Impact statement
Services provided by water lifeline infrastructure (e.g., drinking water, wastewater, and stormwater [DWS] systems) are critical to modern societies. However, stresses from aging and external threats (e.g., sea level rise, floods, earthquakes) on existing DWS systems and the current practice of siloed governance and management of DWS systems have worsened the vulnerabilities in many communities, especially in the marginalized ones. This paper presents a new framework on managing the resilience of DWS systems in the water sector to three major hazards (Sea-Level Rise, Earthquake, and Cyberattack). The new framework embraces a social–ecological–technical system-of-systems approach and a whole-life approach to allow communities to better understand and operationalize short-term to long-term resilience in their DWS systems. The framework also endorses the integration of the goals of sustainability and resilience for overcoming global water challenges, and provides insights via case studies on how communities could identify technologies and policies that promote both goals in the near term and in the far future. Finally, a case is made for using emerging digital technologies and Artificial Intelligence to operationalize the proposed framework in communities. We should consider how general trends, and digital technology including digital twins and artificial intelligence and machine learning, as well as cloud and edge computing offer opportunities for the future of sustainable and resilient water infrastructure systems.
Introduction and background
Promoting resilience is a growing need due to the increased frequency and magnitude of distruptive events affecting the lifeline infrastructure systems that support communities. Services provided by water lifeline infrastructure (e.g., drinking water, wastewater, and stormwater [DWS] systems) are critical to modern societies. Herein, we refer to the water sector as consisting of drinking water, wastewater, and stormwater systems. The Drinking water systems provide potable water for consumption. Wastewater systems include sanitary systems that collect industrial and consumer-used water for cleaning and disposal. Stormwater systems that collect surface runoff, and may be contaminated by human activities, for cleaning and disposal. In some urban areas, the sanitary and storm systems are managed as combined systems. Collectively, these three systems in the water sector are defined as DWS systems in this paper. Major cities, which are the economic backbone of the nation, cannot exist without access to safe water sources, and the ability to treat wastewater, and return water to the environment (Sinha and Graf, Reference Sinha and Graf2014).
The increasing interest in resilience has resulted in numerous definitions. The National Academy of Sciences (NAS) defines resilience as ‘the ability to prepare and plan for, absorb, recover from, or adapt to actual or potential adverse events’ (NRC, 2012). As a common thread to all definitions, resilience is seen as the ability of a system to withstand external perturbation(s), adapt, and rapidly recover to the original or a new level of functionality. However, we believe that resilience should be conceptualized not only in relation to a perturbation, but as part of the entire life of a system. Resilience is a function of the state of the system at any time. As a result, improving resilience also means improving the reliability of the system under normal and also under stressed conditions. Although there is significant high-quality information available on resilience-related topics (e.g., hazard and vulnerability assessment, risk assessment and management, and loss estimation, as well as disaster resilience itself), there is no integration framework that also provides a central source of data and models/tools to the owners and managers of water infrastructure systems, community planners, policymakers, and other decision-makers. Such a framework can help in defining and measuring the resilience throughout the lifetime of infrastructure systems. Developing a better description of resilience and metrics and tools for defining and measuring the resilience DWS infrastructure system is an important step in meeting the challenge of water sector resilience at the community scale.
Resilience for the water sector requires a ‘Social–Ecological–Technical System-of-Systems’ and ‘Whole-Life’ approach to mitigating risks from disruptive events and improving long-term resilience driven by societal needs for sustainability, and social and environmental justice. The Social–Ecological–Technical System-of-Systems approach considers all interactions and interdependencies among subsystems of the water sector in a holistic way. The Whole-Life approach considers the lifespan of infrastructure systems in activities related to planning, design, operations, and renewal of the infrastructure. Resiliency in the water sector integrates and derives information from cyber-space, physical-space, and social-space and the underlying interdependencies within water system-of-systems to improve the overall management of risks from extreme events and optimize life-cycle management. Such considerations facilitate equitable, affordable, efficient, reliable, sustainable, and resilient provision of water infrastructure services, and as a result, sustain long-term health and economic productivity of communities (NSF ERC Policy and Governance Workshop, 2021).
Measuring the resilience of water sector systems and communities, however, poses difficult technical challenges due to a lack of adequate:
-
(1) understanding of how natural processes in the environment that interact with DWS infrastructure systems can lead to stresses and failures over time;
-
(2) use of predictive technologies and information on hazards and mitigation strategies by design professionals, standards developers, and emergency managers, for the purpose of promoting resilience-risk-informed behavior, improving performance-based management of complex spatially distributed DWS networks, and accelerating the transfer of results of research into practice; and
-
(3) standardized methods to assess the resilience of water systems and communities to sudden disasters and chronic stresses during the whole life of water infrastructure systems.
It is already difficult enough to assess the resilience of an individual infrastructure asset. Considering the interdependencies among multiple assets creates additional levels of complexity that have long exacerbated the difficulties in managing interconnected natural, built, and social water systems. It is also important to consider dependencies and interdependencies with other sectors (i.e., energy, agriculture). While there are theories and models for disaster resilience, system reliability and vulnerability analysis, and emergency management, there remain fundamental and methodological gaps related to the analyses of integrated resilient systems. For example,
-
(1) Efforts are needed to increase the discoverability and accessibility of data on integrated systems. This will help communities understand appropriate uses of data for resilience planning, increase compatibility with community software platforms and models, and standardize data to facilitate the application for utilities, practitioners, and researchers. The development of common data standards and best practices for the curation and dissemination of validated data and tools would reduce the technical burden on utilities as they support community infrastructure.
-
(2) Available tools continue to be inadequate in their ability to conduct big-data analytics for resilience, conduct a comprehensive assessment of consequences and vulnerabilities, and employ time-varying multi-objective optimization and community-level platforms for long-term decision support. Additionally, regional resilience tools often are limited in engaging and collaborating with end-users to align the technical requirements of analytical tools to community decision-support needs.
The water sector should develop a synthesizing framework capable of articulating the explicit inputs for the resilience analysis of DWS infrastructure. The framework needs to consider different adverse event scenarios (e.g., acute service disruptions, chronic stress like aging and deterioration, and uncertain natural hazards and malevolent threats); the support of different resilient management processes (e.g., during pre-event mitigation and post-event recovery), the incorporation of flexible and robust engineering practices; and integration with various quantitative modeling approaches and qualitative analysis methods. This includes the integration of resilience plans into long-range infrastructure plans and the use of innovative technologies for system vulnerability assessment. This will lead to increased service life, minimized disruptions, and faster and less costly response and recovery after the events. Advancements in fundamental knowledge and measurement science will bring tangible changes to the practice with a clear societal impact.
The main goal of this paper is to draw extensively on previous resilience-related work undertaken for infrastructure in general, and in particular in the water sector, for the purpose of:
-
(1) Examining current practice and gaps in the water sector for management of resilience to sea level rise, earthquakes, and digital hazards.
-
(2) Identifying future research needs that are driven by a whole-life approach to managing resilience of interconnected social, built, and natural water sector systems.
Water sector infrastructure systems
There are over 155,000 public drinking water systems, and over 16,500 publicly owned wastewater facilities in the U.S. (AWIA, 2018). However, the majority of the population is served by a small number of mostly large or very large systems that are predominantly owned and operated by municipalities in the United States (AWWA, 2011). While individual utilities vary widely in size and complexity, Figure 1 shows a typical water flow path through drinking water and wastewater infrastructure under normal operations (NIAC, 2016). Drinking water and wastewater systems are some of the most important sectors for ensuring and protecting the health of the nation.
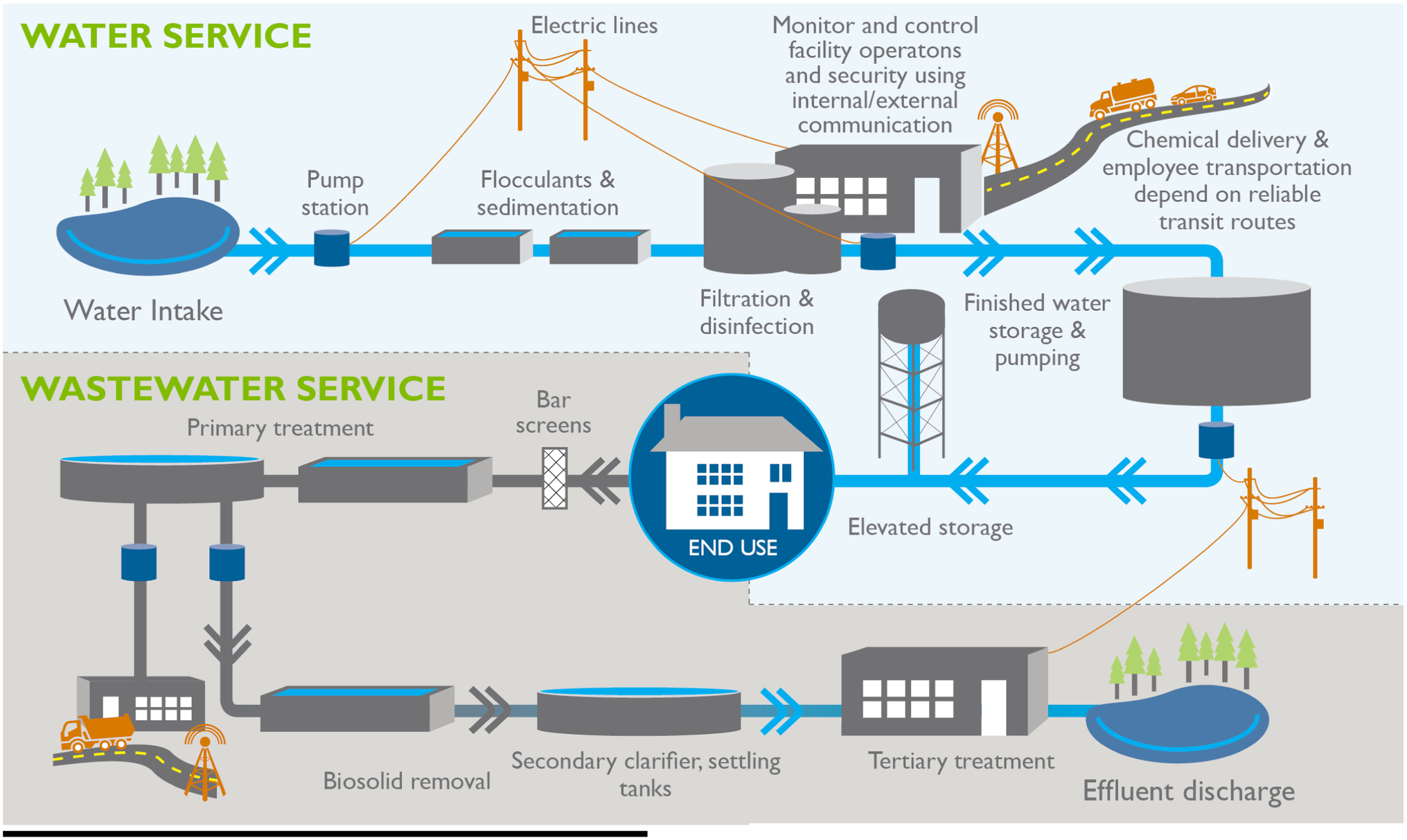
Figure 1. Typical drinking water and wastewater operations. Source: NIAC.
The crisis in Flint, Michigan (MDAG, n.d.), and the crisis in post-hurricane Puerto Rico (Vick, Reference Vick2023) reveal how a loss of safe drinking water can devastate entire communities, regions, and even countries. Also, the loss of water services can cripple other critical infrastructures and trigger additional disruptions with significant cascading effects. An analysis of vulnerability assessments conducted by the U.S. Department of Homeland Security (DHS) Office of Cyber and Infrastructure Analysis (OCIA) (USDHS, n.d.) revealed that among surveyed critical infrastructure that depend upon water for core operations, the services degraded 50% or more within 8 h of losing drinking water services. Figure 2 shows the impacts on interdependent critical facilities when the water infrastructure system is unable to provide the desired level of service. Further, operational costs, capital costs, and increasing/expanding regulation are also included among the top five water sector issues (NIAC, 2014).

Figure 2. Infrastructure interdependencies on water sector. Source: NIAC.
A system-of-systems and whole-LIFE approach
Resilience is a seemingly difficult concept to define due to the many perspectives that exist in different fields (e.g., engineering resilience (Pimm, Reference Pimm1984), ecological resilience (Holling, Reference Holling1996), social-ecological resilience (Carpenter et al., Reference Carpenter, Walker, Anderies and Abel2001), social resilience (Adger, Reference Adger2000), development resilience (Pasteur, Reference Pasteur2011; Barrett and Constas, Reference Barrett and Constas2015), socio-economic resilience (Mancini et al., Reference Mancini, Salvati, Sateriano, Mancino and Ferrara2012), community resilience (Norris et al., Reference Norris, Stevens, Pfefferbaum, Wyche and Pfefferbaum2008), and psychological resilience (Tugade et al., Reference Tugade, Fredrickson and Barrett2004). Further, not every hazard occurs in the same geographical region of the U.S. For instance, cities on the west coast are more vulnerable to earthquakes so their resiliency definition and plans incorporate damages that result from earthquakes (i.e., liquefaction). Cities on the east coast include resiliency definitions leaning toward hurricanes. The metrics for measuring resiliency are based on the size of expected degradation in the quality of infrastructure and requires knowledge of robustness, redundancy, resourcefulness, and rapidity to recovery, as presented in Figure 3. The water sector resilience framework should, therefore, consider a system-of-systems approach that takes into account the complex interactions and interdependencies in the water infrastructure systems. This perspective is inspired by the SETS (Social–Ecological–Technical systems) framework which highlights the importance of coordinating natural, built, and social systems for water management, and understanding their interactions and the factors that affect urban ecosystem services (Mukheibir et al., Reference Mukheibir, Howe and Gallet2014; Chester et al., Reference Chester, Grimm, Redman, Miller, McPherson, Munoz-Erickson and Chandler2015; FAO and WWC, 2018; Hager et al., Reference Hager, Mian, Hu, Hewage and Sadiq2021; Pokhrel et al., Reference Pokhrel, Shrestha, Hewage and Sadiq2022). The SETS framework also highlights the importance of hybridity in infrastructure, which is the built environments coupled with landscape-scale biophysical structures and processes.

Figure 3. Functionality curve for water sector infrastructure systems. Source: NIST.
The Presidential Policy Directive (PPD)-21 (White House, 2013) identified 16 critical infrastructure sectors whose assets, networks, and physical conditions are vital to the security, national economy, and public well-being. A large portion of the critical infrastructure systems in the U.S., such as water systems, was built before some of the modern risk and resilience issues were identified. There are inherent vulnerabilities in such legacy systems. The resilience of critical infrastructure has become a particularly important discussion topic after recent disasters that crippled regional infrastructure and left many communities stranded without basic infrastructure and utilities services, or access to emergency facilities. To address the need to enhance resilience, federal agencies, as well as states and cities, have developed conceptual guidelines for the assessment and improvement of resilience at the community and regional level scale (Walpole et al., Reference Walpole, Loerzel and Dillard2021). Figure 4 shows potential hazards in the United States, illustrating the importance of multi-hazard community resilience, preparedness, and planning. Meanwhile, the state of the practice for critical infrastructure asset management has been predominantly corrective-reactive. Most of the focus has been on condition as a basis for maintenance and operation of the asset inventory, rather than addressing design and operation practices to improve performance and resiliency of the network at the level of the system.

Figure 4. National hazard map for the United States. Source: USDHS.
To shift this paradigm, many federal, state, and local agencies as well as research institutions have initiated programs to develop guidelines for modern performance-based approaches to ‘system-level and whole-life’ management of infrastructure systems. Another notable gap in science and practice is the consideration of interdependencies (either internal within the sector system or external with other sectors). It is critical to include an analysis of disruptions that may originate in one sector and cascade into another sector. System-wide resilience management with a systematic focus on life-cycle resilience can deliver safe, efficient, survivable, and reliable water systems. The proposed approach is driven by the transformative integration of existing technologies, knowledge, data, models, and tools across related and disparate disciplines and facilitated by fundamental science and knowledge for water sector infrastructure resilience.
The increasing reliance on digital capabilities to operate water systems makes them more efficient and secure against traditional threats such as extreme events and physical failures. At the same time, digitalization can create additional vulnerabilities in the system and can expose the water sector to cyberattacks. While cyberattacks remain responsible only for a small fraction of water service disruptions, the potential damage is significant and increasing quickly. We should consider digital resilience as encompassing the various ways the water sector uses digital tools and systems to quickly recover from or adjust to crises, including cyberattacks. During the COVID-19 pandemic, a common water sector response was to turn to digital technologies to maintain certain levels of activity and service delivery during the pandemic (OECD, 2020). This included the use of digital platforms and the implementation of intelligent sensor and communication technologies. A continued dependence on digital platforms also means that the utility workforce now needs new training paradigms in order to effectively use current and upcoming new technologies and protect themselves from ever-increasing malicious attacks. Resilient technologies, workforce and cyberinfrastructure managed by utilities can also serve a necessary inputs to trustworthy data analytics and decision support systems that can ultimately create a resilient water sector and society. A conceptual digital resilience framework for water sector information systems is shown in Figure 5 (Bodeau et al., Reference Bodeau, Graubart, Heinbockel and Laderman2015; USDHS, 2015; NIST, 2018).
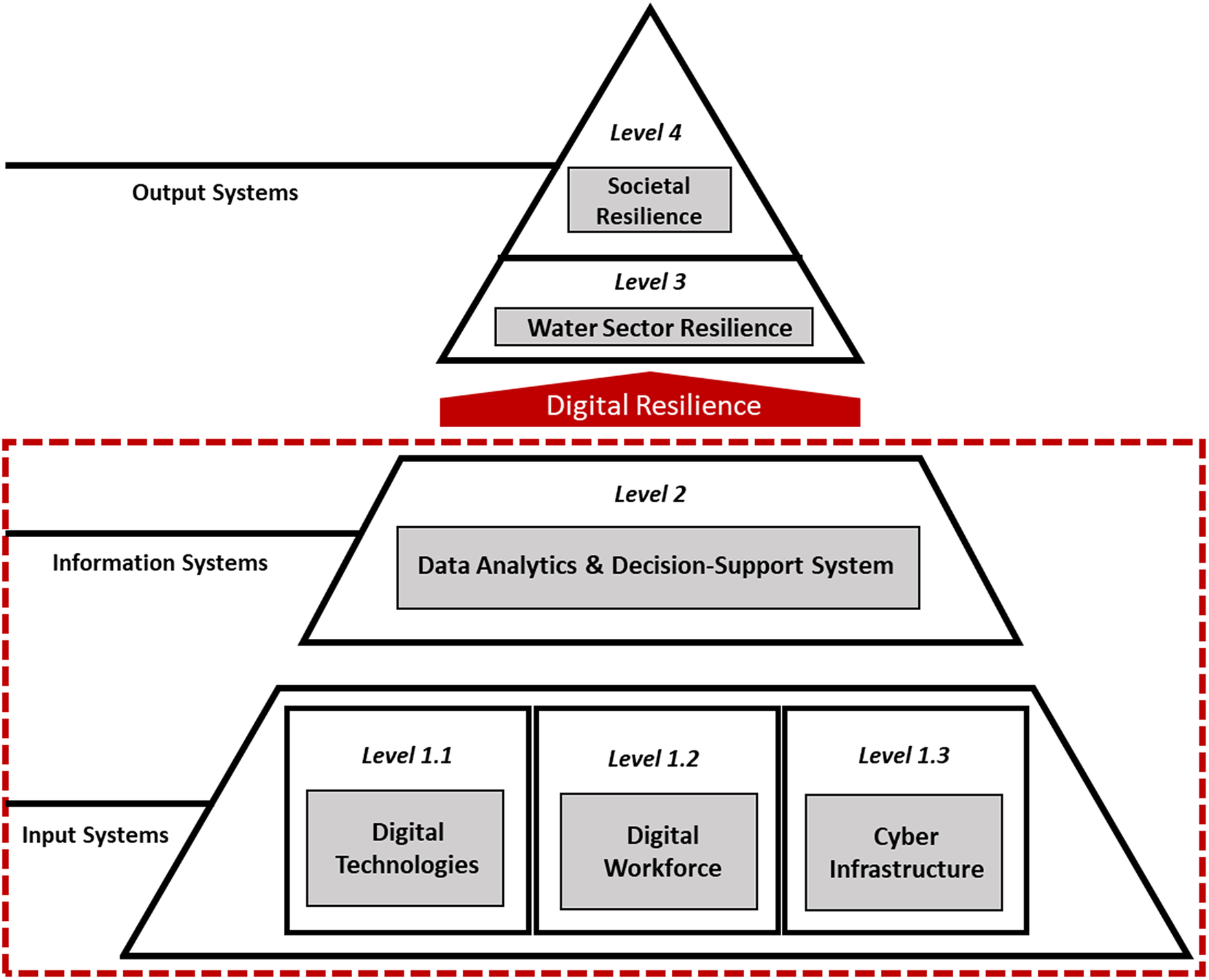
Figure 5. A digital resilience framework.
A holistic understanding of dependencies and interdependencies is required to improve the resilience of the water sector systems. An example of dependency is a water pumping station dependent upon electric power to operate. An example of interdependency is the electric power system being dependent upon water from the pumping station to generate the power needed by the pumping station. An interdependency is a more complicated concept than a dependency. Dependencies and interdependencies among natural, built, and social infrastructure systems play a crucial role in defining the instantaneous and long-term performance, resilience, and sustainability of infrastructure system services. In the literature, there are several descriptive classifications of dependencies and interdependencies (Rinaldi et al., Reference Rinaldi, Peerenboom and Kelly2001; Zimmerman, Reference Zimmerman2001; Dudenhoeffer et al., Reference Dudenhoeffer, Permann, Manic, Perrone, Wieland, Liu, Lawson, Nicol and Fujimoto2006; Halfawy et al., Reference Halfawy, Vanier and Froese2006; Gardoni and Murphy, Reference Gardoni and Murphy2008; Buldyrev et al., Reference Buldyrev, Parshani, Paul, Stanley and Havlin2010) based on different dimensions. Figure 6 shows an example of natural, built, and social systems interactions for water sector.

Figure 6. Different dimensions of infrastructure systems interdependencies.
To facilitate mathematical modeling, we could consider the classification of interdependencies developed by Sharma et al. (Reference Sharma, Nocera and Gardoni2020), as shown in Figure 7. This classification considers the epistemology dimension, which is needed to define the type and form of the interdependencies’ models. As the first step in the implementation of this general classification, we can identify the specific dependencies of water infrastructure systems (natural, engineered, and socio-economic water systems). In the second step, we can identify the interdependencies of other supporting infrastructure systems. For example, the water infrastructure has operational and performance interdependencies with power infrastructure due to the requirement of power for the operation of pumps and other treatment facilities. Also, water infrastructure has episodic recovery interdependencies with transportation infrastructure to support the movement of crews, equipment, and material. After classifying the dependencies and interdependencies, we can develop the respective mathematical models for the resilience management of integrated water infrastructure systems.
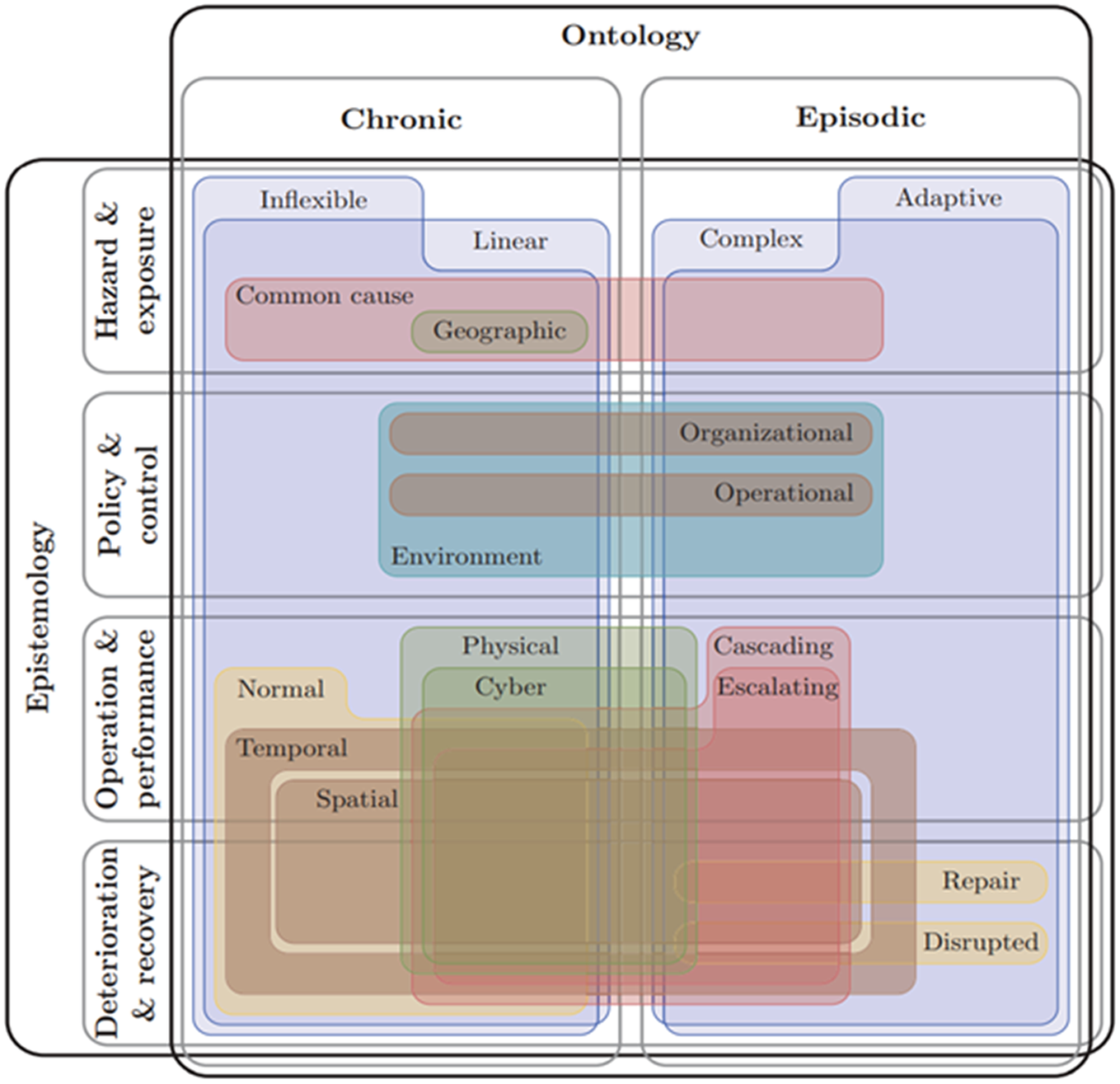
Figure 7. A classification of infrastructure systems interdependencies. Source: Carpenter et al. (Reference Carpenter, Walker, Anderies and Abel2001).
The nexus of sustainability and resilience
Resilience and sustainability are two separate terms and concepts that are often used interchangeably, sometimes without fully understanding what they mean. To reap the full benefit of combining resilience thinking with sustainable development, the nexus between the two concepts needs to be both understood and appreciated. Sustainability ensures that current and future generations are not compromised with respect to the environment, the economy, the society or human health (often informally referred to as people, profit, planet). Resilience refers to the ability to withstand and recover quickly from disruptions or shocks such as natural and/or manmade hazards and/or cyber-attacks. Some frameworks define sustainability as a goal and resilience as a feature of the goal (Roostaie et al., Reference Roostaie, Nawari and Kibert2019). Others define each as a goal in themselves (Boakye et al., Reference Boakye, Murphy, Gardoni and Gardoni2019; Faber, Reference Faber and Gardoni2019; Gardoni and Murphy, Reference Gardoni and Murphy2020; Trejo and Gardoni, Reference Trejo and Gardoni2023). Regardless of the framework, sustainability and resilience are complementary and interlinked concepts, since without resilience it is not possible to operationalize sustainability.
DWS water systems, similar to other critical infrastructure, are a part of the massive public infrastructure investments that are resource intensive and are critical for sustaining the well-being of our communities. However, similar to many other infrastructure systems, existing water infrastructure systems are aging and are overburdened, while new infrastructure is being established to meet new demands. The survivability or rapid restoration ability of these infrastructure systems is critical to the rapid recovery of our communities during and post-disaster. That recovery ensures that our economic life, social fabric, and public health systems are not only sustainable but are also resilient and ready to serve our communities through and post-disaster. The key is balancing of sustainability-driven and resilience-driven performance goals in water infrastructure systems, as graphically illustrated in Figure 8. The future of water management for overcoming global water challenges lies in successfully using technologies and policies that promote the global water cycle for both sustainability goals (i.e., environmental, social, and economic goals) and resilience goals (i.e., robustness, redundancy, rapidity, and resourcefulness).
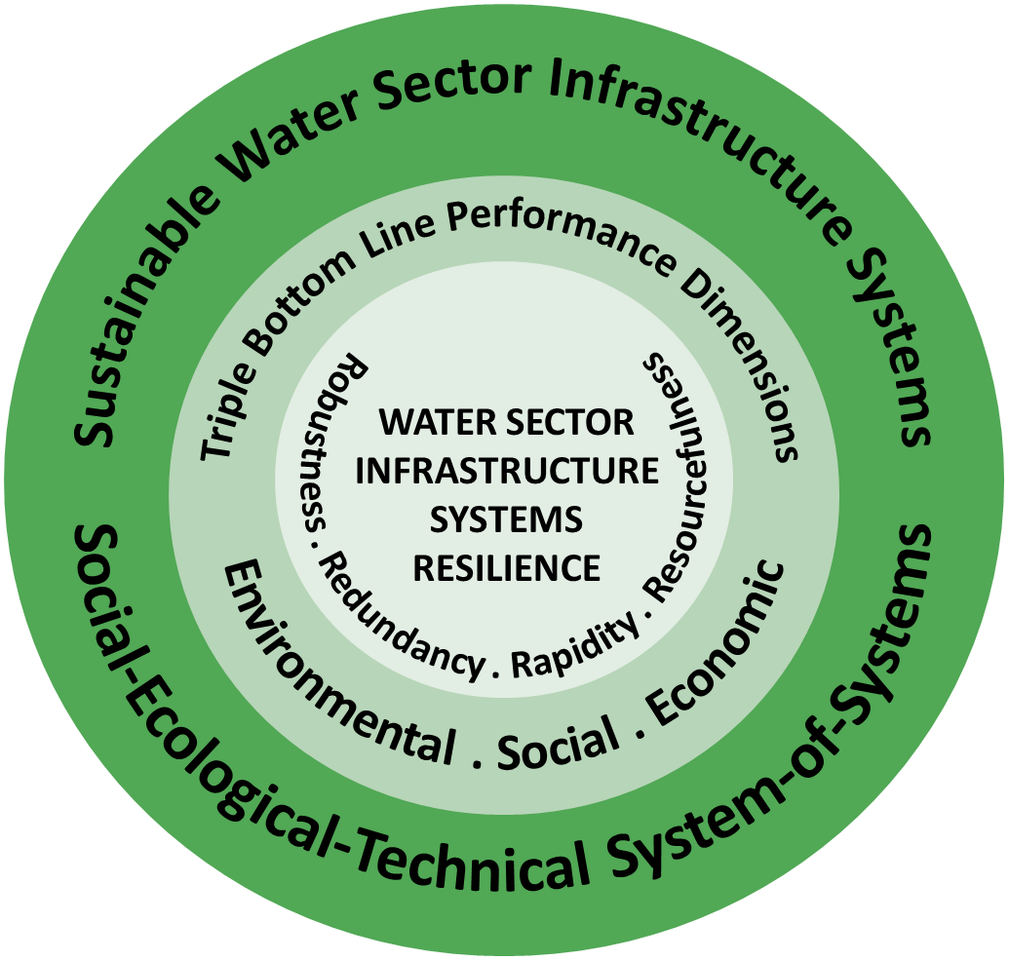
Figure 8. Graphical representation of the nexus of sustainability and resilience.
Water systems, similar to other critical infrastructure, are a part of the massive public infrastructure investments that are resource intensive and are critical for sustaining the well-being of our communities. However, similar to many other infrastructure systems, existing water infrastructure systems are aging and are overburdened, while new infrastructure is being established to meet new demands. The survivability or rapid restoration ability of these infrastructure systems is critical to the rapid recovery of our communities during and post-disaster. That recovery ensures that our economic life, social fabric, and public health systems are not only sustainable but are also resilient and ready to serve our communities through and post-disaster. The key is balance. The future of water management for overcoming global water challenges lies in successfully using technologies and policies that promote the global water cycle for both sustainability and resilience (Argyroudis et al., Reference Argyroudis, Mitoulis, Chatzi, Baker, Brilakis, Gkoumas, Vousdoukas, Hynes, Carluccio, Keou, Frangopol and Linkov2022). Integrating the management of water in all its forms – drinking, storm, waste, and the natural surface water and groundwater resources– is the only way to solve our current and future water challenges. Sustainable water infrastructure that is designed to minimize environmental impacts and resource use is also more likely to be able withstand and recover from hazards, both natural and human caused, because it is adaptable and flexible. Likewise, water infrastructure that is designed for resilience through redundancy, designed above code minimums, and leverages backup systems for robustness is also more likely to be sustainable over the long term because it can withstand or rapidly recover from natural and human-caused hazards. A lack of resilience also harms the sustainability triple bottom line, especially when particular vulnerable groups or systems excessively struggle to recover. For example, one may argue that when additional costs are incurred in trying to make DWS systems resilient to regional hazards, the cost of water services may become unaffordable for some customers. Such countereffects can be mitigated via interventions such as financial tools (e.g., governmental investments) that subsidize the initial investment on upgrading the DWS systems for resilience. In such scenario, when vulnerable communities with upgraded DWS systems are affected by disruptions then their recovery is not invariably more expensive and it takes less time to recover (thereby, also supporting sustainability goals).
Considering the number and magnitude of hazards that a community may face, disruption is inevitable – the ability to resist, absorb and become stronger is the differentiator. The ability to either repel impact in the first place or recover from impact in the short and medium term is of course central to building resilience but it is also crucial to developing long-term water sector sustainability. An integrated water management approach (Alanis and Sinha, Reference Alanis and Sinha2014) that seeks to disrupt the current siloes in the water sector is better prepared to advance the scientific understanding of and managing risks and challenges associated with short-term, medium-term, and long-term resilience in the water sector. Such an approach is also more effective at engaging with partners and stakeholders at all levels (e.g., citizens, agencies, environmentalists, social scientists, public officials, and industry) in order to develop innovative solutions for mitigating impacts from hazards, while also supporting a healthy ecosystem and economic growth. Figure 9 illustrates how the integrated water sector management under normal, stressed, and catastrophic events provides a comprehensive framework for guiding decisions and investments for both sustainable and resilient water services.

Figure 9. An integrated framework for water sector management and governance.
The costs and challenges associated with implementation
According to the findings compiled in the National Climate Assessment Report (USGCRP, Reference Reidmiller, Avery, Easterling, Kunkel, KLM, Maycock and Stewart2018), climate change is already causing more frequent and severe weather across the U.S. Extreme storms, heat waves, wildfires, and floods are becoming regular events rather than rare ones. The report’s authors clearly state that action must happen soon, and it must be significant. ‘The severity of future impacts will depend largely on actions taken to reduce these impacts and to adapt to the changes that will occur’, they write. If there is one clear lesson from the federal report, it is that we need to anticipate and prepare for extreme weather-related events, rather than simply react to them – the cost of critical infrastructure failure is too high. The study by the National Institute of Building Science (NIBS) (MHMC, 2019) found that mitigation saves up to $13 per $1 Invested. We need to invest in resilient and sustainable infrastructure.
Undoubtedly there is a cost to the utility of not investing in resilience. There are expenses associated with being prepared for a disaster and having the ability to rapidly respond and recover lost services. Based on the size of an event, there is a duration of potential lost services and the costs associated with that. Customers have a tolerance level for how long water and wastewater services outages can last. The tolerances are different for each customer, for example, hospitals cannot tolerate service outages for very long without extreme consequences to the community, while a sports complex may tolerate long service outage durations. For different events, the costs of response, recovery, and community services outages (including the community losses) may be acceptable. In many cases, however, there may be justification to make resilience improvements to the system to reduce the possibility of service losses during a disaster and/or the service loss duration.
A key challenge facing utilities is finding the right balance between the need to invest in chronic issues such as aging infrastructure with the need to invest in resilience enhancement to guard against acute threats. In addressing aging infrastructure investment, utility managers have increasingly adopted good practice asset management approaches that help to identify the best balance of performance, cost and risk. Typically, these approaches consider risk reduction per $ USD spent and/or lifecycle net present value (NPV) cost of ownership of different investments to justify and prioritize spending. As utilities consider how best to deal with their resilience issues, they will need to identify the type and level of mitigations and how these are combined with other investments to develop an optimized Capital Improvement Program (CIP). Figure 10 summarizes some of the key considerations and the interaction between risk-based asset management, resilience programs and the assessment of financial impact of the overall investment budget. Typically, these resilience mitigation investments, have a wide range of costs associated with them, from relatively inexpensive planning studies, through operational solutions to significant capital investments.

Figure 10. Critical resilience decisions illustrating types of risk mitigation strategies.
Investments in improving asset resilience can be considered in four broad types, shown in Figure 11. Investments in improving asset Resistance are focused on hardening against damage or disruption, while investments in Response and Recovery ensure that levels of service are restored as quickly as possible after an event. Both these types of investment (shown in dark blue below) need to be integrated into the CIP under purely resilience-related drivers. These investments need to demonstrate a tangible cost–benefit. They need to be scored in a way that allows the systematic comparison and prioritization against investments of other drivers, like aging infrastructure, growth or regulatory.

Figure 11. Types of infrastructure resilience.
Reliability investments are those that ensure that assets can operate under a wide range of conditions and are more robust, while Redundancy investments provide back up capacity. These investment types can be considered incremental investments that improve resilience by augmenting or increasing the capacity of existing assets. As part of asset lifecycle planning, assets are replaced with more reliable, redundant infrastructure to build resilient networks over time. When considered over the lifecycle of the assets, such investments may provide considerable benefit for relatively small incremental costs. Resilience does not always need to be expensive! In fact, when incorporating resilience thinking, there are activities which can improve infrastructure resilience at no cost or even with a cost savings. The plans often need to be system-level to show how each project works for incremental improvement, as resilience is not easy to achieve by separately looking only at one project at a time. The plans should clearly identify the cost-effectiveness of these resilience improvements.
Resilience has traditionally not been a central component of utility capital improvement plans, but rather a parallel, somewhat peripheral activity. With the increased challenges faced by Utilities in providing resilient service, investments in resilience are more important than ever and need to be brought into the mainstream of Utility planning and asset lifecycle management. The most important aspect is to incorporate resilience thinking into the organization to systematically identify what needs to be done. If this is achieved, plans can be made that allow for continuous improvements that target an objective resilience state at some time in the future. Clearly, resilience investments need to be evaluated in a systematic way and compared with investments of different types to ensure a balanced capital improvement plans that is fiscally prudent and delivers levels of service at an optimal cost/risk balance. Integrating resilience into master planning and CIP development needs to be more widely adopted to ensure that an investment backlog does not build which puts resilient infrastructure out of the reach of utilities.
Physical and digital resilience literature review
The literature review was conducted in two stages: First, a literature search on resilience-related material in bibliographic databases identified five key domains (Physical, Ecological, Societal, Economics, and Engineering) as the key fields for understanding resilience in infrastructure systems (Alanis, Reference Alanis2013; Sinha and Alanis, Reference Sinha and Alanis2014). Digital resilience arose as a separate cross-cutting domain separately, and was mainly related to cyber-security in digitalized infrastructure. The second stage of the literature review explored the concept of resilience in networked infrastructure systems like DWS that operate within the dynamics of natural, social, and built environments (Pearce and Vanegas, Reference Pearce and Vanegas2002; Pedicini et al., Reference Pedicini, Stolte, Sinha and Smith2014). The societal environment drives DWS infrastructure to form and function through demand. The natural environment imposes constraints on resources available for satisfying those demands. Hence, the resilience of DWS systems should consider influences and interactions across all these networked systems to address comprehensively the specific challenges (Biggs et al., Reference Biggs, Gordon, Raudsepp-Hearne, Schlüter, Walker, Biggs and Schlüter2015; Preiser et al., Reference Preiser, Biggs, De Vos and Folke2018; Glazer et al., Reference Glazer, Tremaine, Banner, Cook, Mace, Nielsen-Gammon, Grubert, Kramer, Stoner, Wyatt, Mayer, Beach, Correll and Webber2021; Farhad and Baird, Reference Farhad and Baird2022; Reed et al., Reference Reed, Hadjimichael, Moss, Brelsford, Burleyson, Cohen, Dyreson, Gold, Gupta, Keller, Konar, Monier, Morris, Srikrishnan, Voisin and Yoon2022). The NIAC report (NIAC, 2016) on water sector resilience has highlighted the importance of interdependencies, the need to address emerging risks, and the significant challenge of the funding needed for improvements to systems. Resiliency was first explained for ecological systems (Holling, Reference Holling1973). Since then, many definitions have been proposed outside of the water sector (Walker et al., Reference Walker, Holling, Carpenter and Kinzig2004; White House, 2007; ANSI/AWWA, 2010; Alanis and Sinha, Reference Alanis and Sinha2013; Ayyub, Reference Ayyub2014; AWIA, 2018) and specific to the water and wastewater sector (Butler et al., Reference Butler, Farmani, Fu, Ward, Diao and Astaraie-Imani2014; Matthews, Reference Matthews2016; Shin et al., Reference Shin, Lee, Judi, Parvania, Goharian, McPherson and Burian2018; Ebrahimi et al., Reference Ebrahimi, Mortaheb, Hassani and Taghizadeh-yazdi2022). Most definitions hold three characteristics of resilience: (1) the amount of change a system can undergo, or the amount of stress it can sustain and still retain the same controls on functions and structure; (2) the degree to which the system is capable of self-organization; and (3) the degree to which the system expresses capacity for learning, adaptation, and recovery. Other work specific to the water sector has been around major challenges (Goldbloom-Helzner et al., Reference Goldbloom-Helzner, Opie, Pickard and Mikko2015; Juan-Garcia et al., Reference Juan-Garcia, Butler, Comas, Darch, Sweetapple, Thornton and Corominas2017; Kuisma et al., Reference Kuisma, Nickum, Bjornlund and Stephan2020; Lawson et al., Reference Lawson, Farmani, Woodley and Butler2020; Pamidimukkala et al., Reference Pamidimukkala, Kermanshachi, Adepu and Safapour2021), frameworks and modeling techniques for measuring resilience and resilience in the context of numerous disasters and multiple concurrent crises (Balaei et al., Reference Balaei, Wilkinson, Potangaroa and McFarlane2020; Knodt et al., Reference Knodt, Fraune and Engel2022; Saikia et al., Reference Saikia, Beane, Garriga, Avello, Ellis, Fisher, Leten, Ruiz-Apilánez, Shouler, Ward and and Jiménez2022).
The five domains of resilience presented in the context of physical and digital resilience are presented below.
Physical resilience
Infrastructure assets need to maintain physical integrity to function above the expected level of service. The physical resiliency of a water system can be described as the ability of different assets like pipelines, treatment plants, and so forth to reduce the magnitude and/or duration of disruptive events and to provide uninterrupted or to restore rapidly levels of services to acceptable levels. The resilience of physical systems’ can be measured based on attributes like asset condition, network design, deterioration rate, and time to recovery as metrics that represent the ability to absorb, adapt and recover.
DWS infrastructure systems are built interfaces between the natural environment and societal needs, and therefore are affected by dynamic ecologic processes and also feed impacts back on the natural environment. Ecological resilience of water and wastewater infrastructure systems considers the ability of the natural system to move to an equilibrium state, after being affected by disruptions like contamination due to sewers overflows, flooding due to water main failures, water extraction and other major shocks and stresses due to natural-built system interactions. Ecological resilience acknowledges the fact that ecosystems frequently do not return to the original state after disruption, but instead reach a new equilibrium, as occurs in other fields such as economics. The ecology approach to resilience inspired developments in the engineering resilience field, significantly with the paper ‘Resilience and stability of ecological systems’ (Holling, Reference Holling1973). Ecological resilience includes complex models with regime shifts, thresholds, and multiple equilibriums (Abel et al., Reference Abel, Cumming and Anderies2006; Davidson et al., Reference Davidson, Jacobson, Lyth, Dedekorkut-Howes, Baldwin, Ellison, Holbrook, Howes, Serrao-Neumann, Singh-Peterson and Smith2016; Krievins et al., Reference Krievins, Plummer and Baird2018; Kang et al., Reference Kang, Bowman, Hannibal, Woodruff and Portney2023; Palilionis, Reference Palilionis2023). Ecosystem resilience is related to sustainable development, and therefore a resilient built environment (including infrastructure systems) incorporates ecological considerations.
Social resilience includes among others, the role of agents such as organizations and businesses (West and Lenze, Reference West and Lenze1994; Quarantelli, Reference Quarantelli1999; Prud’homme, Reference Prud’homme2008; Sharifi, Reference Sharifi2016), community response to disasters (Mileti, Reference Mileti1999; Anon, 2006), emergency management (Saja et al., Reference Saja, Goonetilleke, Teo and Ziyath2019), societal impacts from infrastructure failure (Collins et al., Reference Collins, Carlson and Petit2011; Meerow et al., Reference Meerow, Pajouhesh and Miller2019), and community effects of infrastructure interdependencies (Davis and Giovinazzi, Reference Davis and Giovinazzi2015; Rose, Reference Rose2016; NAE, 2017). Social resilience has a variety of methods and perspectives (Allenby and Fink, Reference Allenby and Fink2005; Berkes, Reference Berkes2007; Coaffee, Reference Coaffee2008; Keck and Sakdapolrak, Reference Keck and Sakdapolrak2013) that can help improve social resiliency during external shocks that affect water and wastewater infrastructure systems. Social resiliency for water and wastewater infrastructure systems considers the ability of communities served by utilities to tolerate, absorb, cope with, and adjust to three major types of shocks and stresses – (1) disruption of water and wastewater services due to external shocks like tropical storms, floods, earthquakes, and so forth. (2) long term stresses due to water unavailability, declining water quality and frequent disruptions due to poor infrastructure and (3) changing societal habits and urbanization resulting in regional economic transformation and uncertainties.
The economic resilience approach to water and wastewater infrastructure resilience is based on evaluating the financial implications of system preparedness, failure, and recovery such as revenue loss, restoration, and recovery cost; and economic impact on community activities (Qiao et al., Reference Qiao, Jeong, Lawley, Richard, Abraham and Yih2007; Weick and Sutcliffe, Reference Weick and Sutcliffe2007; Jain and McLean, Reference Jain and McLean2009; Vugrin et al., Reference Vugrin, Warren, Ehlen and Camphouse2010; Morris-Iveson and Day, Reference Morris-Iveson and Day2021). Some economic resilience definitions include only post-disruption recovery. Economic resilience is classified into two main types, inherent and adaptive (Rose, Reference Rose2004). Engineering resilience specialties have developed resilience concepts, such as safety, and reliability, among others.
Some resilience developments significant for civil infrastructure systems are generated from structural engineering. The current structural engineering approach to infrastructure resilience is significantly influenced by earthquake engineering (Tabucchi et al., Reference Tabucchi, Davidson and Brink2010). Many papers in structural engineering resilience come from the Multidisciplinary Center for Extreme Event Research (MCEER) ‘R4’ framework and therefore share similar methodologies (Alanis and Sinha, Reference Alanis and Sinha2012a,Reference Alanis and Sinhab). The R4 framework considers resilient systems as having four attributes: robustness, redundancy, resourcefulness, and rapidity (Bruneau et al., Reference Bruneau, Chang, Eguchi, Lee, O’Rourke, Reinhorn, Shinozuka, Tierney, Wallace and Von Winterfeldt2003; Bruneau and Reinhorn, Reference Bruneau and Reinhorn2004; Cimellaro et al., Reference Cimellaro, Reinhorn and Bruneau2006; Reference Cimellaro, Reinhorn and Bruneau2007). Engineering resiliency similarly can be defined as the performance of these systems in the R4 framework, by controlling the amount of service losses when subjected to the shocks and stressors and rapidly returning the basic levels of service to customers after extreme events.
Digital resilience
The increasing use of digital tools like sensory technologies, computational modeling, data repositories, and centralized control systems has resulted in increased connectivity of discrete information system assets and a proliferation of cyber access points, escalating water and wastewater infrastructure system complexity (Adedeji and Hamam, Reference Adedeji and Hamam2020). These systems can be considered ‘Cyber-Physical-Social’ systems owing to the increasing integration of digital and physical assets for achieving societal benefits. The use of digital technologies has enabled utilities to develop decision support systems with improved real-time data and advanced analytics promoting greater reliability, efficiency, and productivity. However, the reliance on digital tools can bring vulnerabilities from the digital space to physical assets, thereby threatening the confidentiality, integrity, and availability of infrastructure services. Previous studies have recommended the use of panoply approaches with multiple layers of protection for data communication services, modeling, and decision support platforms to increase redundancy in digital assets (Mohebbi et al., Reference Mohebbi, Zhang, Christian Wells, Zhao, Nguyen, Li, Abdel-Mottaleb, Uddin, Lu, Wakhungu, Wu, Zhang, Tuladhar and Ou2020). This study also recommended the use of network-based metrics based on techniques like graph theory to identify the strategic locations for redundancy implementation. Recent studies have also investigated the challenges and opportunities for achieving a resilient water sector workforce (Germano, Reference Germano2018), specially since the outbreak of the COVID-19 pandemic (Cotterill et al., Reference Cotterill, Bunney, Lawson, Chisholm, Farmani and Melville-Shreeve2020).
Most of the literature in digital resilience investigates the extents of threat and risk mitigation measures for cyberattacks (Sarkar et al., Reference Sarkar, Wingreen, Ascroft and Sharma2021; Kohn, Reference Kohn2023). These studies claim that although cyber attacks can occur from any person anywhere in the world, there are certain threat vectors like weak authentication, network scanning, spear phishing and abuse of access authority, among others that critical infrastructure asset owners face regularly and can be targeted for security improvements (Heeks and Ospina, Reference Heeks and Ospina2018).
Most definitions of digital resilience in the water sector have focused on cybersecurity, especially from threats like cyber-attacks (Mohebbi et al., Reference Mohebbi, Zhang, Christian Wells, Zhao, Nguyen, Li, Abdel-Mottaleb, Uddin, Lu, Wakhungu, Wu, Zhang, Tuladhar and Ou2020). Studies outside of the water sector have proposed different definitions for digital resilience with similar keywords like information systems resilience (Sarkar et al., Reference Sarkar, Wingreen, Ascroft and Sharma2021), digital security resilience (Kohn, Reference Kohn2023) and e-resilience (Heeks and Ospina, Reference Heeks and Ospina2018). We define digital resilience as the ability of the deployed information systems to detect shocks, deter malevolent actors, prevent attacks, absorb major stresses, evolve to address new failure mechanisms, respond by alerting decision-makers, and recover to at least the minimum level of service.
Current frameworks and standards for digital resilience in the water and wastewater sector have recommended risk-based methodologies from the perspective of cyber security (USDHS, 2015; NIST, 2018). In general, the literature defines cybersecurity frameworks for critical infrastructure in two frameworks; mission assurance engineering and cyber resiliency engineering (Mohebbi et al., Reference Mohebbi, Zhang, Christian Wells, Zhao, Nguyen, Li, Abdel-Mottaleb, Uddin, Lu, Wakhungu, Wu, Zhang, Tuladhar and Ou2020). Mission assurance is an emerging discipline that aims to apply systems engineering, risk management, and quality assurance to achieve successful delivery of service to customers. Cyber resiliency engineering seeks to elevate mission assurance by bringing the ever-evolving set of resilience practices into real implementations of cyber infrastructure (Mohebbi et al., Reference Mohebbi, Zhang, Christian Wells, Zhao, Nguyen, Li, Abdel-Mottaleb, Uddin, Lu, Wakhungu, Wu, Zhang, Tuladhar and Ou2020). This definition of cyber resiliency corresponds well with our definition of digital resilience as cyber resilience differs from traditional cyber security. Studies have recommended this broader digital resiliency concept and have developed frameworks with a categorized set of digital resiliency metrics to address the problem comprehensively (Bodeau et al., Reference Bodeau, Graubart, Heinbockel and Laderman2015).
Digital and physical assets should have built-in multiple protection layers (Björck et al., Reference Björck, Henkel, Stirna and Zdravkovic2015) and support the monitoring and analysis of all components. The systems should also utilize techniques such as dynamic positioning (ability to relocate system assets), diversity (using a heterogeneous set of technologies), nonpersistent design (time-limited retention policy), privilege restriction (fine-grained access control), and segmentation (logical and physical separation of components) (Bodeau and Graubart, Reference Bodeau and Graubart2011). Operation and management strategies including periodic education and training programs customized for operations staff, utility managers, and IT staff. It should also be noted that both over and under-regulation can lead to diminished system resilience based on human factors such as an overabundance of information, raised stress levels, and decreased time to perform critical functions (Gisladottir et al., Reference Gisladottir, Ganin, Keisler, Kepner and Linkov2017). Additionally, utilities should also consider their digital resilience and a variety of factors before purchasing the appropriate cyber insurance. These factors include the role of security in the resiliency plans, procedures for the measurement of risks, understanding the impacts of cyber-attacks on critical cyber-physical infrastructures, and processes for the organization to address known digital threats (Tonn et al., Reference Tonn, Kesan, Zhang and Czajkowski2019).
Coastal, seismic, and digital resilience practice review
Each water sector owner and operator manages a unique set of assets and operates under a distinct risk profile. As such, each utility’s risk-management priorities depend on many factors, including utility size, location, assets, distinct risks, governance, availability of adequate funding, and perhaps most importantly, the resources, staff training, and capabilities the utility can access. While each utility is responsible for its own risk management, sector-wide collaboration, national, state, regional, and local initiatives play a major role in boosting the resilience of the water sector as a whole. The current practice of utilities for managing resilience to coastal, seismic, and digital hazards is reviewed in more detail in the following subsections. We propose that similar lessons can be applied to also address numerous other hazards that are not discussed specifically in this section but are relevant to the risk profiles of many water sector utilities.
Coastal resilience
In all countries with access to oceans and bays, the coastal settlement has been the historic location of choice for reasons of commerce, mobility, and the opportunities provided by the waterfront. The United States, with Atlantic, Pacific, Gulf, and Arctic coastlines, has a population distribution concentrated in these coastal areas. Sea level rise, one of the distinctive and well-documented outcomes of warming global air and water temperatures, represents a serious challenge to the built infrastructure of coastal developments, including water sector infrastructure systems as shown in Figure 12 (Azevedo de Almeida and Mostafavi, Reference Azevedo de Almeida and Mostafavi2016; Hummel et al., Reference Hummel, Berry and Stacey2018).

Figure 12. National sea-level rise map for the United States. Source: Sealevelrise.org.
System-of-systems approach for sea-level rise
Utilities like the New York City Department of Environmental Protection (NYCDEP) uses a system-of-systems approach to include and manage the water and energy systems. The systems are comanaged to reduce their carbon footprints using specialized sustainability teams for proactively tracking and identifying opportunities that offset GHG emissions and/or optimize indirect energy cobenefits. (Balci and Cohn, Reference Balci and Cohn2014). Additionally, the NYC Wastewater Resiliency Plan studies different systems like the wastewater infrastructure of buildings (including 96 pumping stations and 14 wastewater resource recovery facilities) to identify and prioritize infrastructure that is most at risk of flood damage. Through the study, NYCDEP has developed a set of recommended design standards and cost-effective protective measures tailored to each facility to improve resiliency in the face of future flood events. The East Side Coastal Resiliency project from NYCDEP addresses threats due to sea level rise by reducing flood risk to property, landscapes, businesses, and critical infrastructure while also improving waterfront open spaces and access. An integrated flood protection system across a 2.4-mile span considers different systems like waterfront open spaces, urban streets, residences, businesses, schools and other vital infrastructure, including a pump station and electrical substation. Similarly, the Thames Estuary Asset Management 2,100 (TEAM 2100) Programme in the United Kingdom is a 10-year capital investment programme to refurbish and improve existing tidal flood defenses (UK Government, 2012). This plan focuses on utilizing systems-of-systems approach to protect the social, cultural and commercial value of the tidal Thames, tributaries and floodplain. Other examples include the Sydney Coastal Councils Group that brings together 20 councils within the Greater Sydney Harbor catchment to collaborate with state agencies to develop a whole-of-system Coastal Management Program for Greater Sydney Harbor (Harbour Reference Harbour2015).
Whole-life approach for management of risks
There is a lack of studies investigating the lifecycle management of risks to the water security in the context of coastal resilience. In the current practice, risks associated with sea level rise include: enhanced storm surge beyond historic experience and planning parameters (Jevrejeva et al., Reference Jevrejeva, Williams, Vousdoukas and Jackson2023), decreased efficiency of drainage systems (Boogaard et al., Reference Boogaard, Rooze and Stuurman2023) and increased incidence of inundation from both storm surge-related flooding and rain event flooding (Paulik et al., Reference Paulik, Wild, Stephens, Welsh and Wadhwa2023); the elevation of local ground water tables that may be hydraulically connected to coastal waters (Befus et al., Reference Befus, Barnard, Hoover, Finzi Hart and Voss2020), possibly causing salt water intrusion and greater infiltration and inflow into gravity sewer lines and other underground infrastructure, and increased deterioration of underground infrastructure; and interruption of surface transportation modes needed to support utility operations when flooding occurs. A true understanding of a risk profile also involves the dynamic coupling of groundwater, surface water, and climatic models so that the capital infrastructure planning can be developed more comprehensively.
Consequence
The consequences of these risks can be infrastructure failures, more rapid deterioration of equipment subject to chronic salt water exposure (Sangsefidi et al., Reference Sangsefidi, Bagheri, Davani and Merrifield2023) and the wet-dry cycle with changing groundwater and tide, and the potential for stranded or oversized assets if utility service areas experience chronic flooding to the point that residents and businesses no longer find the area viable for either business or living. The continued availability of affordable insurance can be another factor relevant to the need for and viability of utility infrastructure to serve coastal areas (Rasmussen et al., Reference Rasmussen, Kopp and Oppenheimer2023).
Planning
Planning for sea level rise requires some ability to forecast a probable set of future sea level rise scenarios for the area (Jevrejeva et al., Reference Jevrejeva, Williams, Vousdoukas and Jackson2023). Sea level rise, perhaps surprisingly, does not occur uniformly as it would if water was added to a bathtub. Currents and wind play a definite role, and whether a land mass is subsiding (as along much of the Gulf coast) or rebounding from the pressure of glaciers (as in Alaska and other northern locations) will make a difference (Seitz et al., Reference Seitz, Kenney, Patterson-Boyarski, Curtis, Vélez, Glodzik, Escobar and Brenner2023). Tools needed for this type of analysis include storm surge models that can characterize wave height and surge-related flooding under a variety of tidal, wind, and sea level rise scenarios (Elahi et al., Reference Elahi, Wang, Salcedo-Castro and Ritchie2023; Makris et al., Reference Makris, Tolika, Baltikas, Velikou and Krestenitis2023; Mathew and CA, Reference Mathew and CA2023). In the past sea level has been regarded as a fixed and static condition that varies with tide and wind within some set of confined extremes. Changes due to the expansion caused by ocean warming and the melting glaciers are transferring enormous water volumes from the land to the oceans. Sea level must be considered a dynamic rather than a static factor for planning purposes (Brammer, Reference Brammer2014). It is therefore necessary to forecast sea level rise changes over time to estimate the vulnerability of assets over that time and to formulate risk mitigation measures. Miami-Dade County has undertaken such analyses due to its extraordinary vulnerability to sea level rise (Tompkins and Deconcini, Reference Tompkins and Deconcini2014; Sukop et al., Reference Sukop, Rogers, Guannel, Infanti and Hagemann2018). Southeast Florida, and much of the Gulf and Atlantic coastal areas, are low-lying areas that rely on effective drainage systems to make upland development viable. In many instances, the development of barrier islands has attracted tourists and residents to tropical beaches (Major et al., Reference Major, Major-Ex, Fitton and Lehmann2021). The regional County water and sewer utility has undertaken detailed storm surge modeling using regionally adopted forecasts to evaluate the potential consequences of sea level rise over the remainder of this century, with emphasis on risks to its three coastal sewer plants. With an assumption of about 3 ft of sea level rise by 2075, the storm surge models predict that facilities should be built to about 20 ft of elevation to avoid worst-case storm surge flooding resulting from an extremely high tide and a severe hurricane. Most of the existing infrastructure is at 10–15 ft of elevation.
Design
The design guidelines have been developed for the ongoing reconstruction of much of the sewer infrastructure as required through a federal consent decree. The elevation of facilities where practical will be combined with hardening and waterproofing to mitigate future risks in a cost-effective manner over the projected life of the specific assets being replaced or being added to the system. Of greatest importance during and after an extreme weather event is the ability to maintain flow through the sewer plants, even if treatment is not complete. This requires the availability of power and pumps, electrical switchgear, and control systems. These components tend to be the most vulnerable to flooding conditions. The entire Miami-Dade water and sewer system already can (and does as needed) run off the grid, so long as the generators are not impacted by storm surge or flooding.
Preliminary analysis suggests that the marginal cost of improving resiliency as the system is renewed is in the range of 5% of the capital costs of doing the work. This is a good and necessary investment to make on a progressive basis, being careful to tie the additional investment to the expected life of the facility being replaced. By the time that facility is again in need of replacement, sea level rise forecasts will likely be more certain and technological advances may be available to mitigate risks more cost-effectively. However, considering that Miami-Dade County has three wastewater treatment plants along the coast line and which could be potentially impacted in the future due to the threats of hurricanes and sea level rise, any future planning effort must take into account not only the relocation of these plants inland but also the reversal of wastewater flows in the opposite direction through a system of over 1,000 pump stations.
Sea level rise can also impact the water supply side of utility operations. In South Florida, the very shallow surficial aquifer, the Biscayne Aquifer, is hydraulically connected to the ocean to a depth of about 200 ft at the interface. This means that ground water levels will rise as the sea level rises, impacting the regional drainage system that operates largely on gravity, and salt water intrusion will occur into the region’s primary water supply.
Modeling
The extensive modeling of the surface water and ground water has been done in conjunction with the U.S. Geological Survey to understand better both drainage consequences and the likely timing of salt water intrusion that could impact water supply wells and water treatment processes. Other areas of the country may rely on surface waters (rivers) that will be similarly impacted, possibly requiring relocation of withdrawal facilities to avoid increased salinity of the supply, or adding treatment to reduce salt content. For southeast Florida, there will be plenty of water in the future, but it may require expensive treatment for drinking water purposes. The more serious issue is whether the regional stormwater system constructed by the Army Corps of Engineers can be retrofitted to keep developed areas dry as the sea level rises and drainage is thereby limited. Considering the unique topography of the region and the existing governance system for managing stormwater in the region (currently managed and implemented by 33 cities and unincorporated Miami-Dade County) a true collaborative governance would be required to ensure resilience for Miami-Dade County. This necessitates an Integrated One Water approach to planning for the watershed.
Utilities cannot operate in a vacuum. They are essential for both public health and a viable economy, but they are also subject to the consequences of other public and private actions and investments. For that reason, the risk assessment and risk mitigation work that utilities need to incorporate as a dynamic component of their planning process should be integrated with these other sectors and stakeholders. This will ensure that common planning assumptions are used for the extent and timing of sea level rise, and that appropriate and consistent actions are being taken for other infrastructure, such as roads, drainage, and building code requirements, to maintain a coordinated approach to creating a realistic assessment of the community’s future. This is not an effort that can be done by a utility alone, but utilities need to be at the table. An example in this direction would be with population growth. Local governments are aggressively implementing infrastructure efforts to modernize transportation and provide more affordable housing, schools and hospitals. Each of these infrastructure elements requires the services of water, wastewater and stormwater facilities. While utilities would need to incorporate the relocation of assets which are going to be vulnerable to storm surge and flooding due to extreme precipitation events or sea level rise, their efforts need to be integrated with the infrastructure planning efforts of other sectors proactively. Utility assets for water and sewer infrastructure are predominantly underground and hence necessitate planning for future relocation of these assets. A concept called ‘Coastal Islands’ for emergency response is also important for many coastal communities because during disaster events they may be completely cut off by landslides, erosion, flooding, and collapsed bridges.
Mitigation
Overall, sea level rise is agnostic of the types of assets or systems it affects. A social–ecological–technical system-of-systems approach to resilience is essential due to the inherent interconnection between utilities and the scale of the effects of sea-level rise. Planners need a new cross-jurisdictional paradigm that considers the system-of-systems and equitable approach to plan mitigation strategies.
Seismic resilience
Thought by many to be mostly a West Coast phenomenon, US national seismic hazard maps indicate that catastrophic seismic events can occur across the country (Figure 13) with notable hazards in the Midwest near St. Louis, MO, and on the east coast near Charleston, SC. Much of the west coast is vulnerable to intense earthquake ground motions, whether it is the Cascadia subduction zone (CSZ), the San Andreas fault, or one of the many more local crustal faults, and even inland to Utah in the Basin and Range province. Recent studies show a 16–22% probability of an earthquake of a Magnitude 8.5 or greater on the CSZ in the next 50 years (Goldfinger et al., Reference Goldfinger, Wong, Kulkarni and Beeson2016). This would impact the entire Pacific Northwest and much of Northern California. Water and wastewater facilities are vulnerable to seismic events. Many water distribution facilities including tanks, pump stations, wells, and pipelines are quite old and were not designed with earthquakes in mind. For the large part, earthquakes are unpredictable and immediate, allowing for little response time. Most susceptible to earthquakes are areas where permanent ground deformations can occur like in liquefiable soils, landslides, and fault surface rupture. Many of these areas are not adequately mapped. Some, jurisdictions may not be aware of the extent of the vulnerabilities of their facilities. The lack of as-built drawings for critical facilities may be an issue. Determining the resilience of a facility is difficult if you cannot determine how it was designed and constructed, or modified later. There are many issues to be addressed to ensure water sector resilience to earthquakes. The following subsections identify some of the issues and important questions to be answered to ensure a resilient water sector.

Figure 13. National earthquake map for the United States. Source: USGS.
System-of-systems approach for earthquakes
Previous studies have investigated the interdependencies between the power and water systems in the wake of an earthquake to map the multiple earthquake scenarios representing the Los Angeles area seismic hazard. The analyses consider the fragility characteristics of system components with and without seismic retrofits and other system analyses into a performance criteria (Shinozuka and Dong, Reference Shinozuka and Dong2002; Shinozuka et al., Reference Shinozuka, Chang, Cheng, Feng, O’Rourke, Saadeghvaziri, Dong, Jin, Wang and Shi2004). This performance criterion was developed to map quantitatively the response space, in terms of the technological, economic, organizational, and social dimensions of the earthquake disaster resilience. This study also recommended the integration of water and power performance by concentrating on the pump stations vulnerable to the interruption of power supply. Future work on this area can focus on a more comprehensive integration of these systems with other critical systems, such as emergency response organizations, medical care systems (e.g., acute care hospitals) and highway transportation systems from the viewpoint of community resilience. Similar efforts are underway within many utilities around the world like the Water Seismic Study from the Portland Water Bureau’s as part of the Oregon Resilience Plan (ORP) (Saling and Stuhr, Reference Saling and Stuhr2017); performance and management of the gas, electric, water, wastewater and road networks in Christchurch, New Zealand to rapidly reinstate the functionality of critical infrastructure systems (Giovinazzi et al., Reference Giovinazzi, Wilson, Davis, Bristow, Gallagher, Schofield, Villemure, Eidinger and Tang2011); Tokyo Metropolitan Reslience Project (Furuya et al., Reference Furuya, Hirata and Tamura2019) and the San Francisco Public Utilities Commission’s (SFPUC) $4.6B Water System Improvement Program (WSIP) (Ortiz et al., Reference Ortiz, Wong, McVicker, Santos and Hatton2012); among others.
Whole-life approach for management of risks
There is a lack of studies investigating the lifecycle management of risks to the water infrastructure in the context of seismic resilience. In current practice, many utilities do not even have the main infrastructure components identified or knowledge on which facilities should be prioritized for restoration.
Know your system
Understanding your system and its vulnerabilities is key to protecting your infrastructure; Do you know which facilities are on bridges or trestles owned and/or maintained by others? Do you know the condition and design standards of these; Do you know what valves to close when the earthquake occurs? Time will be of the essence and a plan for locking down the system will be critical; how long would it take to restore water or sewer service to your system? Have you modeled your system with outage scenarios? Will you be able to adequately communicate with your political body?
Supplies and resources
An adequate inventory of items inside a pump station or other building may not be available. Is the equipment adequately braced? Do you know what resources might be needed for repair work? Do you have adequate materials in stock? Electrical supply and fuel are an issue during a seismic event. Do you have generators? Do you have the fuel available? Coordination with the energy and transportation sectors is crucial.
Resilience planning strategies
Understanding emergency backup options when the water supply is not available is vital. Redundancy within your system and agreements with local partners are both keys. Are there sufficient agreements in place? Is there a Water/Wastewater Agency Response Network (WARN) (Whitler and Stormont, Reference Whitler and Stormont2011) in your state and are you a member? Water operators should have discussions with their fire departments. This is key so that expectations can be set and water provision can be quickly restored; Early hazard assessment can help a jurisdiction determine improvements that are best made before the event and that can be included in a Capital Improvement Program. There is also some limited Federal funding for pre-disaster mitigation. Do you want to provide seismic valving on storage tanks? Is it better to maintain storage or allow flow for firefighting even if it means the tank will drain?
Design issues
There is a lack of consistent system seismic analysis and design standards. Resilience is a system-level concept. This requires each component to be designed to ensure the system can perform as intended and restore services after an earthquake promptly to meets societal needs. The system-level design does not require the complete prevention of damage or service outage, but it does require proper management to ensure services are provided to customers and users when they need them in a disaster. Users can adapt during a disaster and utilize alternative means for obtaining water and sewage services for a short timeframe. Alternatives include drinking bottled water; using water from lakes, rivers, and swimming pools to fight fires; and using portable toilets when the sewage collection system is not working. This all requires coordination with the system-level and component-level design assumptions. A good design will perform a systems evaluation with all the known seismic hazards. The analysis uses the post-earthquake damaged system in a hydraulic analysis through tools like Water Network Tool for Resilience (WNTR) (Klise et al., Reference Klise, Bynum, Moriarty and Murray2017a; Reference Klise, Hart, Moriarty, Bynum, Murray, Burkhardt and Haxton2017b; Reference Klise, Murray and Haxton2018) to check the component-level design assumptions and fragilities to ensure the system can perform in a post-earthquake environment to meet expected service recovery times (Abrahams et al., Reference Abrahams, Van Pay, Sattar, Johnson, McKittrick, Bartels, Butcher, Rubinyi, Mahoney, Heintz and Kersting2021). The problem that currently exists is a lack of system-level and component-level performance objectives for the water sector (Gilbert et al., Reference Gilbert, Butry, Helgeson and Chapman2015). Additionally, there is limited information on the fragilities of water systems, which demands further study to improve analysis.
Digital resilience
The increasing cyber-attacks on water sector utilities have forced utilities to address cybersecurity issues under the larger digital resilience umbrella following the current frameworks proposed by different federal agencies and associations (USDHS, 2015; NIST, 2018).
System-of-systems approach for digital resilience
Previous studies have recommended systems thinking approaches for building digital resiliency into the water and wastewater infrastructures that can be regarded as cyber-physical systems (Tuptuk et al., Reference Tuptuk, Hazell, Watson and Hailes2021). The major need in this area is to integrate the computational and physical capabilities to control and monitor physical processes. This is currently being undertaken through the increasing use of ‘smart’ systems due to the emergence of Internet of Things (IoT). Studies have shown that most of the research in this area is related to cybersecurity issues in drinking water systems and can benefit from investigating interdependencies with wastewater, stormwater and irrigation systems (Tuptuk et al., Reference Tuptuk, Hazell, Watson and Hailes2021). The City of Toronto has developed a Digital Infrastructure Plan to tackle issues from the digital-social systems like personal information and privacy, security, data management, procurement, intellectual property, consumer protection among others (Patriarca et al., Reference Patriarca, Simone and Di Gravio2022). Similarly, Singapore has developed a National Cybersecurity Masterplan as part of the Smart Nation vision (Chia, Reference Chia2016). The plan recommends integrating of all the city’s services by enhancing the digital resiliency through investments in new technologies, regular cybersecurity training and awareness programs for employees, and coordination across multiple city agencies and partners. Other efforts to study the cyber-physical systems in Israel have shown that the answer to future cyber security challenges should include a greater integration of both the private and public sector, and of local and national governments rather than applying a top-down centralized approach through national programs (Tabansky, Reference Tabansky2017).
Whole-life approach for management of risks
Efforts to include lifecycle management of risks and enhancing digital resilience are few. Efforts include building digital resiliency throughout the lifecycle based on the cloud applications for managing data and performing optimizations wastewater treatment plants and pipelines at the BlueKolding utility in Denmark (Regmi, Reference Regmi2022). The main challenge observed in this case was the uncertainty in the beginning of the journey of cloud application and replacement of entire IS systems with new technologies. In contrast, the use of lifecycle approach for deploying digital tools for the Waterschapsbedrijf Limburg (WBL) utility in the Netherlands helped them successfully roll out features in small increments starting with a successful Proof of Concept, after which a pilot was done on one of WBL’s wastewater collection and conveyance systems (containing six pumping stations). After the successful pilot, the solution was rolled out to the collection and conveyance systems of all 17 wastewater treatment plants (149 pumping stations in total). Similar success was observed for the Kempner Water Supply Corporation in the US where the application of digital tools to operate pump stations and to control optimally variable frequency drives (VFD) and pumps to assess efficiency while accounting for dynamic system conditions. This resulted in the overall reduction of lifecycle costs, 23% energy savings and reduction by 77% of peak pressure transients during pump starts and stops, from 152 to 35 psi (Regmi, Reference Regmi2022).
Furthermore, Tuptuk et al. reviewed existing research efforts until Tuptuk et al. (Reference Tuptuk, Hazell, Watson and Hailes2021) to enhance the security of water as cyber-physical systems (Tuptuk et al., Reference Tuptuk, Hazell, Watson and Hailes2021). They found that water systems have received lesser attention when compared to other critical infrastructure with most of the studies focusing on cybersecurity of drinking water treatment, supply and distribution, owing to its criticality (Patriarca et al., Reference Patriarca, Simone and Di Gravio2022). However, the digital transformation of utilities with reliance on technologies like sensors, wireless data communication, cloud computing, databases, and control systems may introduce new uncertainties in the collected data and create localized or system-wide shutdowns due to software bugs, extreme climatic conditions, and irregular power supply (Oberascher et al., Reference Oberascher, Dastgir, Li, Hesarkazzazi, Hajibabaei, Rauch and Sitzenfrei2021).
Inevitably issues of human factors arise. Since the COVID-19 pandemic, utilities are looking for creative solutions in the form of better telecommunication tools to enhance their workforce resilience to protect worker safety and retain knowledge from the retiring workforce. The City of Fort Myers has investigated the effectiveness of Augmented or Mixed Reality (A/MR) knowledge management systems to aid their efforts with institutional knowledge capture and transfer as well as providing the operational staff with safe tools to perform their functions remotely (Aldridge and Newberg, Reference Aldridge and Newberg2022). Frameworks such as Safe and SuRe (Butler et al., Reference Butler, Farmani, Fu, Ward, Diao and Astaraie-Imani2014) can also help utilities in raising awareness of the need to tailor approaches to encourage the necessary cultural change across the water sector. Humans can also present major challenges and abuse their access privilege to disrupt services. In contrast, the famous cyber-attack in 2000 on Maroochy Water Services in Australia was an intentional, targeted attack by a knowledgeable person on an industrial control system necessitating the utility to focus on controlling internal attacks by disgruntled employees and identifying ways to retake control of hijacked systems (Abrams and Weiss, Reference Abrams and Weiss2008). As digital resilience is very context-dependent, many utilities have created protocols and strategies to enhance their cyber resilience. For example, the City of Boca Raton in Florida concluded that it needed solutions to better manage its network traffic and monitor plant floor security after an external cyberattack locked up its water treatment plant’s Supervisory Control and Data Acquisition (SCADA) system causing the plant to shut down for nearly 8 h (Horta, Reference Horta2007). More recent case studies stress the importance of having cyber forensic systems in place to understand the types of malware and their behavior, develop more robust cybersecurity protocols, and prevent future attacks (Binnar et al., Reference Binnar, Dalvi, Bhirud and Kazi2021).
Future perspective for water sector resilience
To ensure water sector resilience, in the future, it will be essential to take a more proactive and forward-looking approach that leverages technology, data, and innovative solutions. The use of data and technology will play a key role in enhancing water sector resilience. This includes the use of real-time monitoring systems, predictive analytics, and artificial intelligence to optimize water management and reduce the impact of disruptions. The adoption of Internet of Things (IoT) devices, for example, will provide valuable data on water consumption and distribution, enabling water utilities to quickly identify and respond to stresses and needs. Robust cybersecurity measures will need to be implemented to protect against cyberattacks, as well as the development of physical security measures, such as backup systems, to ensure continuity of service in the event of accidental or malevolent disruptions.
Sustainability and resilience depend on each other but they also may call for diverging actions. Because of this, it is essential to find the right balance with tradeoffs. Sustainability calls for sensible and parsimonious use of limited resources, and a minimal impact on the environment. The sustainable practices based on environmental, social and governance (ESG) framework strategies support water sector infrastructure and create sustainable communities. At the same time, long-term sustainability depends on infrastructure resilience where the infrastructure built today can serve communities for many years, weathering possible disruptions without the need for major reconstruction. However, infrastructure resilience often calls for significant use of scarce resources with significant environmental impact, which in turn hurts sustainability. A crucial challenge that will likely be the focus of significant research in the coming years is to find solutions that are both sustainable and resilient (NSF ERC, 2019). Also, we need to develop methodologies and metrics to measure resiliency of future water sector infrastructure systems and/or vulnerabilities. Since we may create inadvertently future risks with our current decisions.
Data and models for a virtual representation of reality will provide powerful tools to inform and educate through benchmarking, performance metrics, and explaining decision-making. Integrated risk and resilience management strategies will require models to predict: (1) the time-varying state of infrastructure accounting for the effects of deterioration; (2) multi-hazards induced physical damages to infrastructure components; (3) physical and service recovery of the damaged infrastructure; and (4) time-varying measures of infrastructure resilience. The models will need to be integrated into an overarching decision framework (Figure 14) to optimize performance, and resilience objectives.

Figure 14. System-of-systems and whole-life resilience management framework.
A transformation of infrastructure management from an asset inventory-centric focus to a higher systematic-level water infrastructure resilience management will need to take place (NSF ERC Planning Grant, 2020; NSF ERVA, 2022; UN, 2022). Interdependency will become the nexus of infrastructure asset management (IM) and resilience management (RM) due to the growing interconnectedness of system of water systems. A multi-disciplinary synergistic approach that addresses and coordinates both IM and RM analytical requirements will need to be developed for informed decision-making (Figure 15). Some utilities will need additional support and guidance on how to integrate and manage information in a holistic approach. Some utilities will hire full-time emergency preparedness, security, and asset management staff to help manage this effort, but there will likely still be a struggle to sort through the various methodologies and decide which courses of action to take. Several states are beginning to tie emergency preparedness into asset management. Formal programs or guidance will be needed to explain how this is done or tools will need to be developed to tie together the whole picture.
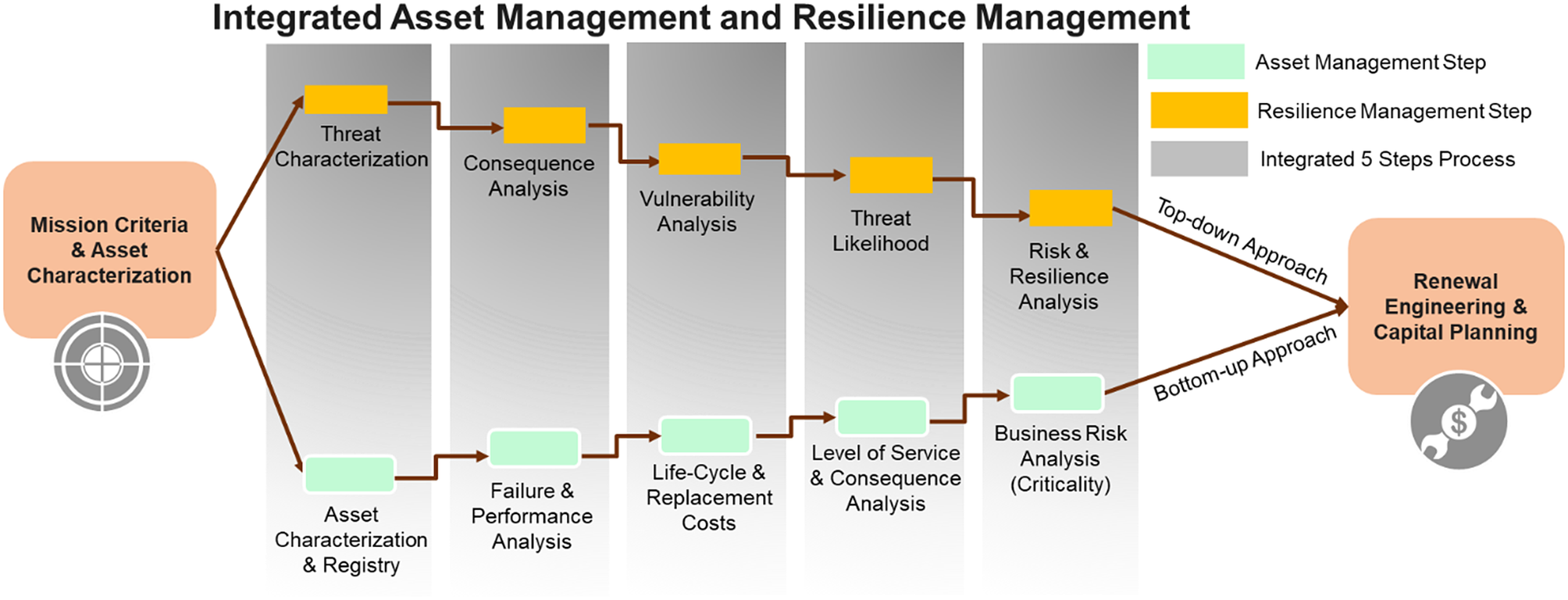
Figure 15. Integrated asset and resilience management for decision-support.
Digitalization of the water sector, specifically the growing applications of digital twins and Artificial Intelligence (AI), can be the winning strategy to achieve service reliability, resilience, and sustainability. However, the challenges to digital resilience mentioned in this study concern digital technologies, workforce, and cyberinfrastructure cannot be ignored. Utilities can follow a risk-based framework by providing a common language between various stakeholders for understanding, managing, and communicating risks in maintaining digital resilience (Figure 16). It can also help to identify and prioritize actions to reduce cybersecurity risk, and to provide a tool for aligning policy, business, and technological approaches to managing that risk.

Figure 16. A risk-based system-of-systems and whole-life framework for digital resilience.
Digital transformation of water sector for resilience
Emerging digital technologies and AI have the potential to enhance the resilience of water infrastructure systems, by providing a rapid and accurate assessment of asset conditions and supporting robust decision-making and adaptation. An important concept underpinning the vision of a resilient water sector is the use of Big Data and Cyber-Enabled technologies where all people, things, and processes are connected through a common platform designed at various levels to integrate stakeholders, knowledge, and system processes. Digital technologies and AI can deliver more efficient, rapid, and reliable evaluations and enable better decision-making, based on actionable performance indicators before, during, and after the occurrence of extreme events. Digital technologies offer unlimited potential to transform the world’s water systems, helping communities become more resilient, innovative, and efficient, and in turn, helping them build a stronger and more economically viable foundation for the future (NSF Innovation Ecosystem Report, 2020). Exploiting the value of data, automation, and artificial intelligence allows utilities to extend water resources, reduce coastal communities’ risk, expand assets’ life, provide the basis for water security, and more (Future of Water Summit Report, 2022).
We must design, assess, and improve water systems considering all aspects at a river basin scale, to reduce risk, increase resilience and provide for a healthy prosperous community. Advances in the Internet of Things, communication systems, data analytics, automation, high-performance computing, and human–computer interfaces should be leveraged to develop a digital, AI-enabled cyberinfrastructure platform. The Digital-Water ecosystem as shown in Figure 17 has the potential to meet the ultimate water sector goal. Water sector utilities need to develop short- and long-term research activities focused on identifying, prioritizing, and addressing research needs related to developing scalable, flexible, and security services for a wide range of system management requirements from detecting consumer behavior to guiding real-time response to emerging threats in different types of water systems. The availability of a large amount of integrated water data and computational resources, together with the development of advanced AI-enabled techniques tailored to specific applications can foster more robust, trustworthy models and algorithms to process and analyze water systems at the river-basin scale. At a granular level, machine learning algorithms should be used to reconstruct missing data and/or identify and fill data quality gaps (Karpatne et al., Reference Karpatne, Atluri, Faghmous, Steinbach, Banerjee, Ganguly, Shekhar, Samatova and Kumar2017). At a higher level, data-driven surrogate modeling can create end-to-end digital twins.
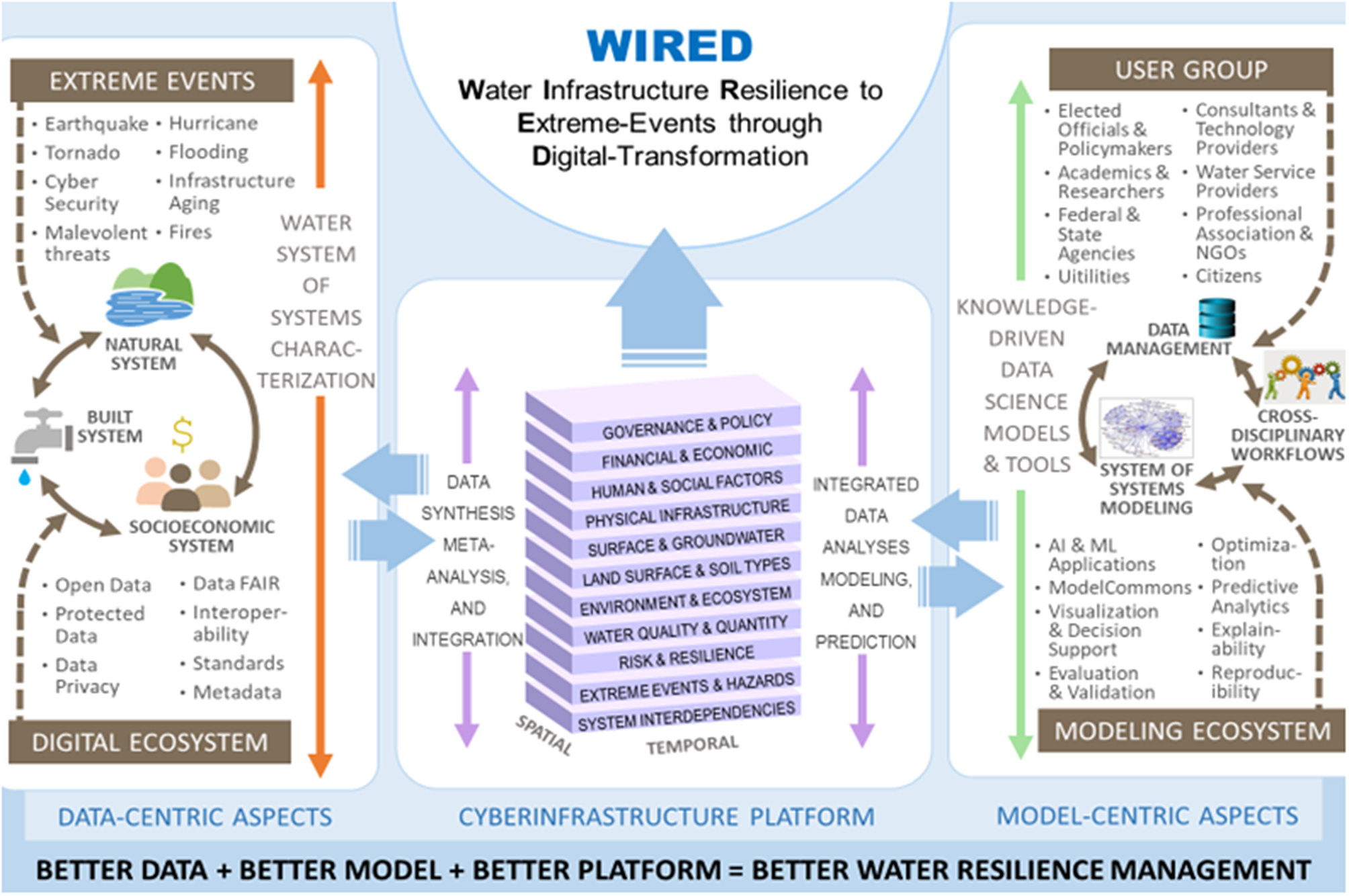
Figure 17. A cyberinfrastructure framework for water sector sustainability and resilience.
Many challenges and struggles can be associated with deploying AI in a system. A survey (IDC, 2022) found that more than 50% of technology buyers struggle with various factors like lack of skilled personnel, lack of AI/ML operations tools and techniques, lack of adequate volume and quality of data, and trust and governance issues associated with AI. Effective AI requires data diversity. Similarly, the full transformative impact of AI can be realized by using a wide range of data types. Adding layers of data can improve accuracy of models and the eventual impact of applications. Most of the utilities are using a wide range of data types, unstructured data use is still largely untapped. In addition, data continues to be siloed, making it difficult to access and govern appropriately. Unfortunately, due to these challenges, water utilities are spending more time on tasks that are not actual data science. The water sector stakeholders should work closely to understand needs, ‘pain points’, preferences, and readiness to operationalize technologies, as well as barriers. Cybersecurity, privacy, fairness, and trustworthiness are examples of known concerns among water stakeholders that should be addressed using approaches such as federated learning, differential privacy, and cost-sensitive learning. Data analytics and system-of-systems knowledge will lead to an understanding of the interdependencies between built and natural systems and communities.
Conclusions and recommendations
Water sector systems are exposed to numerous threats, the potential impacts of which range from inconsequential (infrastructure systems can absorb them without change in performance) to society-threatening (restoration taking years). The concept of a social–ecological–technical system-of-systems and whole-life resilience approach provides a valuable perspective for developing countermeasures to address many of these threats. It allows water sector utilities to deal with moderate disruptions in a more economical manner and is essential in overcoming extreme and less-known threats. The growth in uncertainties and societal costs of disruptions is placing social–ecological–technical resilience among the major considerations for the operation of the water sector infrastructure systems. The water sector resilience must go hand-in-hand with strategies and practices to ensure reliable operation under normal and stressed conditions.
The message is clear: we can no longer ignore the deterioration of the Nation’s water sector infrastructure in the face of emerging and uncertain risks. Considering possible future work several themes appear highly promising for operationalizing resilience in the water sector:
-
(1) Resilience strategy that represents the ‘Social–Ecological–Technical System-of-Systems and Whole-Life’ approach would be very useful for studying and operationalizing the resilience of water sector infrastructure systems;
-
(2) Resilience of water sector infrastructure systems should be considered holistically, inclusive of ‘Physical and Digital Resilience’ as well as integrated ‘Asset Management and Resilience Management’ for sustainability of water sector infrastructure;
-
(3) The Water Sector is facing a dynamic and complex risk environment in which the full impacts of disruptions and the potential cascading impacts are not fully understood. There is a need for fundamental research and development in this area.
Open peer review
To view the open peer review materials for this article, please visit http://doi.org/10.1017/wat.2023.3.
Data availability statement
Data sharing are not applicable to this article as no datasets were generated or analyzed during the current study.
Author contribution
All authors contributed to the research conception, literature review, practice review, and development of this manuscript. All authors reviewed and commented on prior manuscript versions. All authors read and approved the final manuscript.
Financial support
Initially, the work was supported by the NIST under the Community Resilience Program. In part, the work was also supported by NSF ERC Planning Grant No. 1936893 to Virginia Tech. Any opinions, findings, conclusions, or recommendations expressed in this material are those of the authors and do not necessarily reflect the views of the NIST and NSF. We would also like to thank Sustainable Water Infrastructure Management (SWIM) Center at Virginia Tech for in-kind support.
Competing interest
There are no financial or nonfinancial interests to disclose by the authors.
Comments
This is a invited manuscript