cover the entire Oujiang River watershed (18,000 km2) at one time, while the same storm can only affect less than two percent of the much larger, Changjiang River watershed (1,808,500 km2). These kinds of intense flooding events are analogous to those observed for small mountainous rivers in Taiwan (Liu et al. Reference Liu, Kao, Huh and Hung2013), albeit with much milder stream gradients for the Oujiang River.
The mouth of the Oujiang forms a macro-tidal setting with mean and maximum tidal ranges of 4–5 m and 7.15 m, respectively (Li et al. Reference Li, Xie, Xia and Li1994; Xie et al. Reference Xie, Li, Xia, Li, Van Weering and Berger1994). Waves are generally low with a multi-year mean wave height of less than 0.5 m, but a maximum wave height of over 2.4 m can occur when typhoons make landfall. On average, four typhoons impact the coast in this region every year (Xie et al. Reference Xie, Li, Xia, Li, Van Weering and Berger1994). The present day delta and chenier plain formed following the mid-Holocene maximum transgression with an extreme landward boundary near Qingtian (Zhang Reference Zhang1990; Zhu Reference Zhu1993). The south flank of the delta features four shore-parallel chenier ridges (Figure 2), including (in a seaward direction): (1) the Siqian, (2) Ningcun, (3) Wuxi, and (4) Jiangding ridges (Zhu Reference Zhu1993). The total thickness of the Holocene strata in the study area is about 30–50 m (Wang et al. Reference Wang, Min and Bian1982; Yang and Chen Reference Yang and Chen1982).

Figure 2 Drill sites (YQ0901-YQ0904) from the Oujiang Delta. Individual chenier ridges, shown in aquamarine, are Siqian ridge at the foothills, Ningcun and Wuxi ridges at the middle, and Jiangding ridge near the present coastline.
METHODS
Core Logging and Grain-Size Analysis
Four 11-cm-diameter cores were drilled from the Wenrui chenier plain on the south flank of the modern-day Oujiang River in June 2009 (Figure 2). These are referred to as YQ0901, YQ0902, YQ0903, and YQ0904. The elevations of the coring sites were surveyed by a trigonometric leveling method, referring to regional survey datum points. The core depth was determined directly from the length of drilling rods used for core retrieval. The location, elevation, core length and percent recovery of each core is given in Table 1. The cores were split lengthwise in the laboratory, and the working surfaces of the core halves were cleaned with stainless steel knives to reveal sedimentary structures. These were photographed, described, and analyzed with a nondestructive X-ray fluorescence (XRF) core scanner before subsampling. The core descriptions include sediment color, lithology, grain-size variation, sedimentary structures, macrofossil and wood content. Subsamples were taken every two centimeters, sealed in plastic bags, and refrigerated at 4°C, while awaiting further analyses. Large pieces of wood, charcoal, plants and shell, observable to the naked eye, were collected for accelerator mass spectrometry (AMS) 14C dating.
Table 1 Borehole details from the four cores collected from the Wenrui chenier plain.

Sediment samples were selected at ~20-cm intervals for grain-size analysis using a laser diffraction particle size analyzer (Coulter LS230) at the State Key Laboratory of Marine Geology, Tongji University. Samples were pretreated sequentially with 10% H2O2 and 1 N HCl to remove organic matter and carbonates (Fan et al. Reference Fan, Shang, Cai and Tu2015). Mean grain size and the percentage of clay (φ>8), silt (4–8φ), and sand (φ<4) components were determined.
XRF Analysis
XRF spectra were measured for all of the cores in this study to extract elemental concentration data along the length of the sediment cores (Croudace et al. Reference Croudace, Rindby and Rothwell2006; Richter et al. Reference Richter, van der Gaast, Koster, Vaars, Gieles, de Stigter, de Haas and van Weering2006). Our cores were analyzed using an Avaatech XRF Core Scanner at the State Key Laboratory of Marine Geology in Shanghai. Measurements were performed every 1 cm along core, with a counting time of 30 sec and with 10 kV, 30 kV, and 50 kV acceleration voltages. The acquired XRF spectra were processed with the KEVEX software Toolbox to determine elemental abundances, including: Na, Al, Si, Ca, Ti, Mn, Fe, Rb, and Sr.
In this study, we normalized the major element data to Ti, due to its terrigenous origin, conservative nature during transport, and the XRF’s analytical facility for Ti measurements (Murray and Leinen Reference Murray and Leinen1996; Hennekam and de Lange Reference Hennekam and de Lange2012). The relative element content, and single element counts normalized to total counts at 10 kV acceleration voltage, were used to reduce system error (Revel et al. Reference Revel, Ducassou, Grousset, Bernasconi, Migeon, Revillon, Mascle, Murate, Zaragosi and Bosch2010; Wu et al. Reference Wu, Zhang, Xu and Shen2012).
ICP-AES Analysis
A total of 69 subsamples were collected from core YQ0904 at ~50-cm intervals. The concentrations of major elements were determined with ICP-AES (IRIS Advantage) in the State Key Laboratory of Marine Geology, Tongji University (Shanghai). All of the bulk sediment samples were combusted in a muffle furnace at 600°C for 2 hr before acid digestion to determine loss on ignition. The samples were then dissolved in a mixture of HNO3 and HF on a hot plate. Replicate analyses (N=20) of GSR-5, GSR-6, and GSD-9 standards during the study gave an accuracy of 4% for major and trace elements.
Clay Mineral Analysis
Clay mineral assemblages were analyzed by X-ray powder diffraction (XRD) at the State Key Laboratory of Marine Geology, Tongji University (Shanghai). Sample pretreatment and oriented mounts followed the methodology of Liu et al. (Reference Liu, Trentesaux and Clemens2003). Clay minerals were identified based on the position of (001) basal reflections. Four major clay mineral contents, including smectite (including mixed-layers) (15–17 Å), illite (10 Å), and kaolinite/chlorite (7 Å), were calculated according to semi-quantitative estimates of peak areas of the basal reflections using MacDiff software (Petschick et al. Reference Petschick, Kuhn and Gingele1996). No weighting factors were used when calculating the relative weight percentages of each clay mineral. The precision of replicate analyses of selected samples was±2% (2σ), and the accuracy of semi-quantitative evaluation of each clay mineral was ∼5%.
Radiocarbon Dating
A detailed 14C chronology for core YQ0902 has been reported by Shang et al. (Reference Shang, Fan, Yin, Burr, Zhang and Wang2018) and a complete set of 14C measurements for all four cores are reported by Shang (Reference Shang2018). AMS 14C measurements were obtained from Beta Analytic and the University of Arizona. The sample results reported here were from plant remains and charcoals. These were cleaned using a standard acid-alkali-acid pretreatment (Burr and Jull Reference Burr and Jull2010). All of the paleosol layers and overlying sediments were dated directly. 14C ages were also obtained from the underlying sediments in two of the cores. 14C dates reported in Shang et al. (Reference Shang, Fan, Yin, Burr, Zhang and Wang2018) were recalibrated here using the Calib Rev 7.04 program (http://calib.qub.ac.uk/calib/calib.html) with the IntCal13 dataset (Reimer et al. Reference Reimer, Bard, Bayliss, Beck, Blackwell, Ramsey, Buck, Cheng, Edwards, Friedrich, Grootes, Guilderson, Haflidason, Hajdas, Hatte, Heaton, Hoffmann, Hogg, Hughen, Kaiser, Kromer, Manning, Niu, Reimer, Richards, Scott, Southon, Staff, Turney and van der Plicht2013).
RESULTS
Two Paleosols from the Wenrui Plains
Each sediment core contained one paleosol. These were recognized by characteristic lithologic features, including: stiff clays, abundant yellow-brown and gray mottles, brown oxidized layers, and calcareous concretions—sometimes with brown oxidized cores. The 14C results show unambiguously that the two paleosols formed at different times (Table 2). Figure 3 summarizes the dating results. Cores YQ0901 and YQ0902 penetrated a paleosol dating to MIS 3, with a marked unconformity between the paleosol and Holocene sediments. Cores YQ0903 and YQ0904 penetrated a paleosol dating to the Early Holocene with no obvious age unconformity. Six of the dates were not in chronological order, which is typical of deltaic sedimentation where the opportunity for sediment reworking is high (Stanley and Chen Reference Stanley and Chen2000). Two of the outliers were reworked charcoals found in an erosional horizon at the unconformity just above the MIS 3 paleosol. Aside from these two outliers, all of the dates within the MIS 3 paleosol and underlying units date to MIS 3, and all of the dates from cores YQ0903 and YQ0904 date to the Holocene (Figure 3).

Figure 3 14C ages from the four cores. Depositional units (DU) are shown for the paleosols and adjacent units (see text). The MIS 3 paleosol (DU4) was found in cores YQ0901 and YQ0902, and the Early Holocene paleosol (DU1) was found in cores YQ0903 and YQ0904. Holocene sediments lie unconformably above the MIS 3 paleosol.
Table 2 4C dates from each paleosol unit, and adjacent units. The paleosol contained in cores YQ0901 and YQ0902 dates to MIS 3, and the paleosol contained in cores YQ0903 and YQ0904 dates to the Early Holocene.
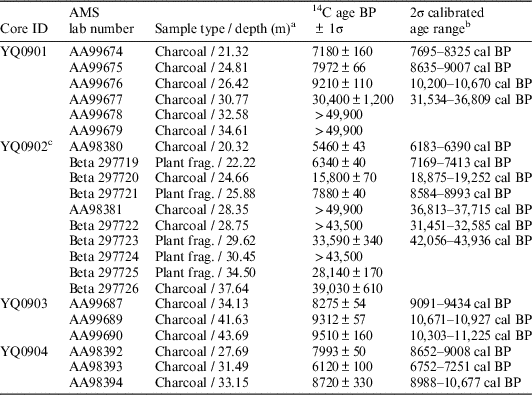
a Depths are meters from the top of the core.
b Finite 14C dates were calibrated with CALIB 7.04.
c 14C data for core YQ0902 are from Shang et al. (Reference Shang, Fan, Yin, Burr, Zhang and Wang2018).
Sediment Features of the YQ0902 Core
The sediments from core YQ0902 are shown in Figure 4. Depositional units (DU) are numbered from the base of the core upward. The depositional environment of the unit that underlies the MIS 3 paleosol (DU3) is lagoonal. This unit is gradational with the overlying paleosol. A conspicuous erosional surface defines the upper boundary of DU4, and this is overlain unconformably by Holocene sediments (DU5).

Figure 4 Core YQ0902 sediments, containing the MIS 3 paleosol.
DU3 (Lagoon): 32.16~30.26 m
DU3 consists of grey and dark grey massive mud with sandy streaks and mottles. Small plant fragments occur sporadically within the core, sometimes concentrated in laminae. The unit is nearly free of shell debris. The mean grain size of DU3 is 6.8~7.3 φ and the sand content is less than 6%. DU3 is rich in benthic foraminifera, with larger individual tests than neighboring units, but contains no marine ostracoda. This unit dates to the early-mid MIS 3 period (Shang et al. Reference Shang, Fan, Yin, Burr, Zhang and Wang2018).
DU4 (MIS 3 Paleosol): 30.26~29.15 m
DU4 is characterized by consolidated soils with mottled colors varying from brownish-yellow and grayish-yellow to light blue (Figure 4). The stiff texture and color of DU4 make it easy to differentiate from the depositional units above and below. Calcareous concretions are widely scattered within this unit, but plant and shell fragments are scarce. The mean grain size is 6.8~7.4 φ and the sand content is relatively low. The foraminiferal abundance is relatively high near the lower boundary but decreases rapidly upward in the core.
DU5 (Intertidal Flat): 29.15~25.07 m
DU5 consists of grey sandy mud and yellow-yellowish brown muddy sand with obvious bioturbation. The erosional surface at the base of the unit is overlain by thick alternating layers of coarse sand and mud (Figure 4). A fining-upward sequence is observed, and the color of the sediments change from yellowish brown near the bottom of the unit, to gray near the top (Figure 4). The mean grain size ranges between 4.6~7.2 φ, and the sand content varies markedly, from 0.1% to 49.6%. Plant and shell fragments are widely distributed throughout. Foraminifers, marine ostracoda and Sinocytheridea impressa are observed and gradually increase upward in the unit.
Sediment Features of the YQ0904 Core
Core YQ0904 is shown in Figure 5 as representative of the lithology of the Early Holocene paleosol and overlying sediments. In contrast to the MIS 3 paleosol, the contact with the overlying strata is gradational, with no obvious unconformity.

Figure 5 Core YQ0904 sediments, containing the Early Holocene paleosol.
DU1 (Paleosol): 32.09~36.11 m
DU1 is brownish-yellow and grayish-yellow unit that contains a 30-cm-thick section of dark grey sediments with shell fragments, centered around 34.65 m depth (Figure 5). We refer to sediments above this dark grey layer as the upper paleosol and those below as the lower paleosol. Compared to the overlying unit, the DU1 paleosol contains relatively stiff clays, but not so well-indurated as the MIS 3 paleosol. Minor wood debris and shells are scattered throughout the unit, and some concretions can be seen. One concretion, found at 32.45 m depth, is 6 cm long and 3 cm in diameter. The lower paleosol features well-developed mottles, with brown cores and yellowish rims. The upper paleosol exhibits weaker mottling.
DU2 (Marsh): 29.10~32.09 m
DU2 consists of massive grey silty clay with a few silty lamina. The lower part of this unit contains a large concretion and persistent mottles that are gradational with the underlying paleosol. A small amount of wood debris is scattered throughout the unit, along with some shell fragments. The gradual contact between DU2 and the underlying paleosol suggests an environment of near-continuous sedimentation.
Element Compositions of YQ0902 and YQ0904
Element ratios obtained by XRF core scanning and major element compositions from ICP-AES results, expressed as oxide percentages, are presented here for the paleosol layers in YQ0902 and YQ0904 (Table 3). Averaged elemental ratios, including: Al/Ti, Si/Ti, K/Ti, Ca/Ti, Mn/Ti, Fe/Ti, and Rb/Sr in DU3, DU4 and DU5, from core YQ0902, and DU1 and DU2, from core YQ0904, are shown in Figures 6 and 7, respectively.

Figure 6 XRF elemental abundance ratios (K/Ti, Ca/Ti, Mn/Ti, Fe/Ti, Al/Si, Rb/Sr) and Chemical Index of Weathering (CIW) profiles for core YQ0902, with depositional units DU3, DU4 and DU5.

Figure 7 XRF elemental abundance ratios (K/Ti, Ca/Ti, Mn/Ti, Fe/Ti, Al/Si, Rb/Sr) and Chemical Index of Weathering (CIW) profiles for core YQ0904, with depositional units DU1 and DU2.
Table 3 Major element ICPMS results from paleosols DU4 and DU1 in the YQ0902 and YQ0904 cores, expressed as oxide percentages.

XRF Results from the YQ0902 and YQ0904 Cores
Major elemental ratios in the basal unit of YQ0902 (DU3) are relatively uniform (Figure 6). By comparison, the overlying MIS 3 paleosol (DU4) exhibits significant down core variability, with Ca/Ti, Mn/Ti, Fe/Ti, and K/Ti concentrated in discrete layers (Figure 6). This variability stems from the presence of nodules and oxidized layers, seen in the core lithology (Figure 4). The Holocene sediments of the overlying layer, DU5, mark a return to uniform major element chemistry, with the exception of high Al/Si variability, and a highly oxidized basal layer between 29.0 and 29.15 m depth, immediately above the unconformity between DU4 and DU5.
A similar pattern is seen in the Early Holocene paleosol unit (DU1), at the base of the YQ0904 core. The variance observed in Ca/Ti, Mn/Ti and Fe/Ti in DU1 is notably higher than in the overlying unit (DU2). The variability is most notable in the upper paleosol (above 34.5 m depth), corresponding to a distinctly oxidized layer rich in Ca/Ti, Mn/Ti and Fe/Ti at about 32.25 m, and low K/Ti and Al/Si ratios throughout the upper section (Figure 7). The presence of mottles in DU1 have little influence on the major element chemistry. Ca/Ti values show a conspicuous increase in the grey sediment layer at about 34.5 m depth (Figure 5).
Both of the paleosols have high Rb/Sr values, as compared to adjacent units. In the MIS 3 paleosol in YQ0902 (DU4), Rb/Sr values are about 30% higher than in the enclosing units: DU3 below, and DU5 above (Figure 6). In the Early Holocene paleosol from the YQ0904 core (DU1), the Rb/Sr values are also about 30% higher than the adjacent unit, DU2 (Figure 7).
Clay Mineral Assemblage of the Early Holocene Paleosol in YQ0904
The clay mineral assemblages from depositional unit DU1, core YQ0904, are shown in Table 4. There is a distinct change in the proportions of clay minerals at about 34.2 m depth (2 m from the top of the paleosol), that corresponds to a decrease in Rb/Sr values (Figure 7). Illite is the dominant clay mineral in the top 2 m of the paleosol, with some smectite in the uppermost strata. The proportion of smectite diminishes rapidly down core, to 1%, and then climbs sharply again at 34.2 m depth. It reaches 49% at the base of the core and the rise in smectite in the lower paleosol is accompanied by a proportional drop in illite, chlorite and kaolinite.
Table 4 Clay minerals proportions in DU1 (Early Holocene paleosol, core YQ0904).

DISCUSSION
The paleosols from this study can be classified as calcic gleysols according to the taxonomic scheme of Mack et al. (Reference Mack, James and Monger1993). These are typical of environments with a high and fluctuating water table. Varying degrees of redox reactions and nodule growth are common among calcic gleysols, giving rise to yellow to grey coloration, a mottled texture, and brown, oxidized zones. This lithological interpretation is also consistent with the K/Ti, Ca/Ti, Mn/Ti, and Fe/Ti variations that we observe at discrete levels within both paleosols, where nodules have formed. For example, we observe a nodule at 29.5 m depth in the MIS 3 paleosol (Figure 4), and a similar nodule at 32.4 m depth, in the Early Holocene paleosol (Figure 5). Both of these depths correspond to sharp peaks in K/Ti, Ca/Ti, Mn/Ti and Fe/Ti (Figures 6 and 7).
The clay mineral data also point to pedogenesis. A distinct change in clay mineral assemblage is observed between 33.7 and 34.2 m depth in the YQ0904 core, at the divide between the upper and lower Early Holocene paleosol. Chlorite, kaolinite and illite are abundant in the upper paleosol, with very little smectite; whereas smectite becomes a primary clay mineral in the lower paleosol, along with illite. These features are consistent with a higher degree of chemical weathering in the upper paleosol.
Chemical weathering is a natural consequence of pedogenesis. In general, chemical weathering increases the relative amount of alumina in sediments, as clays form from feldspars, and calcium and sodium are removed by dissolution. This can be expressed as a chemical index of weathering (CIW, Harnois Reference Harnois1988):

Higher CIW values indicate a greater degree of chemical weathering. We used the ICPMS data in Table 3 to calculate CIW-depth profiles for both paleosols. The absolute values indicate significant chemical weathering, with a maximum within a meter of the top of each paleosol (Figures 6 and 7). The CIW curve for the Early Holocene paleosol shows two maxima, which suggests that it is a compound paleosol, with two periods of emergence. Compound paleosols are common in the Changjiang Delta, north of the study area (Chen et al. Reference Chen, Li, Li, Liu and Sun2008).
Rb/Sr values can also reflect the degree of chemical weathering in paleosols (Chen et al. Reference Chen, An and Head1999; Jin et al. Reference Jin, Wang, Shen, Zhang, Li, Ji and Lu2001; Hong et al. Reference Hong, Wang, Zeng, Gu, Wu, Yin and Li2013). Strontium dissolution in soils follows the same pathways as calcium, while rubidium follows potassium. Since these two elements are concentrated in different mineral phases, ensuing chemical weathering fractionates the two elements (Jin et al. Reference Jin, Cao, Wu and Wang2006). This is observed in both of our paleosols, with elevated Rb/Sr values, as compared to adjacent depositional units. The Rb/Sr trends from both paleosols mirror those of the CIW values, with a maximum value within a meter of the top of the paleosol (Figure 6R, Figure 7R).
The lithologic features, major element chemistry, clay mineral profiles, Rb/Sr profiles, and CIW profiles from DU1 and DU4 confirm their interpretation as paleosols. Since deposition occurred underwater and pedogenesis occurred after emergence, these units can be used to bracket sea level. The 14C dates allow us to place an upper limit on their depositional ages, and on the timing of pedogenesis. We can interpret this transition with reference to a eustatic sea level curve (Figure 8). The 2σ calibrated age range for the MIS 3 paleosol in core YQ0901 is 31.5 to 36.8 cal ka BP, and the 2σ range for YQ0902 is 36.8 to 37.7 cal ka BP, both measured on charcoal and both from about –27 m depth, relative to today’s sea level (Table 1). This date represents a maximum age for the paleosol, because the charcoal was incorporated into the sediments before emergence, and the upper surface of the paleosol has been eroded. Shortly before the charcoals were deposited in our two MIS 3 paleosols, between 40 ka BP and 38 ka BP, global sea level rose by approximately 20 m, from around –90 m to –70 m (Siddall et al. Reference Siddall, Rohling, Thompson and Waelbroeck2008), seemingly providing an environment for subaqueous sedimentation at our site. However, our paleosols are at a depth of about –27 m, above the expected sea level from measurements on corals and model values (Figure 8). This suggests a high regional sea level for the Oujiang Delta region, or possibly uplift. Regional sea level differences have been recognized for many years. Potter and Lambeck (Reference Potter and Lambeck2003) showed that differential isostatic rebound from melting glaciers can produce twenty meter differences depending on their location. Recent studies show that large regional effects can also be produced in the far field (low latitude sites responding to high latitude deglaciation) (Tamisiea and Mitrovica Reference Tamisiea and Mitrovica2011). If the offset we observe is indeed regional, then we should expect to find other anomalous relative sea level estimates along the southeast coast of China from the same time. A number of examples have been documented from the Changjiang Delta, about 450 km north of the Oujiang Delta. Relative sea level estimates based on sediments from the Changjiang coastal plain suggest a relative sea level between –5 m (Zhao et al. Reference Zhao, Wang, Chen and Chen2008; Wang et al. Reference Wang, Jones, Chen, Zhao and Zhan2013) and –10 m (Shi and Yu Reference Shi and Yu2003) during late MIS 3. Our Oujiang Delta relative sea level value lies between these Changjiang Delta values and the eustatic sea level curve (Figure 8).

Figure 8 Age-depth relationships of the MIS 3 (YQ0901 and YQ0902) paleosols and the Early Holocene (YQ0903 and YQ0904) paleosols, compared to relative sea level for the past 40 ka. The dark blue curve is the ice-volume equivalent sea level curve of Lambeck et al. (Reference Lambeck, Rouby, Purcell, Sun and Sambridge2014). The light blue shaded region is the range of values from four different relative sea level curves summarized by Siddall et al. (Reference Siddall, Rohling, Thompson and Waelbroeck2008). These include paleo sea level data from Chappell (Reference Chappell2002), Thompson and Goldstein (Reference Thompson and Goldstein2006), Arz et al. (Reference Arz, Lamy, Ganopolski, Nowaczyk and Pätzold2007), and Shackleton et al. (Reference Shackleton, Hall and Vincent2000). The cross is a U/Th date and inferred relative sea level from a coral that lived near the surface in Papua New Guinea (Cutler et al. Reference Cutler, Edwards, Taylor, Cheng, Adkins, Gallup, Cutler, Burr and Bloom2003). The Changjiang Delta relative sea level values for the end of MIS 3 (shown as stipled rectangle) are from Shi and Yu (Reference Shi and Yu2003), Zhao et al. (Reference Zhao, Wang, Chen and Chen2008), and Wang et al. (Reference Wang, Jones, Chen, Zhao and Zhan2013).
Both the Changjiang Delta and the Oujiang Delta are located adjacent to an actively subsiding continental shelf, impacted by a huge sediment load, brought by the Changjiang River. Both sites are affected because the river sediments are carried south along the coast once they reach the ocean, as part of a belt that extends for more than 1000 km (Liu et al. Reference Liu, Hsu, Yang, Wang, Wu, Du, Li, Chien, Lee, Yang, Zhu, Su, Chang and Huh2018). The outer Changjiang Delta has been subsiding at a rate of 1–2 mm a–1 since MIS 5 (Fang Reference Fang1959; Huang Reference Huang1959; Yu Reference Yu1959; Li et al. Reference Li, Chen, Zhang, Yang and Fan2000; Wang et al. Reference Wang, Jones, Chen, Zhao and Zhan2013), and continental shelf sediments opposite the Oujiang Delta are accumulating at a rate of 0.5–1 mm a–1 (Xu et al. 2012). The high regional sea level we observe in both deltas could be due to local uplift caused by subsidence-induced tilting of the coastal plain sediments (Wang et al. Reference Wang, Jones, Chen, Zhao and Zhan2013). Since the subsidence is greater offshore of the Changjiang Delta, the effect is more pronounced, as seen in the apparent late MIS 3 sea level elevations.
Unlike the MIS 3 paleosol, the relationship between sea level and the Early Holocene paleosol is straightforward (Figure 8). The Early Holocene paleosol developed nearshore, at a time of a fluctuating, stepwise, rising sea level. Fluctuating sea level led to a drop in sea level in the Early Holocene that exposed the sediments and initiated pedogenesis. The primary difference between the two paleosols is the duration of pedogenesis and the depositional environment. The duration of pedogenesis is the time that the paleosol was exposed to the atmosphere, and can be inferred from the eustatic sea level curve. According to the curve of Lambeck et al. (Reference Lambeck, Rouby, Purcell, Sun and Sambridge2014) the MIS 3 paleosol would have been many tens of meters above sea level during the LGM, and exposed for more than 20 ka. The Early Holocene paleosols must have formed near the shore, and were not exposed for longer than a thousand years. This timing explains the lithological differences between the two paleosols. In general, the MIS 3 paleosol is more mature. It is composed of stiff clays with distinct oxidized layers, and is capped by a conspicuous unconformity. The Early Holocene clays are relatively soft, with a mildly mottled texture and brown oxidized zones; and the upper surface of the Early Holocene paleosol grades continuously into the overlying sediments with no obvious unconformity.
Since these paleosols formed in response to global sea level rise, we should expect to see analogous examples elsewhere along the coast of China, and from other parts of the world. A prominent MIS 3 paleosol is widely recognized in hundreds of sediment cores from the Changjiang Delta (Chen et al. Reference Chen, Sun and Li1996; Chen and Li Reference Chen and Li1998; Li et al. Reference Li, Wang, Sun, Zhang, Fan and Deng2002). As in the case of the Oujiang paleosol, the Changjiang paleosol features stiff grey clay and a mottled texture. It is overlain unconformably by Holocene sediments and forms a stratigraphic marker from which the sequence stratigraphy of post-LGM sedimentation is widely referenced (Li et al. Reference Li, Wang, Sun, Zhang, Fan and Deng2002; Chen et al. Reference Chen, Li, Li, Liu and Sun2008; Song et al. Reference Song, Li, Saito, Okuno, Li, Lu, Hua, Li and Li2013). Further to the north, along the west coast of Korea, a MIS 3 paleosol dated to about 40–45 ka (optically stimulated luminescence), has been described by Chang et al. (Reference Chang, Kim and Yi2014). The authors note that this paleosol experienced a lengthy period of subaerial exposure. At Kyonngi Bay, on the west coast of Korea, two paleosols are observed in sediment cores: (1) a Late Pleistocene paleosol, and (2) an Early Holocene paleosol (Choi Reference Choi2005). The Late Pleistocene paleosol is capped by and unconformity and the Early Holocene paleosol has a gradational boundary with the overlying sediments, just as we observe in our cores from the Wenrui plain. Another interesting analog to our Holocene paleosol is the Shandong clinoform. This is a drowned deltaic front of the Yellow River that dates to the same period as our Early Holocene paleosol, and formed in much the same manner, in response to a stepwise rising sea level (Liu et al. Reference Liu, Milliman, Gao and Cheng2004).
CONCLUSIONS
Two paleosols of different age were found in sediments from the Oujiang Delta. The older paleosol (cores YQ0901 and YQ0902) dates to the latest MIS 3 period, with a maximum age of about 38 ka BP. This paleosol was exposed far above current sea level for perhaps 20 ka during the LGM. It features a mottled texture and stiff grey clays, with calcic concretions and brown oxidized layers rich in Mn and Fe. A similar MIS 3 paleosol has been described in numerous studies from the Changjiang Delta, where it serves as a regional stratigraphic marker. The younger Oujiang Delta paleosol (cores YQ0903 and YQ0904) dates to the Early Holocene, 9.2–11.2 ka BP. This paleosol formed nearshore in response to stepwise sea level increases and was probably not exposed for more than a thousand years. It has analogs along the west coast of Korea and is coeval with the Shandong clinoform. When plotted against a eustatic relative sea level curve, the Oujiang delta MIS 3 paleosol lies about 30 m above the global height. We interpret this difference to be caused by upward tilting of the Oujiang delta sediments, in response to active subsidence on the adjacent continental shelf.
ACKNOWLEDGMENTS
This research was funded by the National Natural Science Foundation of China (NSFC-41476031, 41776052), the China-ASEAN maritime cooperation fund (CAM-201501), and the National Programme on Global Change and Air-Sea Interaction (GASI-GEOGE-03). Also, Taiwan Ministry of Education Grant (NTU-107L9010). The authors would like to thank Yijing Wu, Yangyang Wang, and Lingling Chen for their assistance in both field and laboratory work.