Decreased maternal nutrient delivery to the fetus results in impaired fetal development and subsequent postnatal developmental problems(Reference Warner and Ozanne1). Several models of decreased maternal nutrition(Reference Yura, Itoh and Sagawa2, Reference Kumon, Yamamoto and Takahashi3) including protein restriction(Reference Zambrano, Bautista and Deas4–Reference Harrison and Langley-Evans6) have been studied in attempts to understand developmental programming – the process through which nutritional or other challenges during a critical window of fetal or neonatal development elicit persistent responses that produce long-term changes in offspring phenotype(Reference Langley-Evans7). There is compelling evidence that developmental programming results from poor maternal nutrition from human epidemiological(Reference Barker, Osmond and Golding8–Reference Fabricius-Bjerre, Jensen and Faerch10) and animal studies(Reference Minana-Solis Mdel and Escobar5, Reference Armitage, Khan and Taylor11–Reference Alexander13), where the variation in nutrient supply during early development appears to be a strong signal initiating adaptive developmental processes(Reference Nathanielsz14).
Developmental programming by poor maternal nutrition predisposes to adult obesity(Reference Yura, Itoh and Sagawa2, Reference Barker, Osmond and Golding8, Reference Poskitt9). In rodents, maternal protein restriction during gestation followed by accelerated postnatal growth is a risk factor for offspring obesity, hepatic steatosis, hypertension and insulin resistance(Reference Harrison and Langley-Evans6, Reference Brawley, Itoh and Torrens15–Reference Erhuma, Salter and Sculley17). The determination of the direct effect of a restricted diet on the developing fetus and neonate has been the central focus of many studies(Reference Zambrano, Bautista and Deas4, Reference Minana-Solis Mdel and Escobar5, Reference Erhuma, Salter and Sculley17, Reference Bellinger, Lilley and Langley-Evans18). In addition to the direct effect of a specific developmental challenge, lifestyle factors occurring in later life can act as a ‘second hit’ interacting with the initial programmed phenotype. Thus, nutritional restriction during pregnancy can interact with later-life offspring high-fat diet or hyperenergetic nutrition to produce further changes in offspring metabolic phenotype(Reference Yura, Itoh and Sagawa2, Reference Vickers, Breier and Cutfield19–Reference Yates, Tarling and Langley-Evans21). A few studies have been carried out to evaluate the effects of a second hit of high fructose in adulthood on programming by exposure to restricted diets during fetal development(Reference Cambri, Ghezzi and Ribeiro22). We hypothesised that a second hit resulting from increased offspring postnatal sugar consumption would lead to changes in adipose tissue function that are coupled to the initial effects of developmental programming due to a low-protein diet. Therefore, in the present study, we determined the effects of sugared water (5% sucrose in drinking-water) consumption on adult offspring adiposity as a ‘second hit’ following exposure to maternal protein restriction during pregnancy.
Materials and methods
Animal care and maintenance
Animals and diet
All animal procedures were approved by the Bioethics Committee of the Centro Tlaxcala de Biología de la Conducta of the Universidad Autónoma de Tlaxcala, according to the Mexican Guide for Animal Care. All rats were maintained under a 12 h light–12 h dark cycle at a controlled temperature of 18–22°C and humidity of 40 % and were given ad libitum access to food and water throughout the experimental period.
Maternal diet
The details of maternal protein restriction and animal maintenance have been published previously(Reference Zambrano, Rodriguez-Gonzalez and Guzman23). Briefly, twenty-two female Wistar rats aged 14 weeks and with a body weight of 200–240 g were mated with a proven adult male breeder. Upon confirmation of mating by the presence of a semen plug in the vagina, females were randomly allocated to one of two groups: a control group fed a 20 % casein diet and a protein-restricted group fed a 10 % casein diet throughout gestation. The two diets had the same fat and energy composition. At delivery (day 22), all the mothers were fed standard Purina Laboratory Chow 5001. Pregnant and lactating rats were weighed every day until the pups were removed at weaning on postnatal day 21.
Morphometric measurements at birth
Litter size and pup weight were recorded, and body length, head diameter, abdominal diameter and anogenital distance were measured using calipers. All litters were adjusted to nine or ten pups, and the sex ratio was maintained as close to 1:1 as possible. The pups were weighed daily during lactation.
After weaning, all the offspring were fed the standard chow diet Purina 5001 and weighed weekly. Since high sucrose intakes can affect food intake, we first carried out a pilot study to establish the effect of different sucrose intakes on food intake. Food intake was reduced by 74, 57 and 40 % when 30, 15 and 5 % of sucrose (respectively) was provided in the drinking-water. Therefore, we decided to use 5 % sucrose to minimise this effect. At 12 weeks of age, the pups were randomly assigned to one of two groups given the standard control diet alone or the standard control diet plus 5 % sucrose available ad libitum in the drinking-water (a higher concentration has been used in other studies)(Reference El Hafidi, Cuellar and Ramirez24, Reference Aguilera, Diaz and Barcelata25). Thus, four groups of each offspring sex were formed: offspring of mothers fed the control diet that remained on the control diet throughout the study (C); offspring of mothers on the restricted diet that remained on the control diet throughout the study (R); offspring of mothers maintained on the control diet that had access to sugar in the drinking-water in adulthood (C-S); offspring of mothers maintained on the restricted diet that had access to sugar in the drinking-water in adulthood (R-S). The offspring feeding protocol was followed for 10 weeks, during which body weight and water and food intakes were recorded daily and calculated weekly. Herein, we report only data obtained in the final week.
Metabolite measurements
At the end of the experimental period, glucose concentrations were measured in tail blood samples collected between 09.00 and 11.00 hours (Accutrend GCT analyser, Roche Diagnostics). The rats were decapitated using a rodent guillotine. Blood was collected, allowed to clot and centrifuged (3000 g for 10 min) to obtain serum.
TAG and cholesterol concentrations were measured using commercial kits obtained from Stanbio Laboratory, Inc. The intra- and inter-assay CV were, respectively, < 6 and < 7 % for TAG and < 4 and 4 % for cholesterol.
Insulin and leptin concentrations were measured by RIA using commercial rat kits obtained from Linco Research, Inc., as reported elsewhere(Reference Zambrano, Bautista and Deas4). The intra- and inter-assay CV were, respectively, < 4 and < 6 % for insulin and < 4 and < 5 % for leptin.
Fat depot measurements
The pericardial fat depot around the heart; gonadal fat around the epididymis or ovaries; visceral fat located inside the peritoneal cavity around the internal organs were dissected(Reference Roca-Rivada, Alonso and Al-Massadi26). All the fat pads were weighed. Adiposity index was calculated as total adipose tissue (g) divided by body weight (g).
Adipocyte size measurements
A random sample of visceral adipose tissue was fixed in neutral formalin (10 % formaldehyde and 0·1 m-phosphate buffer, pH 7) for 24 h at room temperature. The sample was embedded in paraffin, and serial 6 μm sections were cut using a microtome and stained with haematoxylin and eosin. Photomicrographs were obtained at a magnification of × 400 using an optical microscope (Axio Imager A1, Zeiss) equipped with an Olympus digital camera with a resolution of 5·1 megapixels. Adipocyte area was measured using the AxioVision Rel 4.6 (Zeiss Software, Inc.) software, and it is expressed as μm2. Adipocyte area was measured in cells completely enclosed within the field in six fields for each rat. Average adipocyte area was calculated for each rat within each group, and an overall mean of the averages was determined for comparisons among the groups.
Statistical analyses
Throughout the text, n 11 refers to pups from different litters. From each dam at random, one pup of the same sex was selected. Data are presented as means with their standard errors, unless stated otherwise, and analysed using one-way ANOVA for comparison of diets and two-way ANOVA for comparison of the combined effects of protein restriction and sucrose consumption. Maternal diet was considered as the first independent variable and sugared water consumption as the second independent variable. Where ANOVA indicated a significant (P< 0·05) effect of treatments, a post hoc test was carried out using the Bonferroni correction. Correlations were made using Pearson's correlation. Exact Fisher's test was used to compare the percentage of area according to adipocyte size bin. All statistical analyses were carried out using the program GraphPad Prism (version 5.01 for Windows).
Results
Maternal weight gain and food intake
Control mothers weighed 216 (sem 4) g at the start of gestation and 331 (sem 8) g at the end, a weight gain of 52 %, while the restricted mothers weighed 219 (sem 4) g at the start of gestation and 324 (sem 8) g at the end, a 48 % increase in body weight. These values were not significantly different.
Food intake during pregnancy was similar for both the groups: the control rats consumed 529 (sem 31) g during gestation, while the restricted rats consumed 542 (sem 31) g (P= 0·7).
Pups at birth
Maternal diet had no effect on litter size (C: 11·3 (sem 0·6) and R: 11·01 (sem 0·6), pups/litter) or pup birth weight, body length, and head and abdominal diameter at birth (Table 1). The only effect of maternal diet was the increase in the anogenital distance in both male and female offspring of the restricted mothers (Table 1).
Table 1 Morphometric measurements at birth (Mean values with their standard errors, n 11 litters)
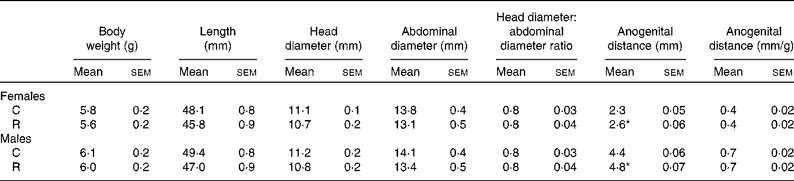
C, offspring of mothers fed the control diet; R, offspring of mothers fed the restricted diet.
* Mean values were significantly different from those of the control group of the same sex (P <0·05).
Offspring body weight and food intake before sugared water consumption
Within each sex, pup weight was similar during lactation. Offspring growth and food intake from weaning to 12 weeks of age did not differ in females and males among the groups. Before sugared water consumption, the control females weighed 219·3 (sem 7·8) g and the restricted females 210·9 (sem 7·8) g (P= 0·8), while the control males weighed 305·9 (sem 7·6) g and the restricted males 306·1 (sem 7·8) g (P= 0·9).
Body weight and water, food and energy intakes from 12 to 22 weeks
Sugared water consumption for a period of 10 weeks did not change body weight in either females or males. Groups that consumed water with sugar (C-S and R-S) increased their water consumption by 200 % and decreased their food intake by 40 %. As a result, total energy consumption did not change (Table 2).
Table 2 Food, water and energy intake measurements at 22 weeks of age and after 10 weeks of sugared water (SW) consumption (Mean values with their standard errors, n 11)
C, offspring of mothers fed the control diet; C-S, offspring of control mothers that drank SW (from week 12 to week 22); R, offspring of mothers fed the restricted diet; R-S, offspring of restricted mothers that drank SW (from week 12 to week 22); MD, maternal diet.
* Mean values were significantly different from those of the C group (P< 0·05).
† Mean values were significantly different from those of the R group (P< 0·05).
Metabolite measurements
In both male and female offspring, serum glucose concentrations were not altered by the sugared water challenge or the diet (Fig. 1). Sugared water consumption increased insulin, TAG and cholesterol concentrations in both the sexes. Sugared water consumption increased leptin concentrations in the restricted female offspring and in the control and restricted male offspring. Maternal protein restriction increased leptin concentrations in males in both the R and R-S groups; there was also an interaction between sugared water and maternal diet in male offspring with regard to leptin concentrations (Fig. 1).
Fig. 1 Serum metabolite concentrations of female ((a)–(e)) and male ((f)–(j)) offspring rats aged 22 weeks, after 10 weeks of sugared water challenge. □, Groups without sugared water; , groups with sugared water. Values are means (n 11), with standard errors represented by vertical bars. Analysis was by two-way ANOVA with Bonferroni's correction. * Mean value was significantly different from that of offspring of dams that had received the control maternal diet (P< 0·01; maternal diet effect). † Mean value was significantly different from that of the offspring that had not received the sugared water (P< 0·01; sugared water effect). ‡ Maternal diet–sugared water interaction (P< 0·01).
Fat depots
Sugared water consumption increased the amount of all the fat depots in both the sexes, except that of pericardial fat in male offspring (P= 0·09, Table 3). Maternal protein restriction increased all the variables, except gonadal fat in females. There were no interactions between sugared water and maternal diet with regard to the fat depots, except for visceral fat in females (Table 3).
Table 3 Fat depot measurements∥ (Mean values with their standard errors, n 11)
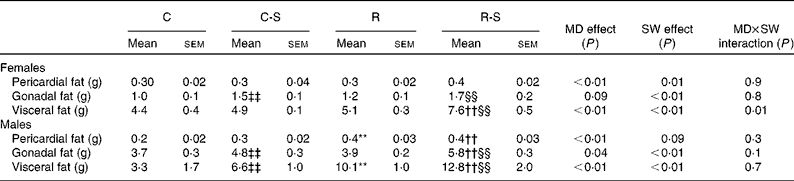
C, offspring of mothers fed the control diet; C-S offspring of control mothers that drank sugared water (SW); R, offspring of mothers fed the restricted diet; R-S, offspring of restricted mothers that drank SW; MD, maternal diet.
** Mean values were significantly different from those of the C group (P< 0·01, two-way ANOVA and Bonferroni correction).
†† Mean values were significantly different from those of the C-S group (P< 0·01, two-way ANOVA and Bonferroni correction).
‡‡ Mean values were significantly different from those of the C group (P< 0·01).
§§ Mean values were significantly different from those of the R group (P< 0·01).
∥ Rats at 22 weeks of age and after 10 weeks of SW consumption.
Sugared water consumption and maternal protein restriction increased the adiposity index in both the sexes, but an interaction between sugared water and maternal diet was observed only in female offspring (Fig. 2).
Fig. 2 Body weight, weight of fat pads and adiposity index of female ((a)–(c)) and male ((d)–(f)) offspring rats aged 22 weeks, after 10 weeks of sugared water challenge. □, Groups without sugared water; ■, groups with sugared water. Values are means (n 11), with standard errors represented by vertical bars. Analysis was by two-way ANOVA with Bonferroni's correction. * Mean value was significantly different from that of offspring of dams that had received the control maternal diet (P< 0·01; maternal diet effect). † Mean value was significantly different from that of the offspring that had not received the sugared water (P< 0·01; sugared water effect). ‡ Maternal diet–sugared water interaction (P< 0·01).
Adipocyte size and its relative distribution
Sugared water consumption increased adipocyte size in both male and female offspring (Fig. 3(a) and (f)). Maternal diet affected adipocyte size and showed an interaction with diet in females but not in males. Sugared water consumption increased the proportion of larger adipocytes in both female (Fig. 3(b)–(e)) and male (Fig. 3(g)–(j)) offspring.
Fig. 3 Adipocyte size of (a) female and (f) male offspring rats aged 22 weeks, after 10 weeks of sugared water challenge. □, Groups without sugared water; ■, groups with sugared water. Values are means (n 11), with standard errors represented by vertical bars. Analysis was by two-way ANOVA with Bonferroni's correction. * Mean value was significantly different from that of offspring of dams that had received the control maternal diet (P< 0·01; maternal diet effect). † Mean value was significantly different from that of the offspring that had not received the sugared water (P< 0·01; sugared water effect). ‡ Maternal diet–sugared water interaction (P< 0·01). Relative distributions of adipocyte size and representative photomicrographs: female offspring from control group (b), control group with sugared water (c), restricted group (d), restricted group with sugared water (e); male offspring from control group (g), control group with sugared water (h), restricted group (i), restricted group with sugared water (j). Analysis was by Fisher's test. * Mean value was significantly different from that of offspring of dams that had received the control maternal diet (P< 0·01; maternal diet effect). † Mean value was significantly different from that of the offspring that had not received the sugared water (P< 0·05; sugared water effect). (A colour version of this figure can be found online at http://journals.cambridge.org/bjn).
Correlation studies
In females, leptin concentrations were positively correlated with TAG concentrations. Leptin concentrations were correlated with adiposity in both the sexes and with adipocyte size in males (Fig. 4).
Fig. 4 Correlation of leptin and TAG concentrations in (a) females (P= 0·05; r 0·3) and (b) males (P= 0·3; r 0·2); correlation of leptin concentrations and adiposity index in (c) females (P= 0·04; r 0·3) and (d) males (P= 0·0001; r 0·8); and correlation of leptin concentrations and adipocyte size in (e) females (P= 0·5; r 0·1) and (f) males (P= 0·01; r 0·4). ○, Offspring of mothers fed the control diet; ●, offspring of control mothers that drank sugared water; ▽, offspring of mothers fed the restricted diet; ▾, offspring of restricted mothers that drank sugared water; n 44.
Discussion
Fetal undernutrition occurs throughout the world as a result of many different factors(Reference Poskitt9, Reference Godfrey, Barker and Robinson27, Reference Potera28). In developing countries, maternal diet is often specifically protein restricted or is globally energy deficient(Reference Victora, Adair and Fall29). This phenomenon is particularly prevalent in rural populations and areas where education levels are low(Reference Watson and McDonald30, Reference Zhang, Yi and Fang31). The developmental consequences of poor maternal and fetal nutrition have been addressed in many human epidemiological and animal research studies(Reference Zambrano, Bautista and Deas4–Reference Fabricius-Bjerre, Jensen and Faerch10, Reference Alexander13, Reference Nathanielsz14). Offspring exposed to poor fetal nutrition encounter many other challenges in their environment throughout life that may interact as a second hit with the programmed phenotype. For example, following poor fetal nutrition, increased food availability during lactation leads to catch-up growth and metabolic problems in later life(Reference Bieswal, Ahn and Reusens32, Reference Watkins, Lucas and Wilkins33). Greater nutrient availability due to migration or improvement of living standards with the incorporation of a Western-style high-carbohydrate diet may constitute a ‘second hit’ for individuals undernourished during development(Reference Lozada, Flores and Rodriguez34, Reference Thompson, McNeel and Dowling35). This combination of the ‘thrifty phenotype’(Reference Hales and Barker36) and later-life overnutrition may play an important role in the current epidemic of obesity worldwide, which is manifesting itself at very young ages(Reference Warner and Ozanne1).
The rat maternal protein restriction model has been used by many investigators(Reference Zambrano, Bautista and Deas4–Reference Langley-Evans7) and shown to predispose offspring to hypertension, dyslipidaemia and insulin resistance(Reference Minana-Solis Mdel and Escobar5, Reference Harrison and Langley-Evans6, Reference Brawley, Itoh and Torrens15) associated with increased serum TAG, cholesterol and leptin concentrations in adult offspring(Reference Zambrano, Bautista and Deas4, Reference Zambrano, Rodriguez-Gonzalez and Guzman23). As has been shown previously, in the present study, the protein-restricted mothers had a body weight similar to that of the control mothers during gestation(Reference Bellinger, Lilley and Langley-Evans18, Reference Bautista, Boeck and Larrea37). The only difference in the pups at birth was the increase in the anogenital distance; we have previously reported this difference for both male and female offspring. The probable cause for this is the increase in maternal serum steroid levels(Reference Zambrano, Rodriguez-Gonzalez and Guzman23, Reference Guzman, Cabrera and Cardenas38).
After weaning, the growth trajectory before sugared water consumption in offspring of the control and restricted mothers was similar to that reported previously(Reference Zambrano, Bautista and Deas4, Reference Erhuma, Bellinger and Langley-Evans20, Reference Zambrano, Rodriguez-Gonzalez and Guzman23). No differences in weight were observed between the groups since rats in the sugared water groups adapted to the energy in sugared water by reducing solid food intake. This is consistent with rodent models that use very high concentrations of sucrose in the water, e.g. 30 % sucrose(Reference El Hafidi, Cuellar and Ramirez24), or a high-fat content diet(Reference Erhuma, Bellinger and Langley-Evans20) and indicates that rodents regulate their total energy intake successfully at least for the period of the challenge imposed. Although rodents have been used extensively for this type of study, rodent species have some limitations since humans and other precocial species such as sheep tend to not regulate intake as precisely as rodents and gain weight in similar situations(Reference Metges39, Reference Wang, Ma and Tong40). In addition, the decreased chow intake will result in lower levels of protein and other nutrients in animals drinking sugared water. This pattern of food intake resembles that in migrants who were undernourished during their development and then moved to a society where relative proportions of carbohydrate and other nutrients in the diet change due to economics and availability(Reference Satia-Abouta, Patterson and Neuhouser41).
Sugared water consumption stimulated sufficient insulin secretion to maintain normoglycaemia and increased TAG, cholesterol and leptin concentrations in both the sexes. Studies carried out by Cambri et al. (Reference Cambri, Ghezzi and Ribeiro22) have reported similar findings, i.e. a fructose-rich diet increases TAG and cholesterol concentrations. The major production of endogenous cholesterol and TAG is from hepatic acetyl CoA from the metabolism of glucose and fructose(Reference Saltiel and Kahn42).
The present results show that sugared water consumption leads to hyperinsulinaemia in male and female offspring. Similar results have been reported in experimental models with high sucrose concentrations(Reference El Hafidi, Cuellar and Ramirez24, Reference Aguilera, Diaz and Barcelata25, Reference Oron-Herman, Kamari and Grossman43). By contrast, other researches have not found any difference in insulin concentrations in adulthood in offspring of mothers fed a protein-restricted diet during gestation after consumption of a fructose-rich diet(Reference Cambri, Ghezzi and Ribeiro22). Differences may be due to the form in which fructose is ingested, either directly as such or as present in the sucrose molecule.
In the study carried out by Cambri et al. (Reference Cambri, Ghezzi and Ribeiro22), high fructose consumption has been shown to impair body weight gain and reduce the weight of some adipose depots independently of the nutritional state during fetal life. By contrast, the present results indicate an accumulative effect of negative intra-uterine condition coupled to a second hit in adulthood, such as the observation of the increase in serum leptin concentrations in male offspring of mothers fed the protein-restricted diet and with sugared water consumption. Previous studies have shown that serum leptin concentrations in male offspring of mothers fed a protein-restricted diet are increased(Reference Zambrano, Bautista and Deas4, Reference Bautista, Boeck and Larrea37). The present results suggest a predisposition to hyperleptinaemia established by the prenatal stage with amplification by sugared water in the adulthood. Similar data have been reported by other authors using fetal undernutrition and postnatal hyperenergetic diet as experimental model(Reference Vickers, Breier and Cutfield19). This observation demonstrates leptin resistance associated with hyperleptinaemia and higher adiposity.
In the present model, the fat depots of all the groups were affected by maternal dietary restriction and sugared water consumption. Some reports have reported a correlation between maternal protein restriction intake and higher adiposity(Reference Erhuma, Bellinger and Langley-Evans20, Reference Chamson-Reig, Thyssen and Hill44). The present results indicate lower food intakes but similar energy intakes with higher adiposity and concentrations of insulin and leptin, which might play a role as lipogenic hormones that regulate metabolism and energy expenditure(Reference Otero, Lago and Lago45, Reference Bastard, Maachi and Lagathu46). These data are supported by the positive correlation found between serum leptin concentrations and adiposity index. Circulating leptin concentrations are proportional to adipose tissue mass.
In the present study, the increase in both body adiposity and serum leptin concentrations, which are clear indicators of obesity(Reference Lafontan and Girard47), in the 22-week-old offspring of restricted mothers subsequently exposed to 5 % sugared water indicates the synergic actions between fetal and neonatal environment and the second hit in adulthood.
One potential mechanism that may affect adipocyte size is increased hepatic TAG storage. Maternal protein restriction promotes the hepatic expression of PPARα RNA(Reference Lillycrop, Phillips and Torrens48), which together with PPARγ regulates adipocyte growth and metabolism(Reference Hihi, Michalik and Wahli49).
The present results suggest that there are sex differences related to adipocyte size. Oestradiol and testosterone are essential for the maintenance of energy homeostasis in both females and males(Reference Shi and Clegg50). Indeed, the effects of oestradiol through interactions with oestrogen receptors are relevant for carbohydrate metabolism, as has been described for rodents(Reference Shi and Clegg50–Reference Mauvais-Jarvis52). Further studies are required to determine whether sex differences in the increase in adipocyte size are related to androgenic and oestrogenic actions. In the present study, differences in the results obtained for male and female rats indicate an interaction between sex and metabolism(Reference Shi and Clegg50–Reference Mauvais-Jarvis52).
In summary, the present results suggest that adipocyte metabolism and development depend on both intra-uterine and postnatal nutritional conditions. We propose that the intake of carbohydrate-enriched beverages could promote the expression or accentuation of mechanisms that may have been programmed by adverse intra-uterine conditions but are accentuated when challenged by a second hit. Furthermore, there is the possibility that the latter effects are influenced by subject sex. Postnatal environmental factors are major enhancers to be considered in the aetiology of adult metabolic illness.
Acknowledgements
The authors thank Laura García Rivera, Iván Bravo, Eliut Pérez and Claudia Bautista for their excellent technical assistance. They also thank Miguel Acuña and Martín Serrano Meneses for editing the language of the manuscript.
The present study was partially supported by grants from CONACYT (105882 to M. M.-G., 155166 to E. Z., and 93 494 to M. C.-R.); PAPIIT-UNAM (228110 to M. M.-G.); PROMEP/UATx (UATLAX-233 to M. C.-R., Red de Cuerpos Académicos ‘Fisiología, Farmacología y Biología Molecular de la Conducta’); and Universidad Autónoma de Tlaxcala (Proyecto CACyPI-UATx-2013). The latter funders had no role in the design and analysis of the study or in the writing of this article.
The authors' contributions were as follows: M. C.-R. was responsible for data collection and management and manuscript writing; M. M.-G. was responsible for manuscript writing and obtaining funds; L. N., F. C. and E. C. collected and managed the data; P. W. N. was involved in study design and manuscript writing/editing; E. Z. was responsible for study design, manuscript writing/editing, and obtaining funds; J. R.-A. was responsible for study design, manuscript writing/editing, obtaining funds, project development.
None of the authors has a financial or personal relationship with other people or organisations that could inappropriately influence or bias the content of this paper.