According to the concept of energy balance (EB), energy intake must equal energy expenditure to maintain body weight(Reference Hill, Wyatt and Peters1). As a result, to lose body weight preferably in the form of body fat, energetic restriction (ER) is the prevalent therapeutic strategy(Reference Koliaki, Spinos and Spinou2). The theory of an asymmetric body weight regulation proposed by Mayer et al. in 1956(Reference Mayer, Roy and Mitra3) extends this paradigm by proposing that body weight control is more effective when EB is reached at a high energy expenditure and corresponding high energy intake (i.e. at a high energy turnover, ET). This theory is based on the analysis of dietary intake of 213 workers in West Bengal who had large differences in their physically demanding occupational activities. At a higher level of physical activity, a positive relationship was observed between energy intake and energy expenditure, whereas below a certain threshold of physical activity, a decrease in activity did not lead to a corresponding decrease in food intake. A low ET at a sedentary lifestyle may therefore pose a risk for weight gain. In line with this hypothesis, a recent observational study found that increasing energy expenditure rather than decreasing energy intake is more effective for reducing body fat(Reference Hume, Yokum and Stice4). The present study was, however, challenged because of methodological issues such as that the level of ET was defined only by energy expenditure in weight-stable individuals, and no adjustment of total energy expenditure (TEE) by RMR was performed(Reference Thomas and Westerterp5). Other authors, who adjusted TEE by RMR, did not find that a high ET provides protection against fat gain(Reference Saris, Blair and van Baak6, Reference Westerterp and Plasqui7).
Besides EB, fat balance is crucial for body weight control(Reference Flatt8) and may be improved by a higher ET. Correspondingly, subjects with medium or high fitness level had a better fat utilisation (lower respiratory quotient) than subjects with a low fitness level(Reference Shook, Hand and Paluch9). It is well known that an improved aerobic fitness positively affects the capacity to oxidise fat (maximal fat oxidation) during exercise(Reference Scharhag-Rosenberger, Meyer and Walitzek10, Reference Bircher and Knechtle11). However, little is known about the impact of acute changes in ET on 24-h fat oxidation and fat balance. Previous studies that investigated the acute effect of physical activity on fat balance under tightly controlled EB conditions in a metabolic chamber found no impact of exercise (≤60 min or 1674–2218 kJ/d) at the intensity of 40–70 % VO2max, performed in the postprandial state, on 24-h fat oxidation(Reference Iwayama, Kurihara and Nabekura12–Reference Melanson, Gozansky and Barry14). It is known that in particular physical activity at a medium intensity up to 55–65 % VO2max is able to enhance whole-body fat oxidation(Reference Romijn, Coyle and Sidossis15). A higher fat oxidation was found with low-intensity (33 % VO2max) and long duration (90 min) activity compared with moderate-intensity (66 % VO2max) and shorter duration (45 min) exercise of similar energy expenditure(Reference Thompson, Townsend and Boughey16). These observations were, however, made during physical activity performed in the fasted state.
The impact of a high ET obtained by prolonged physical activity (>60 min/d) with low intensity on 24-h fat oxidisation, fat utilisation and fat balance in the postprandial state remains unclear.
Improved regulation of fat balance is especially important in the condition of a positive EB. On a day-to-day basis or even shorter time periods, there is a continuous change between overfeeding (OF) and compensatory underfeeding or, conversely, between underfeeding and compensatory OF(Reference Edholm, Fletcher and Widdowson17). OF for example at weekends or holidays may lead to long-term weight gain if not compensated by subsequent underfeeding(Reference Racette, Weiss and Schechtman18, Reference Schoeller19) or by an increased energy expenditure.
Therefore, it is particularly interesting to investigate the impact of ET not only during zero EB but also during short-term over- and underfeeding periods.
We hypothesise that a higher ET obtained by long-duration physical activity of low intensity (walking at 4 km/h) beneficially affects 24-h fat oxidation and fat balance.
Thus, the aim of the present study was to investigate the impact of different levels of ET (obtained by different periods of low-intensity physical activity) on 24-h fat oxidation and fat balance (fat oxidation as a percentage of fat intake) during zero EB, ER (−25 % of energy requirement) and OF (+25 % of energy requirement). Therefore, a tightly controlled intervention study in a metabolic chamber was performed with three different levels of ET.
Subjects and methods
The present analysis included nine healthy men and was part of a larger trial that investigated the impact of ET on macronutrient balance, appetite control and glucose metabolism (clinicaltrials.gov as NCT03361566). Participants were recruited by notice board postings at the University of Hohenheim and Stuttgart as well as on the social media platform Facebook between December 2016 and January 2018. Exclusion criteria were food allergies or intolerances, alternative nutrition habits (e.g. vegetarian or low-carbohydrate diet), competitive sports, smoking, chronic diseases and regular use of medications. The study protocol was approved by the ethics committee of the Medical Council of Baden-Württemberg, Germany (F-2016-099) in accordance with the Declaration of Helsinki. All subjects provided written informed consent before participation.
Study protocol
The randomised crossover trial was conducted at the University of Hohenheim. An outline of the study protocol is given in Fig. 1. Participants underwent 3 × 3 24-h interventions in a metabolic chamber with three different levels of ET: (i) low, physical activity level (PAL) = 1·3 − 1·4, (ii) medium, PAL = 1·5 − 1·6 and (iii) high, PAL = 1·7 − 1·8. Each ET level was carried out at three levels of EB: zero EB, ER and OF (100, 75 or 125 % of individual energy requirement). Thus, in total, the study protocol consisted of nine intervention days. Different levels of ET were accomplished by walking on a treadmill (Kettler Track 9, KETTLER GmbH) with 4 km/h (2·49 min/h) for different time periods. During low ET participants were sedentary and did not walk on the treadmill. During medium ET, they walked for 3 × 55 min and during high ET for 3 × 110 min. The three interventions with different ET levels were separated by one washout day, and the three EB conditions were also separated by at least one washout day to avoid carry-over effects. A 3-d run-in period with controlled diet preceded the intervention phase. Low, medium and high ET levels as well as OF and ER were randomised by block randomisation. Therefore, the sequence of the three EB conditions was either ER-EB-OF or OF-EB-ER. Block randomisation was conducted by using computer-generated random numbers.
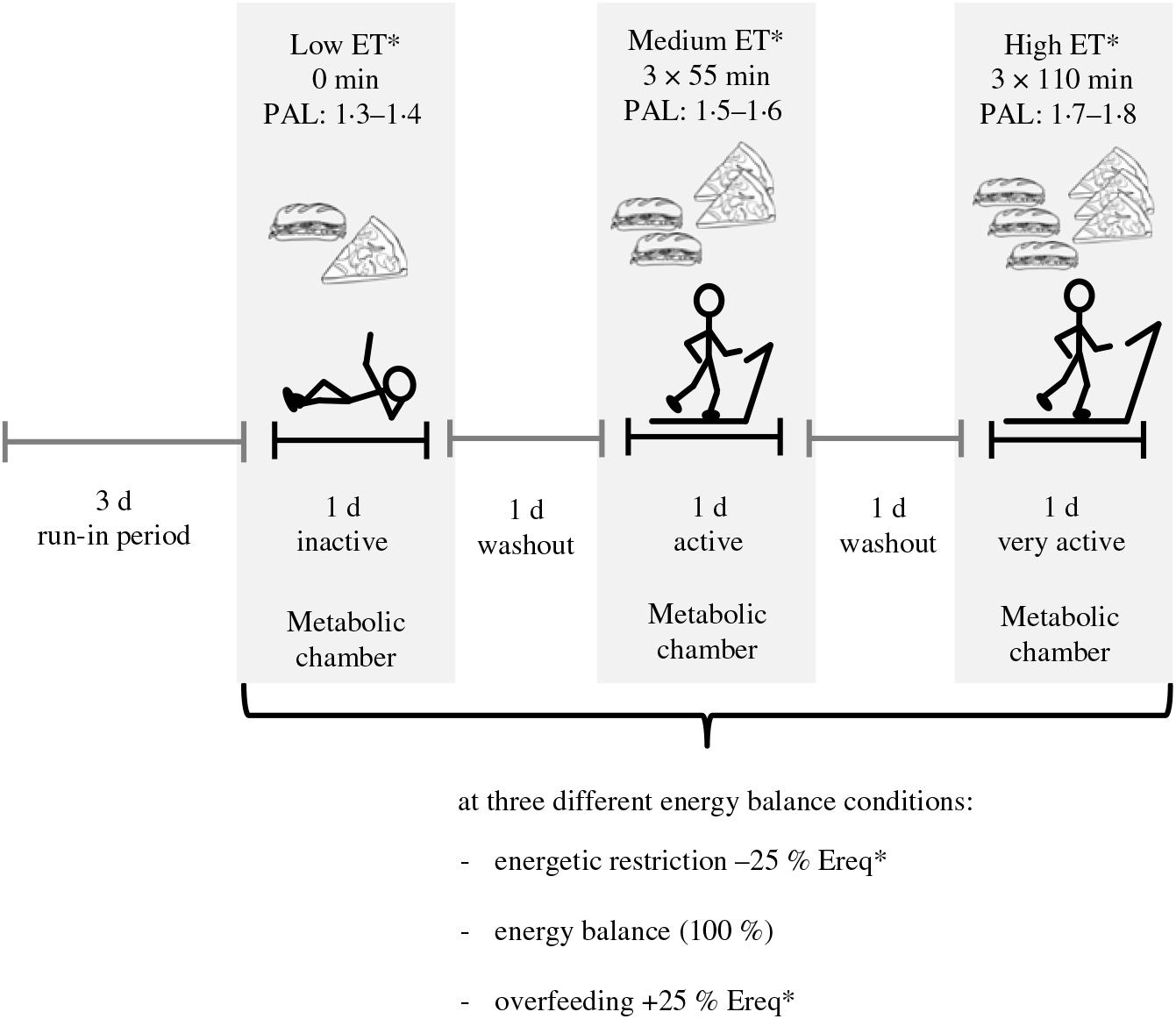
Fig. 1. Outline of the study protocol of a randomised crossover trial with 24-h interventions in a metabolic chamber with three different levels of energy turnover (ET): low, medium and high; each at energy balance, energetic restriction and overfeeding (100, 75 or 125 % of individual energy requirement). Different levels of ET were accomplished by walking on a treadmill with 4 km/h for various time periods (0, 3 × 55 min and 3 × 110 min). A 3-d run-in period with a controlled diet preceded the intervention phase, and ET level interventions were separated by one washout day. * Randomly assigned. PAL, physical activity level; Ereq, energy requirement.
The 24 h-interventions took place between 06.00 hours in the morning and 06.00 hours the following morning. Participants were admitted to the institute at 18.30 hours on the day before the 24-h intervention and spent the night before the intervention in the metabolic chamber. Participants left the morning after the intervention day (36 h length of stay in the metabolic chamber for one intervention day). On the washout day, they were allowed to go home for 12 h. During chamber days, participants followed a constant daily routine: wake up at 06.00 hours; meals at 07.00, 13.00, 19.00 hours and bedtime at 22.30 hours. Prescribed physical activity at medium and high ET was performed after each meal at 07.40, 13.40 and 19.40 hours. Blood samples were taken every 2 h during the intervention days between 07.00 and 21.00 hours using an intravenous catheter. Twenty-four-hour urine was collected during the intervention days.
Control of energy intake
All food during the study period was provided by the Institute of Nutritional Medicine. Participants were instructed to only consume the provided food and to only drink water and unsweetened herbal or fruit tea. Throughout the whole study period, macronutrient composition was kept constant with 50 % carbohydrate, 35 % fat and 15 % protein for each day and each meal. During the 24-h interventions, participants received the same food items on each day and were asked to eat all the provided food within half an hour. Individual energy intake was based on individual energy requirement for the three distinct ET levels. Individual energy expenditure for each ET was therefore measured in a pre-study-test prior to the actual study period by 3 × 24-h room calorimetry and by performing the actual prescribed physical activities for each ET level. On washout days, during the 3-d run-in period and during the pre-study test, food intake was ad libitum and leftovers were back-weighed to calculate dietary intake. Under the condition of ER, energy intake was calculated to be 25 % less than the energy requirement for the respective ET level and under the condition of OF 25 % greater than energy requirement. Diet composition was calculated by using Prodi®6 software (Wissenschaftliche Verlagsgesellschaft).
Control of physical activity
In advance of the study, it was tested in which walking time and walking speed were appropriate to reach the predetermined PAL. The walking speed was set at 4 km/h (2·49 min/h). On medium ET, participants walked 165 (3 × 55) min and on high ET 330 (3 × 110) min. Hence, they covered a distance of 11 km during medium and 22 km during high ET. Walking time, distance and speed were controlled with the software Kettler World Tours 2.0 (KETTLER GmbH).
Participants were asked to stay sedentary and to spend their time sitting at the desk or lying in bed during all interventions except for the walking session at medium and high ET. However, they were not allowed to sleep during the day. On the washout days and during the 3-d run-in period, participants were asked to refrain from exercise to avoid any impact of such activity on the outcome parameters(Reference Poehlman and Horton20, Reference Schenk and Horowitz21).
Throughout the entire study period, the step count per h was continuously measured using a triaxial activity monitor (ActivPAL, Paltechnologies Ltd). The data were analysed with the Software activPAL Professional v7.2.32. The ActivPAL was worn at the mid-line of the thigh, one-third of the way between hip and knee fixed with a waterproof tape according to the recommendation of the manufacturer. Because of technical problems with the ActivPAL device, the data of two participants are missing.
Anthropometry and body-composition analysis
Baseline anthropometry and body composition were obtained at baseline before the 3-d run-in period after an overnight fast. Height was measured with a stadiometer (Seca 274, seca GmbH & Co. KG). Body weight was measured on a calibrated impedance scale (Seca mBCA 515, seca GmbH & Co. KG). Fat mass (FM) was assessed using air displacement plethysmography via the BodPod Body Composition System (COSMED). Fat mass index and fat-free mass index were calculated as FM or FFM divided by the square of height (kg/m2).
Energy expenditure and macronutrient oxidation
The two respiratory chambers at the Institute of Nutritional Medicine at the University of Hohenheim each have a base area of 9 m2 and a total volume of 21 000 litres (D&S Consulting Services, Inc.). They are furnished with a day bed, chair and desk, computer with Internet access, telephone, toilet and sink. Air locks are used for the exchange of food and equipment (Life Science Technologies International LSTi). Fat oxidation and energy expenditure were determined at a constant flow of 120 litres/min fresh air through the metabolic chamber and by continuously measuring rates of O2 (${\rm{\dot V}}$O2) and CO2 (
${\rm{\dot V}}$CO2) concentrations on the exhaust side of the system using the Promethion integrated whole room indirect calorimeter system (Sable Systems International). The system consists of a GA-3m2 gas analyser and an FG-250 flow generator. O2 and CO2 concentrations (%) are measured to 0·001 % by two distinguished gas analyser chains, allowing ‘background baselining’ in order to compensate for analyser drift(Reference Melanson, Ingebrigtsen and Bergouignan22). O2 and CO2 concentrations were measured by a galvanised fuel cell analyser (Maxtec) and a non-dispersive IR analyser (Sable Systems International). Water vapour pressure of the sample gas stream was measured directly to 0·001 kPa by a capacitive humidity sensor, and the results are utilised to continuously correct the
${\rm{\dot V}}$O2 and
${\rm{\dot V}}$CO2, along with mass air flow (litres). The rates of VO2 and CO2 production were calculated using equations, originated by Brown et al. (Reference Brown, Cole and Dauncey23). Response time correction of the metabolic chambers to metabolic changes of the participant was performed by a z-Transformation mathematical model(Reference Lighton24). Data processing is performed using Sable Systems ExpeData software (version 1.9.51). Mean values were obtained from minute-to-minute intervals. Macronutrient oxidation and energy expenditure were calculated from
${\rm{\dot V}}$O2 and
${\rm{\dot V}}$CO2 and N excretion. N was calculated from urinary urea (1 g urea contains 46·7 % N), which was measured photometrically from 24-h urine and obligate N losses by faeces and skin were assumed to be +2·5 g N/d. Macronutrient oxidation was computed according to Jéquier & Felber(Reference Jequier and Felber25) by calculation of the non-protein respiratory quotient, whereby protein oxidation was calculated as 6·25 × g urinary N. TEE was calculated using the Weir equation (3·941 ×
${\rm{\dot V}}$O2 + 1·106 ×
${\rm{\dot V}}$CO2 – 2·17 × g urinary N)(Reference Weir26). Sleeping energy expenditure (SEE) was measured as reported by Schrauwen et al. as the lowest energy expenditure value of three consecutive hours during sleep between 24.00 and 06.00 hours(Reference Schrauwen, Van Marken Lichtenbelt and Westerterp27). Relative energy (rEB) and macronutrient balances (%) were calculated as percentage energy intake (EI) of respective TEE
$({\frac{{{E_I}}}\over{{TEE}}} \times 100)$ and as percent 24-h fat oxidation of respective macronutrient intake
$({{{{\rm{24 - h\;oxidation}}}}\over{{{\rm{intake}}}}}{\rm{\; \times 100}})$. In order to examine macronutrient utilisation (fuel partitioning), macronutrient oxidation as a percentage of TEE was calculated
$({{{{\rm{24 - h\;oxidation}}}}\over{{{\rm{TEE}}}}}\; \times 100)$. PAL was determined as TEE divided by resting energy expenditure (
${PAL = {{{\rm{TEE}}}}\over{{{\rm{resting\;energy\;expenditure}}}}}$; resting energy expenditure = SEE + SEE × 0·05).
Determinants of fat oxidation
NEFA in serum were measured photometrically, and total AUC (tAUC) was calculated for 14 h (07.00–21.00 hours).
Twenty-four-hour insulin secretion was derived from 24-h urinary C-peptide excretion by using the luminescence immunoassay method.
Adrenaline and noradrenaline excretions in 24-h urine were measured using liquid chromatography-MS. 24-h urine was acidified with hydrogen chloride within 6–7 h after the beginning of the 24-h sampling period.
Statistical analyses
Data are reported as mean values and standard deviations. The statistical software R (2017) was used to evaluate the data using an appropriate statistical mixed model(Reference Laird and Ware28, Reference Verbeke and Molenberghs29). The data were assumed to be normally distributed and to be heteroscedastic with respect to the different conditions of EB and levels of ET. These assumptions are based on a graphical residual analysis. The statistical model included the three EB conditions (EB, ER and OF) and the three ET levels (low, medium and high), as well as their interaction term as fixed factors. The ID was regarded as a random factor. The correlations of the measured values between several intervention days were taken into account (auto-correlation). Based on this model, a pseudo R 2 was calculated(Reference Nakagawa and Schielzeth30) and an ANOVA was conducted, followed by multiple contrast tests (for example, see Schaarschmidt & Vaas(Reference Schaarschmidt and Vaas31)) in order to compare the several levels of the influence factors. Comparisons were made between different levels of ET within the same EB condition as well as between different EB conditions within the same level of ET. For the three ET levels, all possible comparisons were considered (low to medium, low to high and medium to high). For the comparison of EB conditions, zero EB was considered as control and comparisons between ER or OF and EB were made. Deviations of the relative EB (%) from the values that were predetermined by the study protocol (100, 75 and 125 %) and deviations of relative macronutrient balances (%) from 100 (intake = oxidation) were tested by one-sample t test. Significance was set at P < 0·05. In order to calculate the appropriate sample size to detect differences in fat oxidation using a two-sided paired t test, data of Iwayama et al. (Reference Iwayama, Kurihara and Nabekura12) were used, who examined the impact of exercise timing on fat oxidation (mean values and standard deviations for fat oxidation, control: 1908 (sd 808) kJ/d; intervention: 3000 (sd 845) kJ/d). A total sample size of n 9 is required to assess these differences in fat oxidation at an α-level of 0·05 and a power of 80 % (hypothesised effect size = 1·3).
Results
Baseline characteristics of the study population are shown in Table 1. Nine men aged 20–32 years participated in the present study. BMI ranged between 19·7 and 26·1 kg/m2 and fat mass index ranged between 2·1 and 8·1 kg/m2. According to WHO criteria, two participants were overweight. Body weight and FM did not change during the course of the study (baseline: 74·6 (SD 10·2) kg and 19·1 (SD 4·5) % FM compared with 75·1 (SD 10·1) and 18·7 (SD 4·2) % FM at the end of the study, P > 0·05).
Table 1. Baseline characteristics of the study population (n 9)
(Mean values and standard deviations)

Comparisons of energy metabolism parameters and physical activity between conditions of EB and between ET levels are presented in Table 2. As determined by study design, energy intake (EI, MJ/d) differed between the three ET levels and between the EB conditions and TEE (MJ/d) increased with increasing ET level. EI was calculated to match TEE during EB. This was achieved at medium and high ET (rEB = 100 %, P > 0·05), but at low ET, EI was slightly higher than TEE (347 kJ, P < 0·05). Daily step count (steps/d) and PAL increased with higher ET within all EB conditions (Table 2). There was no difference in step count and PAL at the same ET level between the three EB conditions. SEE was higher with medium and high ET compared with low ET during EB and OF. During OF, SEE was also higher at high ET when compared with medium ET. During ER, SEE did not differ between the ET levels (Table 2).
Table 2. Comparison of energy expenditure variables and physical activity between the energy turnover (ET) levels and conditions of energy balance (n 9)
(Mean values and standard deviations)

EI, energy intake; TEE, total energy expenditure; rEB, relative energy balance; SEE, sleeping energy expenditure; PAL, physical activity level.
* P < 0·05 for comparison with low ET within the energy balance condition. Linear mixed model with multiple contrast tests.
† P < 0·05 for comparison with medium ET within the energy balance condition.
‡ P < 0·05 for comparison of the same ET level with energy balance.
Components of macronutrient metabolism are shown in Table 3. As determined, absolute intake of fat, carbohydrate and protein (g/d) increased with higher ET and differed between EB conditions, whereas macronutrient composition was constant by study design.
Table 3. Comparison of macronutrient intake and oxidation variables between the energy turnover (ET) levels and between conditions of energy balance (n 9)
(Mean values and standard deviations)

npRQ, non-protein respiratory quotient; FI, fat intake, FOX, fat oxidation; rFB, relative fat balance; FOX/TEE, percent fat oxidation of total energy expenditure; CHOI, carbohydrate intake; CHOOX, carbohydrate oxidation; rCHOB, relative carbohydrate balance; CHOOX/TEE, percent carbohydrate oxidation of total energy expenditure; PI, protein intake; POX, protein oxidation; rPB, relative protein balance; POX/TEE, percent protein oxidation of total energy expenditure.
* P < 0·05 for comparison with low ET within the energy balance condition. Linear mixed model with multiple contrast tests.
† P < 0·05 for comparison with medium ET within the energy balance condition.
‡ P < 0·05 for comparison of the same ET level with energy balance.
There were no differences in physical activity (steps/d) and food intake (energy content and macronutrient composition) on washout days between the study conditions (P > 0·05, data not shown).
Impact of energy turnover on fat oxidation
Fig. 2 shows the 24-h fat oxidation profile during all intervention days. Twenty-four-hour fat oxidation (24-h FOX, g/d) increased with higher ET during EB and OF (Table 3). During ER, 24-h FOX was higher at high compared with low ET only.

Fig. 2. Comparison of fat oxidation between the three energy turnover (ET) levels at energy balance (a), energetic restriction (b) and overfeeding (c) (n 9). Mean values are shown for 15 min intervals and standard errors only at every 30 min for clarity. Differences in the corresponding 24-h fat oxidation are reported in Table 3. , High ET;
, medium ET;
, low ET.
During EB and OF, relative fat balance (rFB) was higher at medium and high ET compared with low ET (Table 3). Fat oxidation as a percentage of TEE (FOX/TEE; %) was higher at high ET compared with low ET during EB and OF and compared with medium ET during OF. During ER, there was no difference in rFB between ET levels. FOX/TEE also did not differ between all ET levels (Table 3).
During EB and OF, fat balance was positive (fat intake was higher than 24-h FOX) at all ET levels (relative fat balance, rFB > 100 %, P < 0·05). During ER, fat intake equalled 24-h FOX at all ET levels (rFB = 100 %, P > 0·05).
Determinants of fat oxidation are shown in Table 4. tAUC for NEFA (mmol/l × 14 h) did not differ between the ET levels during EB and ER. By contrast during OF, NEFAtAUC was higher at high ET compared with low ET. Twenty-four-hour insulin secretion (C-peptide excretion) did not differ between levels of ET during EB and OF, whereas during ER, 24-h insulin secretion was lower at high ET compared with medium and low ET. There was no impact of ET on 24-h adrenaline and noradrenaline excretion at all EB conditions.
Table 4. Comparison of determinants of fat oxidation between the three energy turnover (ET) levels within the energy balance condition (n 9)
(Mean values and standard deviations)

tAUC, total AUC.
* P < 0·05 for comparison with low ET within the energy balance condition. Linear mixed model with multiple contrast tests.
† P < 0·05 for comparison with medium ET within the energy balance condition.
Impact of energy turnover on carbohydrate oxidation
Twenty-four-hour carbohydrate oxidation (24-h CHOOX; g/d) increased with increasing ET during EB, ER and OF (Table 3). Relative carbohydrate oxidation (rCHOB, %) did not differ between ET levels at all EB conditions. Carbohydrate oxidation as a percentage of TEE (CHOOX/TEE; %) did not differ between ET levels during EB, ER and OF (Table 3). Twenty-four-hour carbohydrate balance was negative (higher oxidation than carbohydrate intake; rCHOB > 100 %, P < 0·05) at all ET levels during EB and ER and at low ET during OF. During OF, 24-h CHOOX was equal to carbohydrate intake at medium and high ET (rCHOB = 100 %, P > 0·05).
Impact of energy turnover on protein oxidation
Twenty-four-hour protein oxidation (24-h POX; g/d) did not differ between ET levels during EB (Table 3). During ER and OF, 24-h POX was higher at high ET compared with low and medium ET. Relative protein balance (rPB; %) was lower at medium and high ET compared with low ET at all EB conditions. Protein oxidation as a percentage of TEE (POX/TEE; %) was decreased at medium and high ET compared with low ET as well as at high ET compared with medium ET during EB and OF. During ER, no difference was observed in POX/TEE between ET levels (Table 3).
During EB, protein balance was negative at low ET (higher oxidation than intake; rPB > 100 %, P < 0·05) and positive at a high ET (lower oxidation than intake; rPB < 100 %, P < 0·05). Protein balance was negative during ER and positive during OF irrespective of the level of ET (all P < 0·05). Twenty-four-hour protein oxidation was equal to protein intake only at medium ET during EB (rPB = 100 %, P > 0·05).
Discussion
In accordance with the proposed hypothesis, fat oxidation in percentage of intake was increased at medium ET (EB: +17 %, OF: +14 %) and high ET (EB: +23 %, OF: +17 %) compared with low ET during EB and OF (Table 3).
In contrast to the findings of the present study, several metabolic chamber studies of Melanson et al. showed no increase in 24-h fat oxidation with increased physical activity, when participants were in EB(Reference Melanson, Sharp and Seagle13, Reference Melanson, Gozansky and Barry14, Reference Melanson, MacLean and Hill32, Reference Melanson, Sharp and Seagle33). Because physical activity was performed in the postprandial state, the absence of an increase in 24-h fat oxidation in these studies was explained by an insulin-mediated decrease in lipolysis that diminished the supply of NEFA(Reference Horowitz, Mora-Rodriguez and Byerley34). NEFA availability in plasma is a major determinant of fat oxidation in muscle during and after physical activity(Reference Achten and Jeukendrup35). In addition, it has been suggested that increased insulin concentrations can directly inhibit the transfer of fat through muscle cell and mitochondrial membranes and hence inhibit intramuscular TAG oxidation(Reference Spriet36, Reference Coyle, Jeukendrup and Wagenmakers37). This is supported by a recent meta-analysis that has shown a higher fat oxidation during aerobic exercise (≤120 min) performed in the fasted state when compared with aerobic exercise after the ingestion of a meal(Reference Vieira, Costa and Macedo38). Another study found that only when exercise was performed before breakfast (fasted state), 24-h fat oxidation was increased(Reference Iwayama, Kurihara and Nabekura12). Furthermore, Schrauwen et al. investigated twelve healthy subjects in a metabolic chamber and showed that when participants underwent a glycogen lowering exercise session the day prior to the stay in the metabolic chamber, fat oxidation rapidly increased in response to a high-fat diet during EB(Reference Schrauwen, van Marken Lichtenbelt and Saris39). In line with these findings, activation of AMP-activated protein kinase that induces catabolic pathways is inversely correlated with glycogen content(Reference Kiens40).
These findings emphasise the importance of the timing of physical activity in relation to food intake and indicate that 24 h-fat oxidation can only be enhanced when physical activity is performed in a fasted state when glycogen stores in the muscle are depleted. In the present study, despite a higher food intake at higher ET (corresponding to a higher carbohydrate intake), 24-h insulin excretion was not increased (Table 4) presumably because of improved non-insulin-mediated glucose uptake by higher physical activity(Reference Jessen and Goodyear41). Therefore, although all physical activity sessions were performed during the postprandial phase (within 40 min after the beginning of the meal) lipolysis was not impaired as suggested by the fact that NEFAtAUC was not diminished at higher ET during EB but even increased at high ET during OF compared with low ET (Table 4). In summary, the increased 24-h fat oxidation and relative fat balance at higher ET occurred with physical activity in the postprandial state despite higher energy intake and is a unique finding that to the best of our knowledge has not been reported before.
The studies by Melanson et al. compared a day with a single exercise bout of 40–60 min or of 1674 kJ/d energy expenditure and 40–70 % VO2max to a sedentary control day. The exercise was performed by cycling on a stationary ergometer, and participants were lean sedentary, endurance-trained as well as obese sedentary men and women(Reference Melanson, Sharp and Seagle13, Reference Melanson, Gozansky and Barry14, Reference Melanson, MacLean and Hill32, Reference Melanson, Sharp and Seagle33). The main difference between these studies and the current protocol is the duration (40–60 v. 165–330 min/d), the mode of physical activity (cycling v. lower intensity walking) and the frequency of physical activity throughout the day (one v. three sessions). The discrepant findings suggest that not only exercise intensity and meal timing relative to the activity period are important factors to consider but also the frequency and duration of physical activity may affect 24-h fat oxidation and subsequent fat balance. It can be hypothesised that the duration of physical activity in the other studies was too short to affect 24-h fat oxidation. This assumption is supported by Smith et al., who investigated the adaptation to a high-fat diet and observed an accelerated increase in 24-h fat oxidation to a high-fat diet after 1 d, when physical activity was performed by walking two to three times per d on a treadmill with 4·8 km/h to reach a PAL of 1·8(Reference Smith, de Jonge and Zachwieja42). The frequency, mode, duration and intensity of physical activity in the study of Smith et al. (Reference Smith, de Jonge and Zachwieja42) are very similar to the present study. Newsom et al. have shown that an exercise session with 50 % VO2max and longer duration (70 v. 55 min) of the same energy expenditure performed in the afternoon was more effective to improve insulin sensitivity as compared with higher intensity (65 % VO2max)(Reference Newsom, Everett and Hinko43). Thus, maybe the combination of low intensity and long duration physical activity in the present study was superior to affect the impact of insulin on fat oxidation. Nevertheless, the current evidence on the impact of the intensity of physical activity on insulin sensitivity is inconclusive (for a review, see Bird & Hawley(Reference Bird and Hawley44)). Melanson et al. found no difference in 24-h fat oxidation between different intensities of physical activity (40 % VO2max v. 70 % VO2max) of the same energy expenditure performed in the morning(Reference Melanson, Sharp and Seagle13). In addition, it can be presumed that timing of physical activity in the present study had further protective effects in respect to the diminishing impact of insulin on fat oxidation. Circadian variations in insulin sensitivity occur with the highest insulin sensitivity in the morning and a decrease later during the day(Reference Bo, Fadda, Castiglione and Ciccone45). Therefore, especially the physical activity sessions after lunch and dinner had a higher potential to attenuate the insulin-derived inhibition of fat oxidation. In fact, postprandial insulin secretion after lunch decreased with higher ET during all conditions of EB (Buesing et al., unpublished results). Besides, the timing of physical activity, the frequency of physical activity in each postprandial period, might led to a cumulative attenuating effect of the inhibition of insulin on fat oxidation.
To address the mode of physical activity, walking as compared with cycling has shown to have a greater impact on fat oxidation (higher fat oxidation rates), maybe because walking involves the recruitment of a larger muscle mass than cycling(Reference Achten, Venables and Jeukendrup46). This larger recruitment of muscle mass itself may led to higher rates of fat oxidation as fatty acid translocase/CD36, a fatty acid binding protein is suggested to play an important role in particular for the short-term regulation of exercise-induced fat oxidation(Reference Kiens40). This protein was found to be located not only in the plasma membrane but also in the mitochondrial membrane and mitochondrial content of fatty acid translocase/CD36 was increased after 30 min of electrical stimulation of muscle compared with a non-stimulated control(Reference Campbell, Tandon and Woldegiorgis47). Thus, walking may also have a greater impact on insulin-independent glucose uptake by muscle contraction and may result in more effective insulin lowering.
Since the magnitude of fat oxidation during physical activity seems to depend on insulin(Reference Horowitz, Mora-Rodriguez and Byerley34) and glycogen levels(Reference Margolis, Wilson and Whitney48), it can be speculated that the observed effects of a higher ET on relative fat balance would be more pronounced, when physical activity sessions are performed in a fasted state, for example, before breakfast.
The present study showed that a medium ET increases relative fat oxidation during EB and OF. However, there was no further acute beneficial effect of a high ET, although TEE increased by about 1674 kJ between medium and high ET. The duration of 24-h might have been too short to measure the full extent of the impact of high ET on fat oxidation because the adaptation of fat oxidation to a higher fat intake needs several days(Reference Hill, Peters and Reed49). Thus, the dose–response relationship between physical activity of low intensity under the condition of varying ET and relative fat balance remains unclear.
In addition, a limitation of the present study is that the differences in relative fat balance were calculated compared with a very sedentary state (low ET), that may occur rarely in real life. Therefore, step count and fat oxidation for the low ET days were relatively low with 415–482 steps/d (Table 2) and 41 (SD 11)–51 (SD 14) g/d (Table 3). Nevertheless, small differences in fat oxidation were also observed between medium and high ET during EB and OF.
Remarkably, 24-h fat oxidation during EB increased with medium and high ET to the same level as during ER (Table 3). Because fat intake was still higher than fat oxidation at high ET during EB, the impact of increased ET on body FM needs to be evaluated in a long-term intervention.
Another important limitation of the study is that we investigated only young healthy men. Dynamics of fat oxidation in response to physical activity are different in women than in men(Reference Maunder, Plews and Kilding50) and may differ in obese compared with lean subjects(Reference Venables, Achten and Jeukendrup51) and with higher age(Reference Sial, Coggan and Carroll52). Therefore, the study results cannot be transferred to these population groups.
Because the intensity of physical activity was not objectively assessed, it cannot be excluded that at least for some of the participants, the intensity of physical activity was rather medium than low. Fat oxidation is known to increase from low to medium intensity with a peak at 45–65 % of VO2max(Reference Achten and Jeukendrup35). Thus, based on low-intensity physical activity, fat oxidation would have been lower as in the present results. Since the participants are all healthy, young and apparently fit men, it is however unlikely that the walking speed of 4 km/h, which is even slower than brisk walking, reached a medium intensity.
In contrast to EB and OF, during ER, 24-h fat oxidation was equal to fat intake independent of ET and relative fat balance was not further improved at higher ET (Table 3). Thus, there was no synergistic effect between ER and physical activity on relative fat balance.
Relative carbohydrate balance and carbohydrate oxidation as a percentage of TEE did not differ between the ET levels at all EB conditions (Table 3). Therefore, carbohydrate oxidation was still the main source of energy supply without a shift in contribution at medium and high ET.
A higher 24-h protein oxidation than intake was observed at low ET during EB. Relative protein balance shifted from a negative (>100 %) to a positive (<100 %) balance as ET became higher. This is in accordance with the study of Melanson et al., in which a higher protein balance has been found when the participants were exercising compared with a sedentary control day(Reference Melanson, Gozansky and Barry14). The increase in fat oxidation with higher ET was therefore accounted for by a decrease in protein oxidation. Partly, the concomitant increase in protein intake along with a higher ET accounts for the improvement in relative protein oxidation. During ER, relative protein balance was negative with all ET levels but was less negative with higher ET. These results suggest that muscle mass losses during short-term ER can be decreased with a high ET. This is in line with a 12-week and a 4-month intervention study showing that aerobic exercise attenuated the loss of skeletal muscle during ER(Reference Yoshimura, Kumahara and Tobina53, Reference Chomentowski, Dube and Amati54).
In addition to increased fat oxidation at higher ET, we observed a higher SEE with high and medium ET compared with low ET during EB (medium ET: +201 kJ/d, high ET: +335 kJ/d) and OF (medium ET: +318 kJ/d, high ET: +469 kJ/d). This suggests that a high ET facilitates a state of zero EB or reduces the magnitude of a positive EB during short-term OF. Consistently, other studies have shown increased REE values with a higher ET level(Reference Bullough, Gillette and Harris55–Reference Bell, Day and Jones58). Chronically elevated ET led to increased β-adrenergic receptor stimulation that may be mediated by a higher skeletal muscle sympathetic nerve activity(Reference Bell, Day and Jones58). Noradrenaline was found to be higher at high ET compared with low ET and was positively correlated with REE after excluding one single outlier(Reference Bullough, Gillette and Harris55). By contrast, in the present study, no difference in 24-h noradrenaline excretion was found between ET levels (Table 4). Higher SEE values with increasing ET may therefore rather be due to a protracted diet-induced thermogenesis at a higher energy content of the meals with higher ET. In line with this supposition, the magnitude and duration of diet-induced thermogenesis (DIT, in kJ) increase linearly with energy intake(Reference Quatela, Callister and Patterson59, Reference D’Alessio, Kavle and Mozzoli60). After consumption of a single mixed liquid meal (about 4105–4715 kJ) DIT lasted over 8 h(Reference D’Alessio, Kavle and Mozzoli60). In the present study, the average energy content of a single meal in the evening was 4046 kJ at medium ET and 4623 kJ at high ET during EB. Therefore, it can be presumed that the increase in SEE with a higher ET (during EB and OF) is a confounding effect of prolonged DIT. However, Paris et al. have shown that a high compared with a low ET led to a higher DIT as a percentage of energy intake in five out of six participants following a standardised breakfast(Reference Paris, Foright and Werth57). This suggests that energy dissipation in the DIT component of TEE might exist in response to a higher ET and could help to improve the regulation of EB. The extent of DIT (% of energy intake) was linked to SNS-activity(Reference van Baak61), and there is further evidence that DIT can be enhanced when exercise is performed after the meal compared with exercise before a meal(Reference Segal, Gutin and Albu62).
The medium and high ET interventions describe PAL of 1·5–1·6 and 1·7–1·8, respectively, which can be considered as feasible in daily life. In the present study, physical activity was achieved solely by walking because other spontaneous physical activities (i.e. householding) are limited in the confined space of the metabolic chamber. Therefore, the step count at medium and high ET (about 17 500 and 34 500 steps/d) is very high in respect to the PAL. Nevertheless, the study shows that a long duration physical activity of low intensity has an impact on fat oxidation.
Conclusion
The study results suggest that a higher ET obtained by prolonged physical activity of low intensity (walking with 4 km/h) has beneficial effects on body weight regulation when compared with a very sedentary state during EB and OF in young healthy men because relative fat oxidation was increased at the expense of protein oxidation. In contrast, during ER, relative fat balance was not further improved at higher ET. Thus, a higher ET can attenuate the adverse effects in particular of short-term OF on fat balance.
Acknowledgements
We thank all the volunteers for their participation.
The present study was funded by budgetary resources of the University of Hohenheim and received no specific grant from any funding agency, commercial or not-for-profit sectors.
The authors’ contributions were as follows: A. B.-W. designed the research study; A. N., F. B., F. A. H. and A. B.-W. performed the research; A. N. analysed the data; A. N., F. B., F. A. H., M. J. M. and A. B.-W. discussed the results; M. H. provided statistical support; A. N. and A. B.-W. wrote the manuscript; A. N. and A. B.-W. had primary responsibility for final content. All authors read and approved the final manuscript.
There are no conflicts of interest.