INTRODUCTION
Fossil bones and teeth represent significant sources of proxy information on past environments, the geographic mobility and paleodiet of the Pleistocene animals, and post-depositional taphonomic processes. The present climate warming observed in NE Siberia with annual air temperature rise by up to +3°C during the last four decades (Danilov and Degteva, Reference Danilov and Degteva2018; Czerniawska and Chlachula, Reference Czerniawska and Chlachula2020) has triggered exposures of Pleistocene-age geological sections sealing rich fossil fauna records (e.g., Plotnikov et al., Reference Plotnikov, Protopopov, Kolesov and Klimovskiy2016; Kirillova et al., Reference Kirillova, Borisova, Chernova, van Kolschoten, van der Lubbe, Panin and Pečněrová2020; Chlachula et al., Reference Chlachula, Cheprasov, Novgorodov, Obada and Little2021), some in a unique state of preservation (Boeskorov, Reference Boeskorov2009; Boeskorov et al., Reference Boeskorov, Protopopov and Mashchenko2013; Chernova et al., Reference Chernova, Kirillova, Boeskorov, Shidlovskiy and Kabilov2015). Bones are exposed to a variety of factors during the fossilization processes that are reflected in the changes of original chemical composition and micromorphology of the bone tissue formed by two principal components: collagen and calcium phosphate. During the early stages of diagenesis, micro-organisms (e.g., bacteria, fungi, cyanobacteria) have a crucial effect on bone preservation because of their expansion into the bone tissue immediately after the animal's death. Microbial activity is strongly affected by the specific ambient conditions of the microorganisms that are required for their growth and reproduction (Bell et al., Reference Bell, Skinner and Jones1996, Trueman and Martill, Reference Trueman and Martill2002, Turner-Walker, Reference Turner-Walker2012). Traces of the microbial activity captured within the bone microstructure provide a unique opportunity to investigate past depositional (burial) environments and reconstruct long-term conditions for fossil material preservation.
A detailed study of microbial attacks (Hackett, Reference Hackett1981) resulted in differentiation of two main categories of microbial bio-erosion. The first category of microbial attacks created by bacteria, microscopic focal destruction (MFD), is characterized by a poriferous structure with pores 0.1‒1 μm in diameter (spongiform porosity) (Jackes et al., Reference Jackes, Sherburne, Lubbel, Barker and Wayman2001, Turner-Walker et al., Reference Turner-Walker, Nielsen-Marsh, Syversen, Kars and Collins2002) and the presence of hyper-mineralized rims of amorphous bioapatite (Jans et al., Reference Jans, Nielsen-Marsh, Smith, Collins and Kars2004, Jans, Reference Jans, Wisshak and Tapanila2008). The MFDs are divided into three sub-groups based on the shape and the extension within the bone tissue conditioned by different tissue types: (1) linear longitudinal, which is usually circular with the smallest foci (LLF) 5‒10 μm in diameter; (2) budded, which are more irregular (a shape similar to a bud) with the larger foci (BF) 30‒60 μm in diameter; and (3) lamellate, which is the least common foci (LF) 10‒60 μm in diameter, reflecting the lamellar structure of the bone tissue, and are the only ones can pass through the cement line. The budded and lamellate foci can exceptionally reach up to 250 μm in diameter and both have granulous infill (Hackett, Reference Hackett1981, Jans et al., Reference Jans, Nielsen-Marsh, Smith, Collins and Kars2004). The second category of microbial attacks, called “Wedl tunneling,” displays empty tunnels 5‒15 μm in diameter with sharp and well-calcified walls (Marchiafava et al., Reference Marchiafava, Bonucci and Ascenzi1974; Hackett, Reference Hackett1981; Jans, Reference Jans, Wisshak and Tapanila2008; Turner-Walker and Jans, Reference Turner-Walker and Jans2008) that are often attributed to the activity of aquatic organisms such as fungi (Wedl, Reference Wedl1864; Marchiafava et al., Reference Marchiafava, Bonucci and Ascenzi1974), algae (Davis, Reference Davis1997; Fernández-Jalvo et al., Reference Fernández-Jalvo, Andrews, Pesquero, Smith, Marín-Monfort, Sánchez, Geigl and Alonso2010; Nacarino-Meneses et al., Reference Nacarino-Meneses, Chinsamy, Mayda, Kaya and Erismis2021), and cyanobacteria (Davis, Reference Davis1997; Turner-Walker and Jans, Reference Turner-Walker and Jans2008).
Bacteria are the most varied microorganisms living within a broad range of diverse environments. They are adapted to specific temperatures, pH values, oxygen, or soil contents. Aerobic soil bacteria are mostly involved in post-mortem changes in the bone microstructure and contribute preferably to the deterioration of the cortical bone tissue near the periosteal and endosteal surface (Turner-Walker, Reference Turner-Walker, Pinhasi and Mays2008, Reference Turner-Walker2012, Reference Turner-Walker2019; Turner-Walker and Jans, Reference Turner-Walker and Jans2008, Fernández-Jalvo et al., Reference Fernández-Jalvo, Andrews, Pesquero, Smith, Marín-Monfort, Sánchez, Geigl and Alonso2010; Müller et al., Reference Müller, Chadefaux, Thomas and Rieche2011; Fernández-Jalvo and Andrews, Reference Fernández-Jalvo and Andrews2016; Kendall et al., Reference Kendall, Eriksen, Kontopoulos, Collins and Turner-Walker2018). Nevertheless, in several studies, great importance in bone degradation has been given to intestinal bacteria (Bell et al., Reference Bell, Skinner and Jones1996; Jans, Reference Jans, Wisshak and Tapanila2008; White and Booth, Reference White and Booth2014), although this has never been clearly proven. Bacterial activity also may induce the process of bio-mineralization, especially in the form of iron- or manganese-bearing minerals, such as oxides and hydroxides (Mandernack et al., Reference Mandernack, Post and Tebo1995a, Reference Mandernack, Fogel, Tebo and Usuib; Kremer et al., Reference Kremer, Owocki, Królikowska, Wrzosek and Kazmierczak2012), phosphates (Turner-Walker, Reference Turner-Walker2012), or iron sulphides created by sulphate-reducing bacteria (Turner-Walker, Reference Turner-Walker1998).
Apart from the microbial activity, other physical, (geo-)chemical, and biological factors jointly affect fossil osteological material causing mineral content instability. Carbonate apatite [Ca10-a(PO4)6-b(CO3)c(OH)2-d], which is the principal bone constituent chemically similar to hydroxyapatite [Ca5(PO4)3(OH)], and other chemical components are not resistant to postmortem mineralogical enrichment or depletion (Berna et al., Reference Berna, Matthews and Weiner2004; Kremer et al., Reference Kremer, Owocki, Królikowska, Wrzosek and Kazmierczak2012; Reynard and Balter, Reference Reynard and Balter2014; Keenan and Engel, Reference Keenan and Engel2017). A combination of techniques is applied to characterize the bones or teeth chemical element composition and the state of diagenesis (Goodwin et al., Reference Goodwin, Grant, Bench and Holroyd2007, Piga et al., Reference Piga, Santos-Cubedo, Sola, Brunetti, Malgosa and Enzo2009, Galiova et al., Reference Galiova, Kaiser, Fortes, Novotny, Malina, Prokes and Hrdlicka2010; Lebon et al., Reference Lebon, Reiche, Bahain, Chadefaux, Moigne, Frohlich, Semah, Schwarcz and Falgueres2010; Swanston et al., Reference Swanston, Varney, Coulthard, Feng, Bewer, Murphy, Hennig and Cooper2012; Liao et al., Reference Liao, Sun, Li, Sa, Lu, Lin, Xu, Zhan, Pan and Xu2019). Quantification of the diagenetic changes and their visualization is usually performed by laser ablation-inductively coupled plasma-mass spectrometry (LA-ICP-MS).
This study presents results on varying bio-degradation of the Late Pleistocene (MIS 3) megafauna skeletal remains sealed in fossil permafrost. A marked discrepancy of microbial activity controlling bone preservation is documented on the example of an intact and perfectly preserved bison metacarpus and a weathered horse metacarpus. The analyses point to specific taphonomic histories predetermining the diagenetic processes and differential bone matrix preservation. The results add to our understanding of the contextual particularities of osteological material decay and the associated chemical and biological agents that affect the state of preservation of paleontological assemblages.
Study Area
The study area is located in the central and northern part of the Verkhoyansk Region, NE Yakutia (Fig. 1), within a low-relief zone characterizing the middle reaches of the Yana River basin. The sub-Arctic climate at the northern limits of the boreal forest is very harsh, sub-continental, with extreme seasonality. Mean annual air temperature (MAAT) in the central part of the basin (Batagay) is −15°C (average January temperature −45.2°C, average July temperature +16.2°C), 50–70 days per year are frost-free, the mean annual precipitation is 200–300 mm (Agroclimate Database, 1963; Bulygina and Razuvaev, Reference Bulygina and Razuvaev2012). Continuous permafrost, reaching up to 300 m in depth, underlies the broader territory (Harada et al., Reference Harada, Wada, Sueyoshi and Fukuda2006; Grosse et al., Reference Grosse, Robinson, Bryant, Taylor, Veremeeva, Schirmeister and Harde2013; Fedorov et al., Reference Fedorov, Vasilyev, Torgovkin, Shestakova, Varlamov, Zheleznyak and Shepelev2018).

Figure 1. Location map of the study area with the investigated sites.
During the last glacial maximum (LGM), summer temperature was ~6–8°C lower and winter temperature ~12–14°C lower than the present (Frenzel, Reference Frenzel, Frenzel, Péczi and Velichko1962; Velichko, Reference Velichko1993). Progressive warming and ground surface heat absorption observed across Yakutia have triggered changes in the local hydrology systems, mass gravity-flow processes, thermokarst formation, and frozen-ground thaw (Duchkov, Reference Duchkov, Lombardi, Altunina and Beaubien2006; Popp et al., Reference Popp, Diekmann, Meyer, Siegert, Syromiatnikov and Hubberten2006; Iijma et al., Reference Iijma, Fedorov, Park, Suzuki, Yabuki, Maximov and Ohata2010; Romanovsky et al., Reference Romanovsky, Drozdov, Oberman, Malkova, Kholodov, Marchenko and Moskalenko2010; Malkova et al., Reference Malkova, Pavlov and Skachkov2011; Veremeeva and Glushkova, Reference Veremeeva and Glushkova2013; Fedorov et al., Reference Fedorov, Gavriliev, Konstantinov, Hiyama, Iijima and Iwahana2014; Schuur et al., Reference Schuur, McGuire, Schadel, Grosse, Harden, Hayes and Hugelius2015; Czerniawska and Chlachula, Reference Czerniawska and Chlachula2020). The northern lowlands are seasonally water-saturated and boggy as a result of accelerated river-flow dynamics due to the progressing late spring–early summer permafrost thaw. Associated channel erosion and bank undercutting expose the pre-modern (late Pleistocene–early Holocene) organic-rich grounds with embedded fossil fauna and macrofloral remains (Chlachula et al., Reference Chlachula, Czerniawska, Pestereva and Pesterev2014). The northern boreal forest of larch (Larix sibirica), transgressing into tundra-forest in the upper elevations, is the principal biotope in the lowlands and adjoining mountain foothills (Andreev et al., Reference Andreev, Klimanov and Sulerzhitsky2001).
The geologically youngest formations that are the subject of investigations related to the mid-last glacial (MIS 3/Karga) interstadial (55–24 ka BP). This time interval is associated with the principal late Pleistocene paleontological and early cultural occurrences in the Yana-Indigirka-Kolyma lowlands that are buried in fossiliferous sandy, gravelly alluvial and silty, clayey lacustrine facies (Biske, Reference Biske1957; Boeskorov, Reference Boeskorov2005, Reference Boeskorov2010; Lazarev, Reference Lazarev2008; Lazarev et al., Reference Lazarev, Grigor'ev, Plotnikov and Savvinov2011; Cheprasov et al., Reference Cheprasov, Obada, Grigoriev, Novgorodov and Marareskul2015; Chlachula et al., Reference Chlachula, Cheprasov, Novgorodov, Obada and Little2021). During the early part of the interstadial (55‒38 ka BP), the central and upper Yana River valley was covered by parkland-steppes and mixed taiga forests that supported populations of large herbivorous animals (mainly mammoth, woolly rhinoceros, bison, and horse), as evidenced by the rich osteological records and fossil mammal coprolites retrieved from the cryolithic formations (Chlachula et al., Reference Chlachula, Czerniawska, Pestereva and Pesterev2014, unpub.). Fossil herbaceous plants (~70‒80%) with sporadic arboreal taxa of Larix, Betula, Alnus, and Salix point to relatively warm conditions (Murton et al., Reference Murton, Edwards, Lozhkin, Anderson, Savvinov, Bakulina and Bondarenko2017; Ashastina et al., Reference Ashastina, Kuzmina, Rudaya, Troeva-Werner, Schock, Roemermann and Reineck2018). Overall, the Karga Interstadial was biotically the most productive mid-last glacial stage, with rich paleontological sites distributed across Yakutia (e.g., Boeskorov, Reference Boeskorov2010; Boeskorov et al., Reference Boeskorov, Protopopov and Mashchenko2013; Plotnikov et al., Reference Plotnikov, Protopopov, Kolesov and Klimovskiy2016).
The Yana River Basin (YRB Site)
The first investigated site (YRB/Yunigen) is located in the middle reaches of the Yana River (68°54′20.78″N, 134°26′42.75″E, 85 m asl.), ~250 km downstream of the Batagay Site (BAS) (Fig. 1). Contextually, the YRB site is geologically structured by ancient colluvia overlying a laterally eroded gravelly terrace platform exposed 20‒30 m above the present river channel. There, as well as at other loci in the nearby area, abundant osteological remains of Pleistocene ungulates (mammoth, rhinoceros, bison, horse, and reindeer, among others), along with large carnivores (bear, lion, hyena, wolf), are found in intact positions or partly re-deposited in frozen strata. These sandy gravel and sandy/silty clay formations are exposed due to permafrost thaw and/or water pumping (Chlachula et al., Reference Chlachula, Czerniawska, Pestereva and Pesterev2014). The analyzed osseous material originates from the upper part of the documented stratigraphic section (Fig. 2A).

Figure 2. Views of the sample study sites. (A) Yana River Site; 1 = cryolithic exposure of the Late Pleistocene fossiliferous formation; 2 = the investigated section; (B) Batagay Site and the Batagay thermokarst sinkhole stratigraphy; 1 = composite site stratigraphy; 2 = thermokarst sinkhole, eastern wall; 3 = the Equus bone, SE section. Photographs by J. Chlachula, August 2014.
The YRB/Yunigen site is one of the key paleoenvironmental reference sites for chronostratigraphic correlations and paleoecological interpretations of the mid-last glacial ecosystems and early human (Paleolithic) occupation in the (sub-)Arctic East Siberia.
The Batagay Thermokarst Sinkhole (BAS Site)
The second paleontological site (67°34′55.9″N, 134°45′55.8″E; 241 m asl/top surface) lies near the confluence of the Yana and Adycha rivers at the southern margin of a major thermokarst sinkhole/cryolithic crater (the Batagay “proval”), 15 km SE of Batagay town (the Verkhoyanskiy District) (Fig. 1). The geomorphic setting represents the most spectacular place of active permafrost-degradation processes in Siberia (Kunitsky et al., Reference Kunitsky, Syromyatnikov, Schirrmeister, Skachkov, Grosse, Wetterich and Grigoriev2013). The Batagay depression, reaching the present size of ~800×500 m and a depth of ~60–90 m with a lateral face retreat of 10‒20 m/year, demonstrates the intensity and the scale of the current frozen ground thaw in the Siberian North due to the dramatic warming trend observed in Yakutia over the past 40 years (Fedorov et al., Reference Fedorov, Vasilyev, Torgovkin, Shestakova, Varlamov, Zheleznyak and Shepelev2018; Chlachula and Czerniawska, 2021).
The vertical sinkhole is transgressing in the upper part into a conically (~45°) shaped outer margin. On the eastern side of the depression, erosion has exposed a complete suite of geological units formed by dark gray silts and laminated fine-grained sands with a slightly wavy structure in the lower and middle part of the sequence. These alluvial/colluvial deposits are overlain by well-sorted aeolian sands. The conformably sequenced sedimentary series represents ancient (Middle–Late Pleistocene) Yana River shallow-water paleochannel deposits and colluvia. Several fossil soils in the middle and upper part of the sinkhole (Fig. 2B) point to stages of the ancient surface stabilization and pedogenesis. Numerous erosion-exposed remains of Pleistocene flora and fauna are found in isolated positions and in discrete humus-rich layers aligned by ice lenses (Chlachula et al., Reference Chlachula, Czerniawska, Pestereva and Pesterev2014; Ashastina et al., Reference Ashastina, Kuzmina, Rudaya, Troeva-Werner, Schock, Roemermann and Reineck2018; Opel et al., Reference Opel, Murton, Wetterich, Meyer, Ashastina, Günther and Grotheer2019). Preservation of the incorporated paleontological remains is excellent (Lazarev et al., Reference Lazarev, Grigor'ev, Plotnikov and Savvinov2011). The fossil records are the subjects of current contextual taphonomic studies.
AIMS, MATERIAL, AND METHODS
The aim of the study was to define the specific agents affecting differential bone structure degradation and assess the causes of diagenetic changes (chemical and biological) in the analyzed fossil fauna remains. These remains include a bison metacarpus (the YRB site) and a horse metacarpus (the BAS site) that were removed from the Late Pleistocene (MIS 3) permafrost along with other fossils in August 2014 (J. Chlachula). Taphonomic investigations at both sites were performed.
Field studies
The first, well-preserved bison (Bison sp.) right metacarpal bone (Fig. 3A) was collected in situ directly from a buried permafrost ground (Unit 4) of the YRB site underlain by a solid ice body after exposure of the section by water pumping. The isolated bone was fresh in condition, yellow-white colored, with no macroscopic signs of microbial decay, post-mortem mechanical damage, or Pleistocene carnivore scavenging (e.g., tooth marks). The fused epiphyses indicate an adult animal. The radiocarbon age determination of the sample is 45,000 ± 5000 yr BP (Poz-97051).
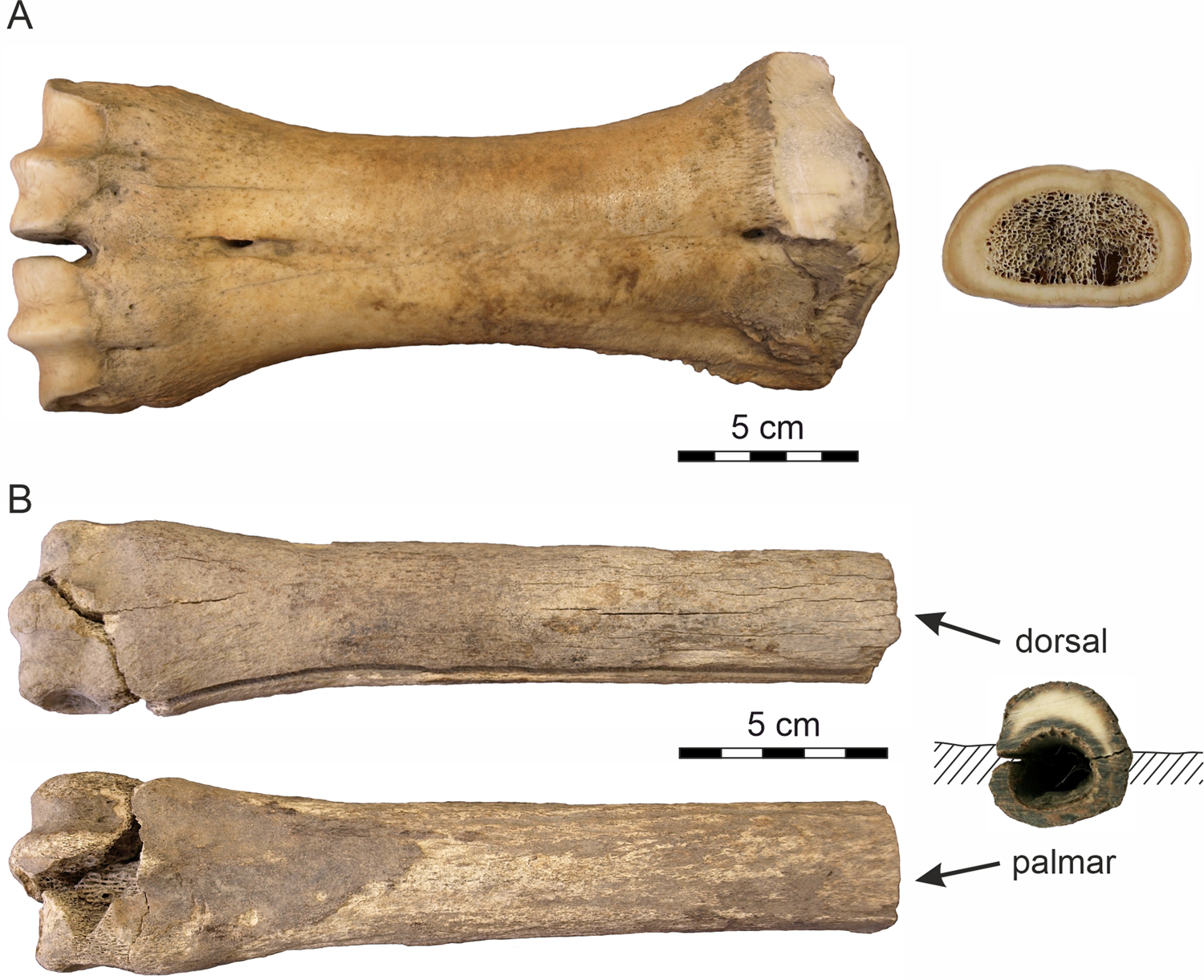
Figure 3. Analyzed bones. (A) The metacarpus of Bison sp.; (B) the metacarpus of Equus sp.
The second sample represents a partly weathered right Equus sp. metacarpus (Fig. 3B) released from the Batagay permafrost sinkhole (the BAS site) as a result of seasonal melting of the massive Pleistocene cryogenic formation. The bone, which was found ~30 m from the sinkhole's vertical wall, presumably was exposed on the present surface at the bottom of the crater for some time (2‒5 years) prior to sample collection, as deduced from the rate of lateral wall retreat of the thermokarst crater (10‒20 m per year; Kunitsky et al., Reference Kunitsky, Syromyatnikov, Schirrmeister, Skachkov, Grosse, Wetterich and Grigoriev2013). The radiocarbon age of the bone, 37,800 ± 1700 yr BP (Poz-97053), corroborates the 14C date of 36,300 ± 700 yr BP (Poz-75782) on fossil roots from the same section (Murton et al., Reference Murton, Edwards, Lozhkin, Anderson, Savvinov, Bakulina and Bondarenko2017). Both dates confirm the mid-last glacial (MIS 3) age of the upper part of the Batagay stratigraphic sequence (Vasil'chuk and Vasil'chuk, Reference Vasil'chuk and Vasil'chuk2019). With respect to the site chronostratigraphy, the initial position of the fossil was likely at ~20 m depth below the present top surface and originally sealed within the sedimentary bed (Unit 4) of massive interbedded colluvial sands (Fig. 2B) (the basal part of Unit 5; Murton et al., Reference Murton, Edwards, Lozhkin, Anderson, Savvinov, Bakulina and Bondarenko2017). The thinner palmar side of the fossil was covered by silty sediments, whereas the thicker dorsal side was exposed and subjected to the extreme, seasonally fluctuating temperature conditions. The distal epiphysis indicates an adult individual.
Both samples were wrapped up in plastic bags and preserved in a frozen state prior to their air transport to the laboratory of the Department of Geological Science at the Masaryk University in Brno, Czech Republic, for analytical processing.
Laboratory studies
Laboratory studies focused on assessing intensity of the past/present microbial activity in the analyzed fossil records as a study case from the (sub)arctic zone of Northeast Siberia exposed to the current climate warming. Both samples were studied by a combination of several analytical methods including scanning electron microscope analysis (SEM-BSE), electron microprobe analysis (EMPA), laser ablation inductively coupled plasma-mass spectrometry (LA-ICP-MS), and mercury intrusion porosimetry (HgIP).
Sample preparation
The diaphyses of the bison and horse metacarpal bones were cross-cut into slices 1 cm thick. The metaphyseal and epiphyseal parts, where the cortical bone is rather thin or absent, were excluded from the analyses. The slices retaining the thick cortical bone were cut radially (Bison: ~1.2×0.8×0.5 cm; Equus: ~1.2×0.8×0.5 cm [dorsal side], and ~1.0×0.5×0.5 cm [palmar side]) and leached in acetone for 24 hours to remove fat residues. Subsequently, the samples were dried in an automatic desiccator at 40°C for 48 hours. The prepared samples were used for the BSE-SEM and HgIP studies, with the former vacuum-coated with gold.
EMPA and LA-ICP-MS analyses were performed on a polished block section of the bone slice embedded in epoxy resin (~2.5 cm in diameter). The sample surface was carbon coated for EMP study. The palmar and dorsal bone sides were treated separately.
Scanning electron microscopy (SEM-BSE)
A Jeol JSM-6490LV electron microscope in the Department of Geological Sciences, Masaryk University, Brno (MU Brno), was used for detailing the bone microstructure on the surface as well as cross-section using an accelerating voltage of 15 kV.
Mercury intrusion porosimetry
The AutoPore IV 9500 mercury intrusion porosimetry analysis (Department of Geological Sciences, MU Brno) was applied to assess the overall distribution of pore sizes represented in the horse and bison cortical bone tissue. The porosimetry model operates at a pressure of 414 MPa covering pore diameters within the range of 0.003‒360 μm. The pore size distribution followed the classification of Turner-Walker et al. (Reference Turner-Walker, Nielsen-Marsh, Syversen, Kars and Collins2002): S- or sub-fibril porosity = pores with a diameter <0.1 μm; M- porosity induced by microbial activity = pores with a diameter in the range of 0.1‒1 μm; L- physiological and dissolution porosity = pores with a diameter >1 μm.
Electron microprobe analysis
The EMP analysis using a Cameca SX100 electron microprobe (Department of Geological Sciences, MU Brno) detailed the chemical composition of the cross-sections through the horse and bison cortical bone tissue (from the periosteal to the endosteal margins, including pore fillings) in the wavelength dispersive mode and an accelerating voltage of 15 kV, beam current of 10 nA and a spot size of 5 μm. Data were processed using the X-Phi matrix correction (Merlet, Reference Merlet1994) and recalculated in the FORMULA program after the values below the detection limit were removed.
Altered apatite was normalized to 12.5 anions and 8 cations according to an idealized formula for hydroxyapatite of Ca5(PO4)3(OH). The presence of phosphate with a significant Fe content was proven in some vascular canals, predominantly within the endosteal and periosteal area. Data were converted to the formula of vivianite Fe3(PO4)2•8(H2O) that was normalized to eight cations. The analyzed phosphates contain H2O either in the form of molecular water (vivianite) or as a hydroxyl anion (apatite). Because the H2O and carbon contents cannot be determined by routine EMP analysis, they were not included in the formula recalculations (Tables 1–3).
Table 1. Chemical composition of the Bison bone carbonate-apatite Ca5(PO4)2.5(CO3)0.5(OH) obtained by electron-microprobe and calculated chemical formulae. wt% = weight percent, b.d.l. = below detection limit; apfu = atoms per formula unit.

Table 2. Chemical composition of the Equus bone carbonate-apatite Ca5(PO4)2.5(CO3)0.5(OH) obtained by electron-microprobe and calculated chemical formulae. wt% = weight percent, b.d.l. = below detection limit; apfu = atoms per formula unit.

Table 3. Chemical composition of the Equus bone vivianite Fe3(PO4)2·8(H2O) obtained by electron-microprobe and calculated chemical formulae. wt% = weight percent, b.d.l. = below detection limit; apfu = atoms per formula unit.

Laser ablation-inductively coupled plasma-mass spectrometry (LA-ICP-MS)
LA-ICP-MS analyses were conducted with two different instrumental setups. The laser ablation system Analyte G2 (Teledyne CETAC Technologies, Omaha, USA) was connected to a double-focusing sector field ICP-MS Element 2 (Thermo Fisher Scientific, Bremen, Germany). This system is installed at the Laboratory of Atomic Spectrochemistry (LAS), MU Brno. The other system, located at the University of Technology in Brno, consists of quadrupole based ICP-MS Agilent 7900 (Agilent Technologies, Santa Clara, CA, USA) coupled with the Analyte Excite+ laser ablation system (Teledyne CETAC Technologies, Omaha, USA). Both instruments used the ArF* excimer laser ablation systems operating at 193 nm equipped with a tunable 2-volume ablation cell HelEx II. Ablation was carried out in the He atmosphere. The operating conditions of the ICP mass spectrometers were optimized using NIST SRM 612.
The elemental distributions across the bone samples were investigated by a line of spots using the laser beam spot size of 65 μm and individual spot spacing of 100 μm, fluence of 9 J/cm2, frequency of 10 Hz, dwell time of 60 s, and washout of 60 s. Time-resolved signals were recorded for the following isotopes: 23Na+, 24Mg+, 27Al+, 28Si+, 31P+, 42Ca+, 43Ca+, 44Ca+, 45Sc+, 47Ti+, 51V+, 53Cr+, 55Mn+, 57Fe+, 63Cu+, 66Zn+, 75As+, 88Sr+, 137Ba+, and 208Pb+.
2D elemental mapping of the small specific areas (structure of the diagenetically modified phosphate phase, in which a change caused by the microbial activity was exempt) was accomplished by line scanning. The laser spot diameter was 8 μm, scan speed 4 μm/s, fluency 9 J/cm2, and frequency 10 Hz. A distance of 15 μm between individual lines was kept constant. Only the selected elements were subjected to the elemental association investigation (matrix: Ca, P; minor: Na, Mg; and trace elements: Mn, Fe, Sr, Ba, Al, Si).
Standard reference materials NIST SRM 1486 bone meal and NIST SRM 610 glass were used for quantification purposes, and Ca was used as an internal reference element. Ca content was determined using an electron microprobe. The limit of detection was calculated as three times the standard deviation of the He-Ar gas blank divided by sensitivity.
The detection limits were as follows: 3 mg/kg Na; 0.08 mg/kg Mg; 0.09 mg/kg Al; 12 mg/kg Si; 0.57 mg/kg P; 19 mg/kg Ca; 0.02 mg/kg Sc; 0.67 mg/kg Ti; 0.01 mg/kg V; 0.19 mg/kg Cr; 0.02 mg/kg Mn; 1.9 mg/kg Fe; 0.05 mg/kg Cu; 0.02 mg/kg Zn; 0.05 mg/kg As; 0.01 mg/kg Sr; 0.04 mg/kg Ba, and 0.04 mg/kg Pb in a case of line of spots (Table 4); and 4.8 mg/kg Na; 21 mg/kg Mg; 8.4 mg/kg Al; 180 mg/kg Si; 19 mg/kg P; 750 mg/kg Ca; 0.9 mg/kg Mn; 90 mg/kg Fe; 0.79 mg/kg Sr; and 0.76 mg/kg Ba when sampling bone tissue via the line scan mode.
Table 4. Elemental contents in dorsal and palmar bone sample determined by LA-ICP-MS expressed as median and median absolute deviation. Contents are listed in mg/kg. The median was chosen so that it would be feasible to eliminate the presence of cracks or physiological pores filled with resin, sediment, or a minor phase with a different chemical composition compared to the phosphate phase.
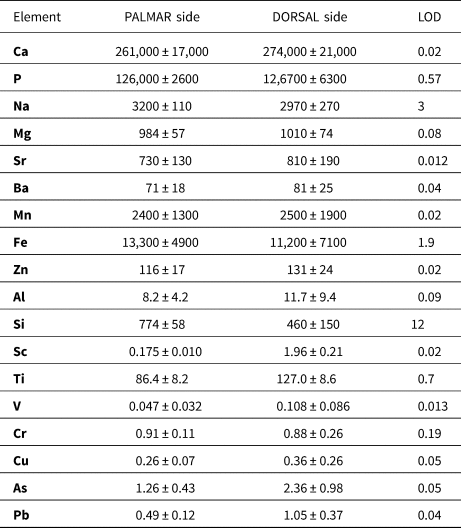
LA-ICP-MS is a widespread technique that is applied for qualitative and quantitative elemental distribution in different types of hard tissues (bone, tooth enamel, dentine, and cementum). This method can directly analyze solid samples with spatial resolution down to the μm scale with high sensitivity and low detection limits. Other benefits are isotopic and multi-element analyses and minimum destructiveness.
The LA-ICP-MS analysis was applied only to the horse bone for detailed evaluation of the alteration processes.
RESULTS
Metacarpal samples preservation
The bison metacarpus was completely skeletonized. The bone surface was smooth, without cracks/fissures or flakes (Fig. 3A), which corresponds to weathering stage 1 (Behrensmayer, Reference Behrensmeyer1978) applied to periglacial environments (Todisco and Monchot, Reference Todisco and Monchot2008). There is no surface pattern indicating activity of carnivores/scavengers. The 14C age of the sample corresponds to the time interval of climatically moderate interstadial conditions.
The horse metacarpus, which was exposed on the surface 2‒5 years prior to being collected, was less well preserved with a partially damaged distal epiphysis. The bone surface was coarse in places, particularly on the palmar side and towards the epiphyses where a spongious tissue is visible. The dorsal side displays numerous, recently created narrow longitudinal cracks on the external bone surface, with moderately developed flaking. This preservation state of horse metacarpus corresponds to weathering stage 2‒3 (Todisco and Monchot, Reference Todisco and Monchot2008). The bone has a dark-colored periosteal and endosteal surface, while the thinner palmar side is completely colored. Increased mobility of humic substances and the presence of Fe-hydroxide coatings on the sealing sand grains and a dark mineral staining (Fig. 3B) point to a periodic ground drying and moistening under a relatively warm to moderate past climatic regime.
Bone micromorphology and microbial bioerosion
Bone degradation is indicated by specific biogenetic changes. In the perfectly preserved bison metacarpus, honeycomb-like pits were observed on the periosteal and endosteal surface in various extents (Fig. 4A–D), most often close to vascular supply (Fig. 4E). Some of these structures contained smaller pores ~1 μm in diameter (Fig. 4F).

Figure 4. Micromorphology and microbial bioerosion SEM images of the fossil bison bone. (A, B) smooth periosteal surface with the resorption pits; (C, D) isolated honeycomb-shaped resorption pits; (E) resorption pits surrounding vascular canal; (F) detail of pits showing microbial attacks to the Bison bone structure.
In the horse metacarpus, both biotic and abiotic destructive factors that affected the degree of bone preservation have been revealed inside the bone structure. The SEM analysis provided evidence of extensive bacterial bio-erosion on the periosteal and endosteal surface, where the meandering or variously branched channels consisting of small micro-tunnels 0.3–1 μm in diameter were observed (Fig. 5A).

Figure 5. SEM images of the fossil horse bone showing microbial bioerosion. (A) Micro-tunnels created by bacteria on the bone surface; (B) typical microscopic focal destruction (MFD) extended in the endosteal bone area; (C) magnified linear longitudinal (LLF) and budded foci (BF) bounded by hypermineralized rims; (D) bacterial budded foci (BF) overlapping the demineralized zone; (E) magnified bone tissue with signs of advanced demineralization at the periosteal margin; (F) superficially dissolved bone tissue spreading from the periosteal margin with gradually demineralized secondary osteon (white square) and mineral vivianite (Vv) in the vascular canal.
Typical bacterial microscopic scale destruction was observed on the cross-section of the cortical bone (Equus), predominantly in the sub-endosteal and less frequently in the sub-periosteal area. The linear longitudinal (LLF) and budded (BF) foci were detected on the bone cross-section (Fig. 5B–D). Chemical demineralization distinctly prevails in the periosteal area, especially on the palmar side of the bone (Fig. 5E, F). Fillings of the vascular canals consist mainly of the vivianite (Vv) (Fig. 5F).
Pore-size distribution
The well-preserved bison bone displays a complete pore size distribution in cortical tissue almost identical with the pore size distribution in the comparative modern bovine bone sample (Fig. 6A, B). The pore size distribution in native mammalian cortical tissue is given by the canalicular porosity (the smallest pores in the area ~0.1‒0.3 μm), the osteocyte lacunae porosity (pore size ranges = 2‒7 μm), and the physiological porosity (pores >10 μm diameter, which represent vascular canals) (Cowin, Reference Cowin1999; Turner-Walker et al., Reference Turner-Walker, Nielsen-Marsh, Syversen, Kars and Collins2002). However, HgIP has a problem with detecting the porosity of osteocyte lacunae because without sufficiently high pressure, mercury does not penetrate the lacuna and is trapped in the canaliculi, the so-called ink-bottle effect (Turner-Walker et al., Reference Turner-Walker, Nielsen-Marsh, Syversen, Kars and Collins2002).

Figure 6. Pore size distributions in the studied cortical tissues. (A) Recent and fossil bovines; (B) periosteal, medial, and endosteal area in fossil bison; (C) the fossil horse; (D) periosteal and endosteal areas of the horse metacarpus palmar side; (E) periosteal and endosteal areas of the horse metacarpus dorsal side. The gray-highlighted areas represent ranges of porosity induced by microbial activity.
The bison cortical tissue displays an increased volume of the S-porosity (≤ 0.1 μm) and L-porosity (>7 μm). There is no indication of porosity induced by microbial activity (M) within the range of 0.1–1 μm in the fossil bison metacarpus (Fig. 6B).
The weathered Batagay horse bone showed increased M-porosity (Fig. 6C), with the largest pore volume maximum at pores of ~0.35 μm in diameter (the palmar side and the periosteal area) where extensive demineralized zones were detected (Fig. 6D–F). In the endosteal area, pores of ~0.28 μm in diameter represented a significant peak within M-porosity. Almost the same trend was observed in the endosteal area of the dorsal side.
Chemical composition
The mineral component of the studied bison and horse bone (Tables 1, 2), reflecting the state of diagenesis and osteological preservation, corresponds to the carbonate-apatite content with the ideal formula Ca5(PO4)2.5(CO3)0.5(OH). This contributes to the bioapatite composition in extant remains, where the actual carbonate content varies among different species and increases with age within a given species (Legros et al., Reference Legros, Balmain and Bonel1987). Mineral re-crystallization into hydroxyapatite results from postmortem unstable conditions (e.g., Sillen and Parkington, Reference Sillen and Parkington1996; de Sousa et al., Reference de Sousa, Eltink, Oliveira, Félix and Guimarães2020). The deficit in the sum of oxides measured by the EMP analysis could be a result of the porous structure of the bone material and the presence of organic components or carbonates or both. The exogenous elements were incorporated into the structure of apatite in place of the cation/anion position or concentrated inside the physiological pores or cracks.
The analytical study was performed only on the horse metacarpus by the LA-ICP-MS on the palmar (Fig. 7A–D) and dorsal (Fig. 7E–H) sides of the bone (Figs. 7, 8). Distributions of the Ca and P matrix (Fig. 7A, E) and the Na and Mg elements (Fig. 7B, F) show their representation within the sample with some apparent fluctuations on the dorsal bone side. The LA-ICP-MS shows increased concentrations of Mn and Fe (Fig. 7C, G), which are orders of magnitude lower in native bone (Skinner, Reference Skinner and Selinus2005). It also shows the presence of other exogenous elements, such as Sr, Ba (Fig. 7B, F), and As, Cu, and V (Fig. 7H), incorporated into the bone mineral matrix at the expense of major elements Ca and P (Fig. 7A, E) and minor elements Na and Mg (Fig. 7F). Al and Si were present in a physiological foramen or cracks, which corresponded with the highest peaks in two-spot positions (Fig. 7D).

Figure 7. Two-dimensional elemental distribution on the fossil horse bone (BAS) determined by LA-ICP-MS in the spot mode line across the palmar (A‒D) and dorsal (E‒H) side. The line of spots was situated from periosteum (left) to endosteum (right).

Figure 8. Two-dimensional elemental mapping (LA-ICP-MS analysis) of the fossil horse cortical bone. (A) Images showing vascular canals filled with iron phosphate vivianite; (B) dissolved bone tissue of the secondary osteon with the vascular canal and secondary microporosity in the vicinity filled with manganese-/iron- (oxy)hydroxides in the periosteal bone area; (C) the microbial focal destruction with almost no impurities contrasted with the surrounding bone tissue in the endosteal bone area.
Bone structure 2D element imaging
2D bone mineralogy mapping was performed in three selected loci of the Equus metacarpus (Fig. 8). These loci show concentrations close to the endosteal part of the compact bone where the vascular systems occur. The Ca and P maps indicate homogeneous distributions over the entire area of the analyzed surface except for osteons, pores, and cracks. Alteration of the phosphate phase is apparent from the Na, Mn, and Fe distributions. The different structure around vascular canals is most evident from the change in Na distribution where the area in the vicinity of pores is Na depleted and subsequently is bordered with “rings” having higher Na content (Fig. 8A). The differential distribution of Mn and Fe highlights a distinct structure and an incorporation of elements into the phosphate phase. The presence of Mn may indicate microbial activity.
The secondary mineral phase (Fig. 8A) fills the vascular pores and the nearby cracks, as evident from the distribution of Mn and Fe, and corresponds to the presence of vivianite. The sums of oxides in the analyzed horse bone vivianite captured mainly near the periosteum are ~7% higher compared to the ideal vivianite (73%). This may be due to the presence of Fe or Mn phosphate with a lower H2O content (Pratesi et al., Reference Pratesi, Cipriani, Giuli and Birch2003; Chukanov et al., Reference Chukanov, Scholz, Zubkova, Pekov, Belakovskiy, Van and Lagoeiro2014) (Table 3). Element mapping shows a positive association among P, Fe, Mn, and Mg within the vascular canal, with Mg and Mn incorporated into the vivianite structure.
The distributions of Ca and P, as with the principal matrix elements, are rather uniform across the whole analyzed area, however their content decreases in the vicinities of bone margins, physiological pores, or cracks (Fig. 8B). Secondary microporosity close to the periosteum was filled exclusively with Mn (up to 0.5%m/m; the red area) and Fe (up to 6.0%m/m; the red area), while the surrounding tissue was distinctly Fe saturated (~4.3%m/m; the green area). This porous structure showed the high content of Mn2+ ions.
The quantified distributions of Ca, P, Na, Fe, and Mn display very heterogeneous spatial patterns for all of these elements (Fig. 8C). The MFDs near the endosteum show absence of impurities or secondary minerals in contrast with the surrounding bone tissue. In the MFDs, which are characterized by the presence of a hypermineralized rim that is resistant to leaching, there was also no contamination of exogenous elements.
ENVIRONMENTAL AND TAPHONOMIC IMPLICATIONS OF BONE DECAY
Several diagenetic agents were identified analytically in the studied bison bone (45.0 ± 5.0 14C ka BP) and horse bone (37.8 ± 1.7 14C ka BP) from the central Yana River valley and the Batagay thermokarst sinkhole. The rate of decay of mammalian remains in the High Arctic environments (Sutcliffe, Reference Sutcliffe and Harington1990) is controlled by (1) the geological context and position of fossils (buried vs. surface-exposed); (2) the amount of ground moisture; (3) pH of the sealing deposits; (4) duration of annual snow cover; and (5) the scale of exposure to atmospheric processes (temperature, precipitation).
The Bison sp. (YRB Site)
The complete skeletonized Bison bone (Fig. 3A) displays no traces of transport—only minor surface weathering (WS 1) and lack of incorporated exogenous elements. This indicates that the bone was not exposed on the surface for a long time after the animal's death when it was buried and subsequently sealed in the gravelly cryolithic deposits. Only superficial resorption pits with a honeycomb pattern (Fig. 4A‒F) have been observed in the bone, indicating a short-term effect of microbial activity. A comparable honeycomb pattern is known in the tooth enamel and bones from continental aquatic environments (Fernández-Jalvo and Andrews, Reference Fernández-Jalvo and Andrews2016). Howship's lacunae, which form during osteoclastic resorption, have a similar shape (Relucenti et al., Reference Relucenti, Miglietta, Bove, Donfrancesco, Battaglione, Familiari, Barbaranelli, Covelli, Barbara and Familiari2020). Because the microstructures also have been observed on the periosteal surface of the bone, microorganisms such as cyanobacteria or algae appear to be the most likely causal agents.
The bison body decomposition began shortly after death of the animal in a climatically moderate interstadial sub-arctic environment. Under colder conditions, the main soft-tissue decay can take 4‒7 days (Janaway et al., Reference Janaway, Percival, Wilson and Percival2009). Body skeletonization is significantly accelerated by the activity of insects, other arthropod species, and soil microorganisms (Smith, Reference Smith1986). Even in periglacial conditions, insect development flourishes within the range of 10‒15°C, although many common blow flies cease laying their eggs at temperatures below 10°C (Erzinclioglu, Reference Erzinclioglu1996). A maggot (fly larva) can develop completely in ca. 30 days at temperatures ~15°C (Grassberger and Reiter, Reference Grassberger and Reiter2002). Even under cold weather conditions, the temperature for maggot growth can be favorable, depending on the degree of infestation of the carcass (Byrd and Castner, Reference Byrd and Castner2010).
During the early stage of the Karga (MIS 3) interstadial (ca. 55‒38 ka BP), the MAAT in North Yakutia was close or even higher than today, as indicated by the permafrost-sealed paleoecology records (Chlachula et al., Reference Chlachula, Cheprasov, Novgorodov, Obada and Little2021). Especially in summer, insects affected and contributed to accelerated carcass decay of the large roaming fauna. Bacterial reproduction is largely slowed at <12°C, and bacterial growth is substantially inhibited at temperatures <4°C (Binford, Reference Binford1978; Micozzi, Reference Micozzi, Haglund and Sorg1997). At temperatures below −5°C, the activity of enzymes and microorganisms is interrupted, and no decomposition occurs (Janaway et al., Reference Janaway, Percival, Wilson and Percival2009). Rapid drying of soft tissues due to solar radiation and freezing causes extensive loss of moisture and nutrients from the cadaver, and most of the carrion fauna ceases bone decomposition (Carter et al., Reference Carter, Yellowlees and Tibbett2007; Smith, Reference Smith1986). The nearly perfect preservation of the bison bone is primarily due to rapid burial of the bison body/skeleton and long-term preservation in stable cryolithic conditions over the past 45,000 years.
The Equus sp. (BAS Site)
Very low temperatures (average January temperature −51°C based on organic microinclusions in ice-wedges of the Batagay yedoma dated to 33,800–27,100 cal. yr) (Vasil'chuk and Vasil'chuk, Reference Vasil'chuk and Vasil'chuk2019) attest to the long-term frozen ground conditions that contributed to the supreme preservation of the fossil remains. The poor preservation of the Equus sample (Fig. 3B) provides evidence of much more dynamic environmental conditions. The lower stage of surface abrasion probably caused by wind erosion along with the absence of a chalky external layer indicate that the horse bone was not exposed to external conditions for a long time before burial in solid permafrost. The differential preservation of the dorsal and palmar sides of the horse bone indicates different mechanisms of recent weathering, with the palmar side buried in sediments and the dorsal side open-air exposed to multiple freeze-thaw and dry-wet cycles after bone exhumation from the thawing cryolithic deposits of the Batahay thermokarst sinkhole. The desiccation process (solar exposure and freezing) due to the extreme seasonal conditions caused loss of moisture from the bone, which generated cracking and flaking (Miller, Reference Miller and Swanson1975; Murphy et al., Reference Murphy, Barnett, Holloway and Sheldon1981) of the dorsal side of the bone and alteration of its physical properties including decrease of natural biomechanical resistance (Johnson, Reference Johnson1985).
The horse bone was buried under relatively warm/moderate Late Pleistocene climatic conditions and changing ground humidity. This is indicated by increased mobility of humic substances, the presence of Fe-hydroxide coatings on the sealing sand grains, and a dark mineral coloring on the periosteal and the endosteal bone surfaces (Fig. 3B). The prominent longitudinal crack was probably created as a result of shrinkage of collagen fibers during recent desiccation. Hydrolysis of the collagen is reflected by the increase of S-porosity, mainly in the periosteal area of the palmar side (Fig. 6D). However, no massive microcracks have been observed in the horse bone, which is in contrast to (sub-)fossil bones deposited in warm and arid settings (Nacarino-Meneses, Reference Nacarino-Meneses, Chinsamy, Mayda, Kaya and Erismis2021) or affected by moisture and temperature fluctuations (Pfretzschner and Tütken, Reference Pfretzschner and Tütken2011; Mayer et al., Reference Mayer, Hubbe, Botha-Brink, Ribeiro, Haddad- Martim and Neves2020).
The well-documented MFDs in the Equus sample (Figs. 5A–D, 6C, D) show at least two generations of bacterial populations, which must have been controlled by past burial of the bone within the active permafrost layer with periods of increased microbial activity during the summer months of the MIS 3 interstadial and inhibited activity for most of the year. The absence of exogenous elements within the MFDs (Fig. 8C) indicates that bacterial alterations formed before the bone was contaminated with the exogenous elements.
Arctic soils and even the underlying permafrost contain a wide range of bacteria (Nelson and Parkinson, Reference Nelson and Parkinson1978; Shi et al., Reference Shi, Reeves, Gilichinsky and Friedmann1997; Vorobyova et al., Reference Vorobyova, Soina, Gorlenko, Minkovskaya, Zalinova, Mamukelashvili, Gilichinsky, Rivkina and Vishnivetskaya1997; Vishnivetskaya et al., Reference Vishnivetskaya, Erokhina, Spirina, Shatilovich, Vorobyova and Gilichinsky2001, Reference Vishnivetskaya, Petrova, Urbance, Ponder, Moyer, Gilichinsky and Tiedje2006; Gilichinsky et al., Reference Gilichinsky, Vishnivetskaya, Petrova, Spirina, Mamykin, Rivkina, Margesin, Schinner, Marx and Gerday2008), including gram-positive bacteria (e.g., Firmicutes, Actinobacteria, Cyanobacteria) and gram-negative bacteria (e.g., Alpha-, Beta-, Gamma-Proteobacteria), of which Bacillus (gram +) and Pseudomonas (gram -) have been described by C.A. Baud (Reference Baud, Duday and Masset1986) as the most active in postmortem bone attack. Aerobic soil bacteria most probably were involved in the formation of MFDs inside the Yakutian horse bone. The arctic microbial communities are also highly resistant to thawing stress (Gilichinsky et al., Reference Gilichinsky, Vishnivetskaya, Petrova, Spirina, Mamykin, Rivkina, Margesin, Schinner, Marx and Gerday2008).
The presence of exogenous elements inside the fossil bone (Figs. 7, 8; Tables 1–4) shows their increased concentrations in the sealing geological sediments (Lambert et al., Reference Lambert, Simpson, Weiner and Buikstra1985). Mineral dissolution mainly in the periosteal area (Fig. 5E, F) most probably resulted from waterlogged and poorly oxygenated conditions that caused chemical hydrolysis of the collagen fibers accompanied by destabilization of bone minerals (Turner-Walker, Reference Turner-Walker, Pinhasi and Mays2008). The hypermineralized rim shows a high resistance to leaching due to recrystallization of hydroxyapatite, which became larger and more stable due to diagenesis (Hedges, Reference Hedges2002). This is documented by MFD overlapping of chemically altered zones close to the vascular canal (Fig. 5D).
The presence of vivianite in the vascular canals (Figs. 5F, 8A; Table 3) attests to the low-oxygen, periodically or permanently waterlogged, often alkaline conditions (McGowan and Prangnell, Reference McGowan and Prangnell2006, Rothe et al., Reference Rothe, Kleeberg and Hupfer2016). The source of hydrogenous iron in the form of Fe3+ oxy-hydroxides relates to the sealing sediment. Vivianite precipitation is often mediated by metal-reducing bacteria from the soil (Zachara et al., Reference Zachara, Fredrickson, Li, Kennedy, Smith and Glassman1998) in the early stage of bone diagenesis (Turner-Walker, Reference Turner-Walker2012). Vivianite is usually found on the bone surface as a product of bone mineral recrystallization (Berg et al., Reference Berg, Doring, Suchenwirth and Weiner1969; Piepenbrink, Reference Piepenbrink1989; Thali et al., Reference Thali, Lux, Lösch, Rösing, Hurlimann, Feer, Dirnhofer, Königsdorfer and Zollinger2011; Turner-Walker, Reference Turner-Walker2012; Papageorgopoulou et al., Reference Papageorgopoulou, Link and Ruhli2015). Vivianite also may be present inside the bone and tooth (Sekanina, Reference Sekanina1937; Johanson, Reference Johanson1976; Turner-Walker, Reference Turner-Walker2012) and in the mummified soft tissues sealed in permafrost (Tessadri, Reference Tessadri2000; Pabst et al.; Reference Pabst, Letofsky-Papst, Bock, Moser, Dorfer, Egarter-Vigl and Hofe2009, Papageorgopoulou et al., Reference Papageorgopoulou, Link and Ruhli2015). The low vivianite concentrations found on the dorsal side of the horse bone corroborate the nature of this easily soluble and highly unstable mineral (Rothe et al., Reference Rothe, Kleeberg and Hupfer2016) after recent bone exposure and oxidation.
Precipitation of Fe-Mn oxide-hydroxides occurred as a result of burial of the Equus bone in the active layer after the formation of the MFDs. The increased content of Fe and Mn in the partially dissolved periosteal margin (Fig. 7C, G) indicates that Fe-Mn oxide-hydroxides precipitation was contemporaneous with the secondary microporosity. It is impossible to distinguish between the porosity induced by microbial activity and porosity caused by abiotic (e.g., chemical) processes solely according to the HgIP. Although mercury porosimetry provided a quality image of the pore size distribution in the bone, this method should be used in combination with SEM to identify properly the factors involved in porosity changes. The extent of bone microbial degradation must be verified by the histology study using other methods (e.g., SEM). The Fe3+ oxy-hydroxides most likely were precursors of vivianite, which originated as a result of reaction of the pore-percolating water with metal-reducing bacteria under reduction (anaerobic) depositional conditions. Absence of vivianite on the dorsal side of the Equus bone is likely associated with recent bone surface weathering and obliteration of the mineral in environmentally unstable oxygenic (open-air) conditions. The high concentration of Mn2+ ions in the endosteal margin (Fig. 8B) indicates precipitation of hydroxides under alkaline conditions (pH ~7) and a later transformation into manganese oxides after open-air exposure from permafrost grounds (Stumm and Morgan, Reference Stumm and Morgan1996; Morgan, Reference Morgan2005). Freshwater bacteria Pseudomonas putida (Villalobos et al., Reference Villalobos, Lanson, Manceau, Toner and Sposito2006; Bargar et al., Reference Bargar, Fuller, Marcus, Brearley, De la Rosa, Webb and Caldwell2009) also may have contributed to the observed Mn oxides accompanied by the brown colored FePO4 on the bone surface. On the other hand, Na and Mg have been leaching out from the bone (Fig. 8A). A similar pattern of Mn, Fe uptake, and Na loss was observed in the bovine bone from a boggy environment (Turner-Walker and Peacock, Reference Turner-Walker and Peacock2008).
The above exogenous elements affected the bone chemical composition as a result of burial within the active permafrost layer. In periglacial areas, bone microbiota (e.g., fungi, algae, bacteria, and Protozoa) along with chemical degradation strongly depend on ground temperature and water availability (White and Hannus, Reference White and Hannus1983; Von Endt and Ortner, Reference Von Endt and Ortner1984). The reconstructed MAAT at the Batagay (Equus) site during MIS 3 derived from ice-wedges (−35‰ δ18O) are among the lowest in Yakutia (Opel et al., Reference Opel, Murton, Wetterich, Meyer, Ashastina, Günther and Grotheer2019). Despite extremely cold winters, the summer months were rather warm with high melt water supply (Opel et al., Reference Opel, Murton, Wetterich, Meyer, Ashastina, Günther and Grotheer2019) approaching the present ones recorded in NW Yakutia (Chlachula and Czerniawska, 2021). The interstadial environmental conditions were favorable for incorporation of exogenous elements into the bone structure prior to its complete freezing, as well as soon after its exposure.
The combined analytical multi-proxy evidence of differential preservation of the studied Pleistocene fossils demonstrates a very rapid rate of horse bone structure deterioration shortly after exposure from the permafrost. A range of a few years is assumed to be sufficient for disintegration of an intact bone, following several tens-of-thousands of years of permafrost burial.
CONCLUSIONS
The osteological material of the mid-last glacial (MIS 3) interstadial from the Yana Basin was treated as an example of the diametrically different fossil bone states (compact fresh versus rather weathered bones) of the large Late Pleistocene herbivores Bison and Equus. The differential preservation of the analyzed fossil remains illustrates the locally specific environmental histories and the post-mortem and post-depositional processes of the fossil fauna records that resulted in the spectrum of bone structure conservation and diagenesis. The applied analytical methods identified several diagenetic factors in the comparative osteological samples. The Bison metacarpus remained almost microbiologically intact over the last ca. 45,000 years while frozen in permafrost until its recovery. Only superficial resorption pits with honeycomb pattern, most probably created by aquatic microorganisms, have been observed on the bone surface. On the other hand, the Equus metacarpus exposed on the surface during the 2‒5 most-recent years shows more extensive bacterial degradation with typical microscopic focal destruction, as well as chemical degradation of the periosteum. The study shows that the application of the HgIP frequently used for estimation of the degree of microbial attack is not reliable if applied separately because other (chemical) processes also can produce pores of a similar diameter (0.1–1 μm). LA-ICP-MS, which detailed the element distribution in the studied bones and within the specific zones on a micrometer scale, helped to detect specific diagenetic events. Along with EMPA, the analyses gave a clearer image of the nature of the depositional and preservational settings.
Fossil fauna remains of diverse physical preservation and chemical composition constitute an informative proxy for reconstruction of past environments, taphonomies of the fossil fauna communities, and the post-mortem processes of particular osteological records. Solid permafrost provides a perfect long-term cryogenic preservation of organic remains, including soft tissue. On the contrary, due to the current extreme climate shifts in North Siberia and the rising MAAT, destruction/degradation of both the ancient and recent skeletal material is rather rapid once the remains are removed from the intact cryogenic grounds. This awareness contributes to the paleontological field reconnaissance in the (sub-)Arctic zone and enhanced understanding of the forms and intensity of the decay of fossil remains.
Acknowledgments
The paleontological investigations in Yakutia were supported by the North-Eastern Federal University, Yakutsk, as a part of the project “Influence of Climate Change to Natural Environments and Peoples in Northern Yakutia” (Jiří Chlachula and Jolanta Czerniawska, 2014). The analytical study was supported by research project MUNI/1263/2020 of the Faculty of Science at the Masaryk University in Brno, Czech Republic (Martin Ivanov); the Ministry of Culture of the Czech Republic within the development program MK000094862 and the Moravian Museum Brno (Gabriela Calábková); the Student Project Grant MUNI/A/1390/2020 of Masaryk University (Michaela Hložková); and the projects FCH-S-21-7398 of the Czech Ministry of Education, Youth and Sports (Michaela Vašinová Galiová). Jindřich Kynický (BIC Brno) provided funding for the LA-ICP analysis. Kyunnei Pestereeva (Laboratory of Archaeology, the North-Eastern Federal University, Yakutsk) assisted in the Yana region field investigations. The authors thank two anonymous reviewers for their very constructive comments and views that improved the original manuscript.