Introduction
Most chemical reactions are conventionally run in bulk solvent. Our group has been developing a so-called ‘microdroplet fusion mass spectrometry’ that would allow observation of early events in chemical and biochemical processes (Lee et al. Reference Lee, Kim, Nam and Zare2015). During the course of our study, we employed the redox reaction between 2, 6-dichlorophenolindophenol (DCIP) and ascorbic acid and found that the reaction rate was over a 1000-fold higher than the previously reported rate in bulk solution. Recently, a few other groups have explored reactions in droplets using electrospray ionization (ESI) (Badu-Tawiah et al. Reference Badu-Tawiah, Campbell and Cooks2012; Bain et al. Reference Bain, Pulliam and Cooks2015; Banerjee, Reference Banerjee2013; Banerjee et al. Reference Banerjee, Prakash and Mazumdar2011, Reference Brownell, Mccrory, Chidsey, Perry, Zare and Waymouth2013; Fallah-Araghi et al. Reference Fallah-Araghi, Meguellati, Baret, Harrak, Mangeat, Karplus, Ladame, Marques and Griffiths2014; Girod et al. Reference Girod, Moyano, Campbell and Cooks2011; Müller et al. Reference Müller, Badu-Tawiah and Cooks2012; Perry et al. Reference Perry, Splendore, Chien, Davis and Zare2011, Reference Perry, Brownell, Chingin, Cahill, Waymouth and Zare2012). These groups have also reported the acceleration of chemical reaction rates in microdroplets, in both charged droplets surrounded by air and non-charged aqueous droplets in oil. Questions arise: How general is the acceleration of chemical reactions in microdroplets? To what degree can the reaction be accelerated? What types of reactions are applicable for accelerating the reaction in microdroplets? What are the mechanisms of the different reaction rates in the bulk- and microdroplet-chemistry?
To address some of these questions, we have investigated two different reactions in microdroplets. One is a chemical reaction with covalent bond rearrangement and the other is noncovalent protein–ligand interaction. First, the well-known chemical reaction of Pomeranz–Fritsch synthesis of isoquinoline (Bobbitt & Bourque, Reference Bobbitt and Bourque1987; Gensler, Reference Gensler2004; Li, Reference Li2009) was examined in microdroplets generated by the electrospray process (Fig. 1a ). We have also explored the kinetics of cytochrome c and maltose interaction in microdroplets with the droplet fusion mass spectrometry we developed (Fig. 1b ). We report more than a million fold increase of the reaction rate of the Pomeranz–Fritsch synthesis of isoquinoline, and more than a 1000-fold increase of the rate of cytochrome c–maltose binding compared with that in bulk solution. We also consider the evaporation of microdroplets and present arguments that evaporation is not the principal cause for acceleration of reaction rates in the case of fused aqueous droplets.

Fig. 1. Schematic diagrams of the experimental setup used in our study of ‘microdroplet chemistry’. The upper panel (a) shows the electrospray assisted synthesis of isoquinoline, and the lower panel (b) shows the droplet fusion mass spectrometry to study the complexation between maltose and cytochrome c.
Materials and methods
Chemicals and sample preparation
Horse heart cytochrome c, maltose, benzaldehyde, aminoacetaldehyde diethyl acetal, and m-nitrobenzyl alcohol (m-NBA) were purchased from Sigma (St. Louis, MO). HPLC grade methanol, N,N-dimethylformamide (DMF), acetonitrile (ACN), and water were purchased from Fisher Scientific (Nepean, ON, Canada).
Electrospray-assisted Pomeranz–Fritsch synthesis of isoquinoline
1 mmol (110 μl) benzaldehyde was mixed with 1 mmol (145 μl) aminoacetaldehyde diethyl acetal and heated at 100 °C for 2 h to form (Z)-N-benzylidene-2,2-diethoxyethanamine precursor (C, in Scheme 1). Then 5 μl aliquot of the above precursor (C) was dissolved in different solvents (methanol, water, 1:1 DMF–ACN mixture, and 1% m-NBA in water) and electrosprayed in positive ion mode (+5 kV) at a flow rate of 15 μl min−1 through silica tubing (100 μm i.d.) with a coaxial sheath gas flow (N2 at 120 psi). The mass spectrometer inlet capillary temperature was maintained at approximately 275 °C, and capillary voltage was kept at 44 V. The spray distance (the on-axis distance from spray tip to the entrance of the heated capillary; see Fig. 1a ) was kept at 1·5 cm. All experiments were carried out under identical conditions to detect the product by a high-resolution mass spectrometer (Thermo Scientific LTQ Orbitrap XL Hybrid Ion Trap-Orbitrap mass spectrometer).
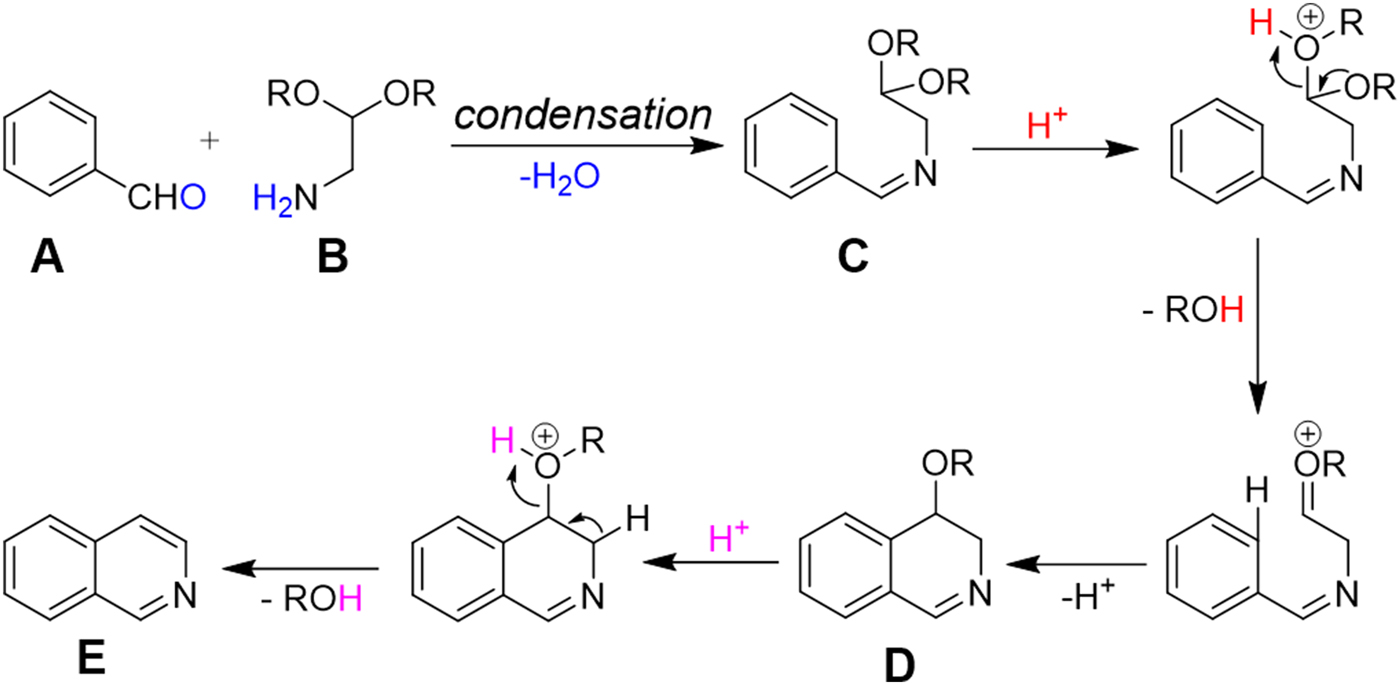
Scheme 1. The plausible mechanism of Pomeranz–Fritsch reaction.
Microdroplet fusion mass spectrometry of cytochrome c–maltose complexation
Thermo Scientific LTQ Orbitrap XL Hybrid Ion Trap-Orbitrap mass spectrometer was used for the cytochrome c–maltose binding studies in fused droplets. Two ESI-like spray sources are equipped with an X–Y–Z micro positioning linear and angular stage for accurate alignment of the two streams of droplets (See Fig. 1b ). This alignment is important for ensuring fusion of most of the incident droplets and to maintain a linear trajectory toward the mass spectrometer inlet. The best alignment was acquired with the angle between two crossed droplet streams at 78°, which showed the highest probability of droplet fusion and straight trajectories of the fused droplets to the inlet of the mass spectrometer. Two aqueous solutions of analytes (cytochrome c at a concentration of 100 μM and maltose at a concentration of 100 mM) were injected from the two ESI sources with a syringe pump (Harvard Apparatus, Holliston, MA) at a flow rate of 30 μl min−1 in positive ion mode. The heated capillary temperature was maintained at approximately 275 °C, and the ion-spray voltage was kept at +5 kV. For measurement of the size and velocities of the fused droplets over a distance x, we used a high-speed optical camera (Phantom v1610, Vision Research, Wayne, NJ).
Results and discussion
Pomeranz–Fritsch synthesis of isoquinoline in charged microdroplets
The charged microdroplet (1–2 μm in diameter) produced by ESI process in positive ion mode is highly acidic and the pH of the droplet continuously decreases during the evolution period (repeated solvent evaporation and Coulomb fission in conventional ESI) of the droplet (Banerjee & Mazumdar, Reference Banerjee and Mazumdar2012; Fenn, Reference Fenn1993; Kebarle & Tang, Reference Kebarle and Tang1993). Here we attempt to induce a typical acid-catalyzed reaction, e.g., Pomeranz–Fritsch synthesis (Bobbitt & Bourque, Reference Bobbitt and Bourque1987; Gensler, Reference Gensler2004; Li, Reference Li2009) of isoquinoline in a charged droplet to investigate whether high proton density in the electrospray droplet surface can accelerate the reaction in a confined space of a continuously evaporating droplet. In bulk solvent, this synthesis is believed to proceed in two steps (see Scheme 1), the condensation of aldehyde (A) and amine (B) to form the imine (C), followed by acid-induced ring closure via intermediate (D) to yield isoquinoline (E) (Li, Reference Li2009). The second step requires a high acid concentration (typically ~70% sulfuric acid) as well as a long reaction time, ranging from hours to days (Gensler, Reference Gensler2004). We prepared separately the imine (C), which was dissolved in methanol, and electrosprayed into a high-resolution mass spectrometer as depicted in Fig. 1a . In sharp contrast to the behavior in bulk solution, we found the production of isoquinoline from (C) in charged microdroplets (see Fig. 2), even though the average lifetime of the charged droplet is of the order of milliseconds and no acid has been added to induce the reaction. Moreover, we detected the intermediate (D), which was proposed earlier but no direct experimental evidence of its existence was presented. The acceleration of the reaction rate is estimated to be roughly more than a factor of a million, based on the yield and ionization efficiency of (E). This remarkable behavior of reactions in electrospray droplets appears to us to be highly promising for preparative scale synthesis of important isoquinoline-based organic compounds (e.g. fine chemicals) on a short timescale. Isoquinoline is a precursor material of many biologically active compounds such as anesthetics, antihypertension agent, antifungal agent, disinfectants, and many other drugs (Waldvogel, Reference Waldvogel2005).

Fig. 2. Pomeranz–Fritsch synthesis of isoquinoline in the charged droplet produced by electrospray process. The left panel shows the two step synthesis of isoquinoline that we followed in the present study. In the first step, the conventional bulk reaction method was used to synthesize the precursor imine C. Then in the second step, the precursor C was injected from methanolic solution through an on-axis electrospray source, in positive ion mode, to form charged droplets encapsulating the precursor C, which was then converted into isoquinoline (E) inside the charged droplet via intermediate D. Each protonated species (precursor C, intermediate D, and product E) were detected and characterized by a high-resolution orbitrap mass spectrometer (see the spectra in the right panel; solvent: methanol). The theoretical values of m/z (see left panel) are in good agreement with that experimentally observed (see the right panel).
We also have investigated the effects of droplet solvent composition (see Table 1) by monitoring the progress of the reaction of (C) in different solvents (of microdroplets). We measured the absolute intensities (counts) of the individual species (reactant, intermediate, and product). Our experimental data (Table 1) suggest that the reaction efficiency in microdroplets depends on cumulative effects of multiple properties of the droplet such as evaporation, charge accumulation, average lifetime, polarity of the droplet, etc. The maximum reaction progress was observed in the droplet produced from 1% m-NBA in water. The m-NBA is popularly known as a supercharging agent in the electrospray process (Iavarone & Williams, Reference Iavarone and Williams2003; Lomeli et al. Reference Lomeli, Peng, Yin, Loo and Loo2010; Sterling et al. Reference Sterling, Daly, Feld, Thoren, Kintzer, Krantz and Williams2010). It also enhances the average droplet lifetime because of its very low volatility (vapor pressure given in Table 2). Thus, the confinement of the reagent (C) in a highly charged, comparatively long-lived droplet might help the reaction to occur to a greater extent. On the contrary, when ACN–DMF mixture was used as the solvent, we observed the lowest reaction efficiency (see Table 1), although low volatile DMF (vapor pressure data given in Table 2) (Lide, Reference Lide1996) enhances the droplet lifetime. The possible reason of this low reaction efficiency in the presence of DMF might be caused by low surface-charge (protons) accumulation, which is largely guided by Rayleigh limit charging (dependence of surface charge density on the surface tension of the droplet; the surface tension data have been listed in Table 2) as given by Eq. (1) (Banerjee & Mazumdar, Reference Banerjee and Mazumdar2012; Kebarle & Tang, Reference Kebarle and Tang1993; Rayleigh, Reference Rayleigh1882).

where Z R is the charge limit, e is the elementary charge, R is the radius of the charged droplet, γ is the surface tension, and ε 0 is the permittivity of the surrounding medium.
Table 1. ESI-MS signal intensities of different protonated species (precursor C, intermediate D, and product E) obtained from different solvents a

a Data are highly reproducible and averaging of 1 min acquisition data is presented here. Signal intensities depend on concentration as well as ionization efficiency of the analyte.
b Mixture of ACN and DMF was used as DMF (low volatile) alone is not recommended for ESI.
c m-Nitrobenzyl alcohol (m-NBA).
Table 2. Physical properties of different electrospray solvents a

a Data taken from (Lide, Reference Lide1996).
b Data taken from (Banerjee, Reference Banerjee2013).
Likewise, the efficiency of product formation in methanol and water droplet is roughly similar under the present experimental conditions (see Table 1) possibly by the combined effects of vapor pressure and surface tension (see Table 2) as discussed above. However, a detailed study of possible effects of droplet solvent composition on the reaction rate enhancement needs to be undertaken at different instrumental conditions to extract more comprehensive information on the mechanism of reaction acceleration in the droplet compared with that in conventional bulk phase. Nevertheless, this preliminary observation of superfast reaction in the microdroplet is quite fascinating. It might open a new dimension in the field of synthetic organic chemistry, where water would be used as an environmentally benign solvent (green chemistry).
Reaction kinetics of cytochrome c and maltose binding in microdroplets
Protein–sugar interaction are known to play important roles for cell–cell binding, cell–matrix interaction, migration of tumor cells, recognition of pathogens, and energy transport (Holgersson et al. Reference Holgersson, Gustafsson and Breimer2005; Quiocho, Reference Quiocho1986; Rauvala et al. Reference Rauvala, Carter and Hakomori1981; Rudd et al. Reference Rudd, Wormald and Dwek2004). However, the kinetics of the sugar–protein interaction is not well understood. Here we investigated the kinetics of cytochrome c and maltose complexation since it was reported that the maltose possesses several hydroxyl groups noncovalently bound to cytochrome c though hydrogen bonding (Liu et al. Reference Liu, Su and Wang2012). In this report, we also show reaction rate acceleration on fusing together two aqueous electrospray droplets (see Fig. 1b ), one containing cytochrome c and the other containing maltose, to form hydrogen-bonded noncovalent complexes in the fused droplet. Here we have used our previously developed droplet fusion apparatus (Lee et al. Reference Lee, Kim, Nam and Zare2015) for investigating kinetics of this protein–ligand interaction. Figure 3 shows the conventional ESI-mass spectra of pure cytochrome c (upper panel) and cytochrome c premixed with maltose (lower panel). A distribution of multiply charged species comprising cytochrome c and different numbers of maltose via noncovalent hydrogen bonding interaction was detected when we electrosprayed the mixture of cytochrome c and maltose (see Fig. 3b ). For kinetics analyses, two different droplets containing cytochrome c at 100 μM and maltose at 100 mM were fused while the distance x (from droplet fusion center to the heated capillary inlet of the mass spectrometer) was varied. A 1000-fold excess concentration of cytochrome c over maltose was used here to ensure binding of maltose to cytochrome c in the fused droplet that is travelling the distance x in very short timescale (tens of microseconds). Figure 4 shows the measured kinetics of cytochrome c–maltose binding. As the distance x increased from 0·7 to 3·875 mm in increments of 0·6 mm, the ion signal intensities corresponding to cytochrome c bound with higher numbers of maltose increased. The deconvoluted mass spectra at x = 3·875 mm (see Fig. 4a ) indicated a maximum of 25 ligands (maltose) bound to cytochrome c. The signal intensity corresponding to cytochrome c with no maltose binding reached its maximum at x = 0·7 mm under the present experimental conditions, followed by a gradual decay over distance x. The ion signals corresponding to cytochrome c bound to 6, 11, and 18 maltose molecules reached their maxima at x = 1·335, 2·605, and 3·24 mm, respectively (see Fig. 4b ), indicating the gradual occupation of maltose to available binding sites in cytochrome c. The average number of bound maltose molecules reached a plateau at 2·605 mm, corresponding to 35·2 μs in time (see Fig. 4c ). The estimated association time constant for cytochrome c and maltose interaction was found to be 17·9 ± 8·6 μs in the present study. The reported time constant for protein–sugar binding in bulk solvent is of the order of 10–100 ms (Miller et al. Reference Miller, Olson, Pflugrath and Quiocho1983). Therefore, this noncovalent reaction in the droplet has been accelerated by a factor of a thousand or more compared to that in bulk solution. It should be noted that this detailed information on the number and distribution of bound ligands to a protein demonstrates the power of mass spectrometric measurement of the protein–ligand interaction at a short timescale which is not readily available by population-averaged spectroscopic methods.

Fig. 3. ESI-mass spectra of (a) cytochrome c (100 μM) and (b) cytochrome c (100 μM) incubated with maltose (100 mM) for 20 min. The subscript n in PLn denotes the number of bound maltose to cytochrome c (square denotes +8 charge state and circle denotes +7 charge state).

Fig. 4. Kinetics of the binding of cytochrome c and maltose. (a) Deconvoluted mass spectra at different distances (x) with cytochrome c (100 μM) in one droplet source and maltose (100 mM) in the other source. The subscript n in PLn denotes the number of maltose bound to cytochrome c. (b) Normalized relative abundances of cytochrome c with different number of bound maltose (green square: PL1, red circle: PL6, blue triangle up: PL11, magenta triangle down: PL18). The normalized factor for each plot for PL1, PL6, PL11, and PL18 is ×1, ×4·7, ×11·7, and ×17·4, respectively. (c) Average number of bound maltose to cytochrome c as a function of distance x and reaction time. The axes on top of (a) and (c) show the converted reaction time from the corresponding distance.
To investigate the origin of acceleration of these noncovalent reactions in aqueous microdroplets, we have measured the sizes of fused droplets composed of pure water traveling from droplet fusion center to mass spectrometer inlet (see Fig. 1b ) with a high-speed camera (see Fig. 5). We have not observed any significant decrease of the droplet size until the distance reaches to x = 7 mm. It needs to be emphasized that all kinetic measurements we make are performed for x = 0 to 4 mm. We do have large error bars on the measured droplet diameter, and the volume of the droplet varies as the cube of the diameter. Even if we imagine that the droplet diameter shrunk in size from 13 to 11 μm, this reduction corresponds to a volume change of less than 50%, which would cause the overall concentrations of reagents to increase by less than a factor of two. We believe that this concentration increase could contribute to but could not account for the marked enhancement in the reaction rate compared to bulk solution we have observed. Hence, we are led to conclude that confinement of reagents (cytochrome c and maltose) in a small volume might be the chief cause for reaction rate enhancement.

Fig. 5. Average diameter of pure-water droplets in the microdroplet fusion mass spectrometry as a function of the distance (x). Few noticeable differences were observed in the average size of microdroplets up to the distance of about 7 mm from the droplet fusion center. All kinetic measurements shown in Fig. 4 are performed at distances of 4 mm or less.
Conclusion
We observe a remarkable acceleration in the reaction rate in microdroplets produced by electrospray and when two droplets containing different reagents are fused together. These findings are elevating our interest to conduct important reactions in liquid droplets (aerosols), which would provide a small confined volume for reagents to react in a faster rate than that in conventional bulk solution. The acceleration of the chemical reaction in microdroplets appears to be general regardless of reaction mechanisms, including specific covalent and nonspecific noncovalent bonding. What causes the reaction acceleration in the microdroplet remains yet to be fully understood. The previous studies (Badu-Tawiah et al. Reference Badu-Tawiah, Campbell and Cooks2012; Bain et al. Reference Bain, Pulliam and Cooks2015; Girod et al. Reference Girod, Moyano, Campbell and Cooks2011) emphasized that solvent evaporation of microdroplets plays an important role in accelerating the reaction. However, nearly constant droplet size in our droplet fusion mass spectrometric study suggests that the evaporation process may not be the only factor in accelerating the reaction. It is apparent that the reaction in a confined environment can occur in a different manner to that in a bulk environment. The chemistry at air–droplet surface interface (Griffith & Vaida, Reference Griffith and Vaida2012; Jung & Marcus, Reference Jung and Marcus2007; Narayan et al. Reference Narayan, Muldoon, Finn, Fokin, Kolb and Sharpless2005, Reference Narayan, Ramaswamy and Menon2007) may play a special role in the reaction acceleration. However, a more detailed study is needed to investigate the possible mechanisms that bring about the acceleration of reaction in microdroplets.
‘Microdroplet chemistry’ is still in its infancy and raises our interest to explore this unknown territory. The aerosol (aqueous droplet) was suggested to be a plausible origin of life (Dobson et al. Reference Dobson, Ellison, Tuck and Vaida2000; Griffith & Vaida, Reference Griffith and Vaida2012). Indeed, the reactions of life systems mostly occur in confined microdroplet-like environments such as cells and cellular organelles. It is well known that macromolecular crowding alters the properties of molecules in a solution when high concentrations of macromolecules such as proteins are present (Ellis, Reference Ellis2001; Zhou et al. Reference Zhou, Rivas and Minton2008). We envision that microdroplet chemistry may lead to a better understanding of the chemistry in confined environments, which may be relevant to biochemical processes in a cell.
Acknowledgements
This work was supported by the Air Force Office of Scientific Research through Basic Research Initiative grant (AFOSR FA9550-12-1-0400); National Institute of Health (NIH 1R21DA039578-01); National Science Foundation under the CCI Center for Selective C-H Functionalization (CHE-1205646); and the Institute for Basic Science (IBS-R013-D1).