Introduction
The first mention of wöhlerite in the literature was made by Scheerer (Reference Scheerer1843) who studied the mineralogical paragenesis of the syenite pegmatites occurring on Løvøya island, Brevig area, Langesundsfjord, Norway. The chemical analysis performed by Scheerer (Reference Scheerer1843) indicated that wöhlerite is a silicate containing mainly Na, Ca, Zr and Nb, as well as minor amounts of Mg, Mn and Fe. At the time of wöhlerite's discovery niobium was not officially approved as a distinct chemical element, and consequently Scheerer (Reference Scheerer1843) had erroneously reported niobium in wöhlerite as tantalum. Among the wöhlerite-group minerals, cuspidine was the first to have its crystal structure solved (Smirnova et al., Reference Smirnova R.F., Rumanova. and Belov.1955) and the wöhlerite group is sometimes mentioned as the cuspidine group in the literature (e.g. Merlino and Perchiazzi, Reference Merlino and Perchiazzi1988; Chakhmouradian et al., Reference Chakhmouradian, Mitchell, Burns, Mikhailova and Reguir2008). However, as wöhlerite is the first described species of the group the name should be wöhlerite group in accordance with Mills et al. (Reference Mills, Hatert, Nickel and Ferraris2009). Merlino and Perchiazzi (Reference Merlino and Perchiazzi1988) demonstrated that the nature of the crystal structure of the wöhlerite-group minerals (WGM) permits the crystallisation in different unit-cell settings and the formation of polytypes. In addition, they identified ten different structure-types that are possible within the fixed cell dimension a ≈ b ≈ 10.5 Å and c ≈ 7.3 Å. The WGM can form multi-domain crystals, as for instance in ‘guarinite’ from Monte Somma, Italy (Bellezza et al., Reference Bellezza, Merlino and Perchiazzi2012).
The new definition of the wöhlerite group has been approved by the Commission on New Minerals, Nomenclature and Classification (CNMNC) of the International Mineralogical Association (IMA) (Proposal 20-D; Miyawaki et al., Reference Miyawaki, Hatert, Pasero and Mills2020). The wöhlerite group includes mineral species that have the general formula X 8(Si2O7)2W 4 (Table 1), where X represents the cationic sites typically occupied by Na+, Ca2+, Mn2+, Fe2+, Ti4+, Zr4+ and Nb5+; and where W represents anionic sites with F–, (OH)– and O2–, which are not bonded to the silicate tetrahedra. The X sites have the same general topology and consequently a specific chemical element will have a different X site preference in different WGM species. The general formula proposed for WGM is similar to that of the rinkite group (seidozerite supergroup) minerals (Sokolova and Cámara, Reference Sokolova and Cámara2017), however rinkite- and wöhlerite-group minerals have different structures. The crystal structure of WGM is characterised by four-columns-wide octahedral walls, which interconnect through corner sharing and via the disilicate groups to create a framework (Fig. 1). The cationic ordering in the walls and the relative position of the disilicate groups lead to different symmetries (monoclinic and triclinic). The crystal structure of the borate minerals warwickite and yuanfuliite show the same type of framework, with isolated triangular BO3 groups replacing the disilicate groups (Bigi et al., Reference Bigi, Brigatti and Capedri1991; Appel et al., Reference Appel, Bigi and Brigatti1999).

Fig. 1. General view of the structure of the wöhlerite-group minerals. The polyhedra of the octahedral walls are in grey, Si and O atoms are in blue and red, respectively.
Table 1. List of minerals belonging to the wöhlerite group.

S.G. – space group. *see Merlino and Perchiazzi (Reference Merlino and Perchiazzi1988).
References: [1] Saburi et al. (Reference Saburi, Kawahara, Henmi, Kusachi and Kihara1977); [2] Mellini (Reference Mellini1981); [3] Perchiazzi et al. (Reference Perchiazzi, McDonald, Gault, Johnsen and Merlino2000); [4] Mellini (Reference Mellini1982); [5] Annehed et al. (Reference Annehed, Falth and Raade1985); [6] Mellini and Merlino (Reference Mellini and Merlino1979); [7] Merlino et al. (Reference Merlino, Perchiazzi, Khomyakov, Pushcharovsky, Kulikova and Kuzmin1990); [8] Biagioni et al. (Reference Biagioni, Bonaccorsi, Perchiazzi and Merlino2010); [9] Merlino and Perchiazzi (Reference Merlino and Perchiazzi1985); [10] this work; [11] Qu et al. (Reference Qu, Dong, Li, Fan, Wang, Cheng, Jin, Sun, Zhao and Wang2020); [12] Chakhmouradian et al. (Reference Chakhmouradian, Mitchell, Burns, Mikhailova and Reguir2008).
Historical synopsis
Cuspidine
Cuspidine, ideally Ca8(Si2O7)2F4 (Z = 2), was described by Scacchi (Reference Scacchi1876) from Monte Somma, Somma–Vesuvius complex, Italy. Cuspidine occurs in different geological environments such as skarns (Tilley, Reference Tilley1947; Taner et al., Reference Taner, Martin and Gault2013), tuff ejecta (Federico and Peccerillo, Reference Federico and Peccerillo2002), pegmatitoid facies of venanzite (Bellezza et al., Reference Bellezza, Merlino and Perchiazzi2004a), calc-silicate xenoliths (Owens and Kremser, Reference Owens and Kremser2010), natrocarbonatite (Mitchell and Belton, Reference Mitchell and Belton2004) and alkaline rocks (Andreeva et al., Reference Andreeva, Kovalenko, Nikiforov and Kononkova2007).
Cuspidine is monoclinic, P21/a, with a = 10.906, b = 10.521, c = 7.518 Å and β = 109.90°. The first structural investigation was provided by Smirnova et al. (Reference Smirnova R.F., Rumanova. and Belov.1955) who considered the structure as an array of chains of edge-sharing Ca(O,F)6 octahedra running parallel to the c axis, by analogy to the structure of ilvaite, epidote and tilleyite. Subsequent refinement of the cuspidine structure by Saburi et al. (Reference Saburi, Kawahara, Henmi, Kusachi and Kihara1977) concluded that the coordination environments of the Ca sites are not solely octahedral but vary between six-, seven- and eight-fold. There are four Ca sites in total, with an average bond distance of 2.367, 2.404, 2.428 and 2.449 Å. In each column of the wall, the sites alternate between being small or large. Bellezza et al. (Reference Bellezza, Merlino and Perchiazzi2004a) reported the incorporation of up to 0.22 Zr atoms per formula unit (apfu) and 0.32 Na apfu in cuspidine, following the substitution mechanism 2Ca2+ + F– ↔ Na+ + Zr4+ + O2–. According to their structural model, Zr is incorporated on the small octahedral site lying in the outer columns of the wall (X1), whereas Na is incorporated on the large site (X3) lying in internal columns and connected by edge-sharing to the Zr-bearing octahedra (Fig. 2). Note that Taner et al. (Reference Taner, Martin and Gault2013) reported cuspidine from the Güneyce–Ikizdere Region in Turkey, with an unusually low F content (1.36 apfu), which may correspond to a hydroxide equivalent. Finally, Krzątała et al. (Reference Krzątała, Galuskin, Galuskina and Vapnik2018) reported from the Hatrurim complex, Israel, a ‘uranian cuspidine’ containing up to 0.64 U apfu, and only 0.98 F apfu. The oxidation state of uranium remains uncertain, as well as the exact insertion mechanism. Although, considering the similar ionic radii of Ca2+ and U4+ (U5+) in octahedral coordination, the occurrence of uranium-bearing cuspidine is plausible.
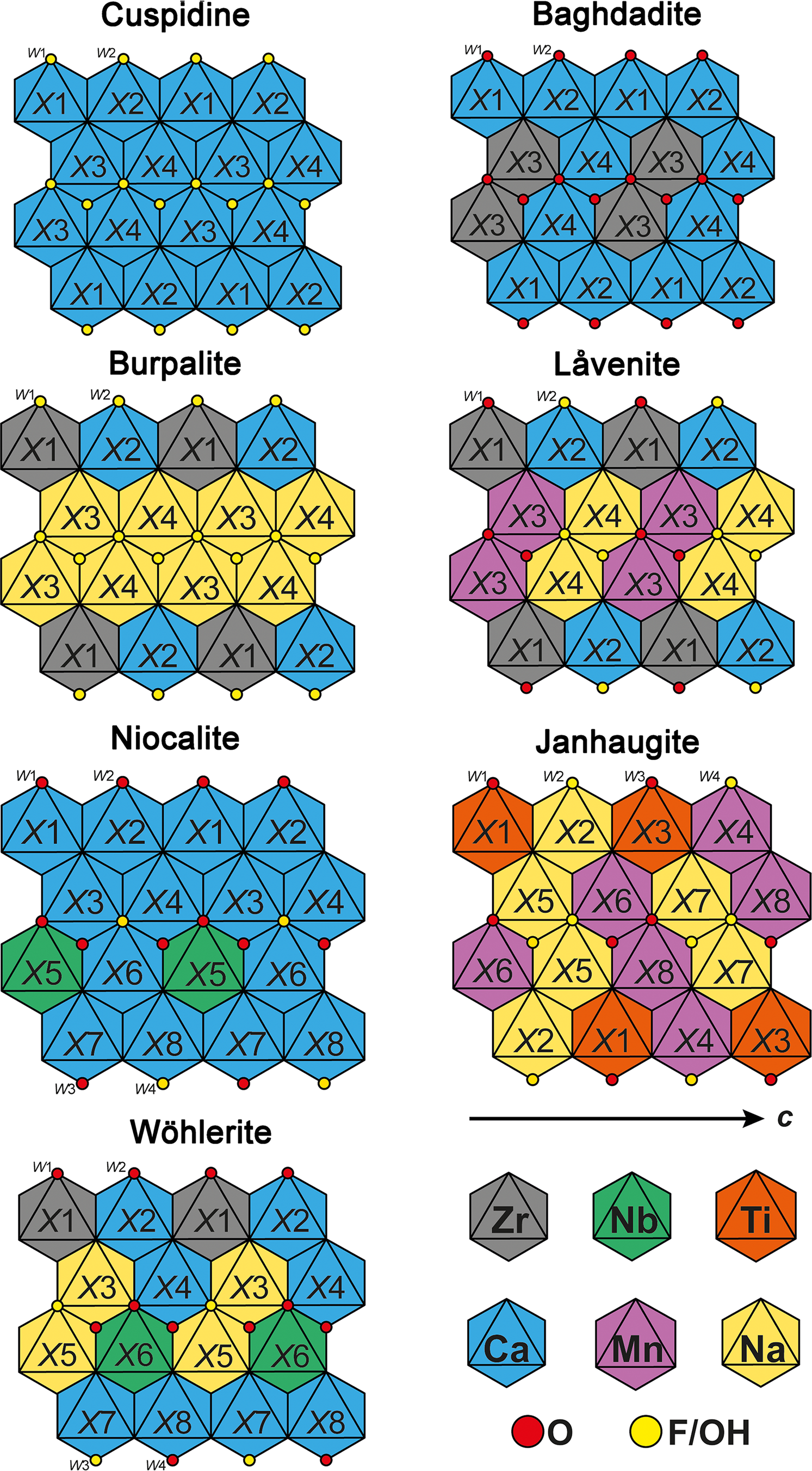
Fig. 2. Schematic and idealised representation of the cationic distribution in the walls of cuspidine, baghdadite, burpalite, låvenite, niocalite, janhaugite and wöhlerite (and marianoite, see text). Normandite has the same cationic distribution as låvenite with X1 = Ti.
Låvenite and normandite
Låvenite, ideally Na2Ca2Mn2Zr2(Si2O7)2O2F2, was described by Brøgger (Reference Brøgger1884) from nepheline syenite pegmatites occurring on the Låven island, Langesundsfjord area, Norway. Låvenite is now reported from many alkaline localities around the world: Igaliku complex, Greenland (Jones and Larsen, Reference Jones and Larsen1985; Friis et al., Reference Friis, Balić Žunić, Williams and Petersen2010); Los Archipelago, Guinea (Biagioni et al., Reference Biagioni, Merlino, Parodi and Perchiazzi2012); Cerro Boggiani complex, Paraguay (Comin-Chiaramonti et al., Reference Comin-Chiaramonti, Renzulli, Ridolfi, Enrich, Gomes, De Min, Azzone and Ruberti2016); Itatiaia complex, Brazil (Melluso et al., Reference Melluso, Guarino, Lustrino, Morra and De’ Gennaro2017); and Burpala massif, Russia (Vladykin and Sotnikova, Reference Vladykin and Sotnikova2017).
The crystal structure of låvenite was refined on samples from the Lovozero alkaline massif, Russia (Simonov and Belov, Reference Simonov and Belov.1960), Langesundsfjord, Norway (Mellini, Reference Mellini1981) and Los Archipelagos, Guinea (Biagioni et al., Reference Biagioni, Merlino, Parodi and Perchiazzi2012). Låvenite is monoclinic, P21/a, with a = 10.83, b = 9.98, c = 7.17 Å and β = 108.1°. These structural data, as well as the chemical data published elsewhere (see the references listed above) indicate clearly the occurrence of cationic substitution on the four X sites (Fig. 2). The larger X2 and X4 sites are dominated by Ca and Na, respectively. Note that X2 usually contains a high amount of Na leading to the mix occupancy close to Ca0.60Na0.40. The smallest X1 site is dominated by Zr, and the main substitution observed is Zr4+ ↔ Ti4+. The last site, X3, has an intermediate size (≈ 2.23 Å) and is occupied by a mix of Ca, Fe, Mn and Zr. In låvenite, Mn2+ is dominant on X3 though the high Ca contents reported in some samples may indicate that Ca could also be dominant, thus leading to a new end-member composition (Na2Ca4Zr2(Si2O7)2O2F2). The W2 site, bonded to the X3 and X4 sites, is fully occupied by F–, while the W1 site, bonded to the X1, X3 and X4 sites, is populated by O2– which is partially substituted by F–.
Normandite, ideally Na2Ca2Mn2Ti2(Si2O7)2O2F2, is the titanium analogue of låvenite described initially from the Poudrette quarry, Mont Saint-Hilaire, Quebec, Canada (Chao and Gault, Reference Chao and Gault1997). Note that a mineral with similar physical properties and composition had been reported prior to the normandite description from the Khibiny massif and Lovozero massif, Russia (Vlasov, Reference Vlasov1966); Tenerife, Canary Islands (Ferguson, Reference Ferguson1978) and Tamazeght, Morocco (Khadem Allah, Reference Allah B.1993). Perchiazzi et al. (Reference Perchiazzi, McDonald, Gault, Johnsen and Merlino2000) refined the crystal structure of normandite from the Poudrette quarry and Amdrup Fjord, Greenland. Normandite is monoclinic, P21/a, with a = 10.799, b = 9.801, c = 7.054 Å and β = 108.08°. The normandite structure confirmed the structural model and the cation distribution established in låvenite. Perchiazzi et al. (Reference Perchiazzi, McDonald, Gault, Johnsen and Merlino2000) also noted that their samples had an excess of Na and Ca with respect to the expected value of 4 apfu in total, and also had an excess of high-charge cations (Zr and Ti). At the same time, the sum of cations (Mn, Fe and Mg) located on the X3 site is significantly below 2 apfu, suggesting that the excess of Ca and Zr is hosted on the X3 site with an average bond distance of ≈2.20 Å (Fig. 2).
Baghdadite
Baghdadite, Ca6Zr2(Si2O7)2O4, is the only wöhlerite-group mineral without any F– or OH- groups. First reported from melilite skarn in contact with diorite, Qala–Dizeh region, Iraq (Hermezi et al., Reference Hermezi, McKie and Hall1986), it has since been described from skarns, calc-silicate marbles and hornfels (Jamtveit et al., Reference Jamtveit, Dahlgren and Austrheim1997; Matsubara and Miyawaki, Reference Matsubara and Miyawaki1999; Shiraga et al., Reference Shiraga, Kusachi, Kobayashi and Yamakawa2001; Galuskin et al., Reference Galuskin, Pertsev, Armbruster, Kadiyski, Zadov, Galuskina, Dzierzanowski, Wrzalik and Kislov2007; Galuskina et al., Reference Gałuskina, Gałuskin, Lazic, Armbruster, Dzierżanowski, Prusik and Wrzalik2010). The main chemical substitution occurring in baghdadite is the homovalent substitution Zr4+ ↔ Ti4+.
Baghdadite is monoclinic, P21/a, with a = 10.432, b = 10.163, c = 7.356 Å and β = 90.96° (Biagioni et al., Reference Biagioni, Bonaccorsi, Perchiazzi and Merlino2010). In addition to its chemical composition, the crystal structure of baghdadite is also unique for WGM as it shows the edge-sharing of two ZrO6 octahedra in the internal columns of the wall (Biagioni et al., Reference Biagioni, Bonaccorsi, Perchiazzi and Merlino2010) (Fig. 2). This structural feature is at odds with the Pauling's fourth rule, which states that high-valence cations tend to not share polyhedron elements (Pauling, Reference Pauling1929). In all other WGM the high-valence cations (Y3+, Ti4+, Zr4+and Nb5+) do not share any ligands.
Burpalite
Burpalite, Na4Ca2Zr2(Si2O7)2F4, was found for the first time within a fenitised sandstone in the contact zone of the Burpalinskii alkaline massif, North Transbaikal, Russia (Merlino et al., Reference Merlino, Perchiazzi, Khomyakov, Pushcharovsky, Kulikova and Kuzmin1990). It is reported in only a few other localities around the world: Umbozero mine, Lovozero massif, Russia; Vesle Arøya, Langesundsfjorden, Norway; and Nanna pegmatite, Igaliku, Greenland (Friis et al., Reference Friis, Balić Žunić, Williams and Petersen2010). Chemical data on burpalite are scarce and the published data on the type material indicates a composition close to the end-member formula.
Burpalite is monoclinic, pseudo-orthorhombic, P21/a, with a = 10.117, b = 10.445, c = 7.255 Å and β = 90.04° (Merlino et al., Reference Merlino, Perchiazzi, Khomyakov, Pushcharovsky, Kulikova and Kuzmin1990). Site occupancies indicate that the X1 and X2 sites are fully occupied by Zr and Ca, respectively. The larger X3 and X4 sites are mainly populated by Na with minor substitutions of Ca. Bond valence analysis confirms the presence of only F on the W2 site, and the replacement of a small amount of F by O on the W1 site bonded to the Zr polyhedron (Fig. 2).
Structural investigations performed by Merlino et al. (Reference Merlino, Perchiazzi, Khomyakov, Pushcharovsky, Kulikova and Kuzmin1990) also indicate that some crystals of burpalite contain domains with a låvenite-type structure. Burpalite- and låvenite-type structures are related, as they are two distinct ordered members in a family of order–disorder structures. In addition, a so-called ‘orthorhombic låvenite’ was reported from the Burpalinskii massif (Portnov et al., Reference Portnov, Simonov and Sinyugina1966; Portnov and Sidorenko, Reference Portnov and Sidorenko1975). This mineral has the same chemical composition as burpalite, but with a B-centred orthorhombic cell, a = 21.01, b = 10.05 and c = 7.23 Å, and is polysynthetically twinned on (100). That unit-cell however, can be transformed to monoclinic, a = 11.11, b = 10.05, c = 7.23 Å and β = 108.99°, twinned on (100), analogous to the unit-cell of låvenite. Consequently ‘orthorhombic låvenite’ is a polytype of burpalite (burpalite-1M 2), corresponding to a twinned maximum degree of order (MDO) polytype.
Merlino et al. (Reference Merlino, Perchiazzi, Khomyakov, Pushcharovsky, Kulikova and Kuzmin1990) suggested that isotypic series may occur between burpalite and baghdadite through the substitution Na+ + F– ↔ Ca2+ + O2–. However, a burpalite–baghdadite series is unlikely because in burpalite the ZrO6 octahedra are lying in the outer columns of the walls, while in baghdadite they are in the central columns (Fig. 2). Therefore, a transformation of burpalite into baghdadite requires a complete reordering of the cationic octahedral sites.
Niocalite
Niocalite, ideally Ca7Nb(Si2O7)2O3F, is a rare niobium silicate reported from the Oka complex, Laurentides, Canada (Nickel, Reference Nickel1956), the Badloch quarry, Baden-Württemberg, Germany (Keller and Williams, Reference Keller and Williams1995) and the Ol Doinyo Lengai, Arusha region, Tanzania (Mitchell and Belton, Reference Mitchell and Belton2004; Reusser, Reference Reusser2010).
Niocalite crystals typically show polysynthetic or contact twinning and therefore the determination of the correct space group was not straightforward. Nickel et al. (Reference Nickel, Rowland and Mawwell1958) reports the unit-cell parameters a = 10.83, b = 10.42, c = 7.38 Å and β = 109.40°, and the probable space group Pa or P2/a. They showed that the twinned crystals have a pseudo-orthorhombic symmetry that is achieved through the twin plane ($\bar{1}$02). Li et al. (Reference Li, Simonov and Belov1966) conducted a structural investigation and proposed a model in P21, though without taking the twinning into account. The presence of Si2O7 groups ambiguously connected to the short edge of the NbO6 octahedra in the Li et al. model prompted Mellini (Reference Mellini1982) to reinvestigate the crystal structure of niocalite using the space group Pa. Mellini (Reference Mellini1982) interpreted the diffraction pattern from twinned niocalite as the result of a twinning on the (100) compositional plane. The same author confirmed the presence of microstructural domains in niocalite through transmission electron microscopy investigations. Although, the structure refinement seems to indicate a disordered distribution of Ca and Nb within two different crystallographic sites (X4 and X6), it was concluded by Mellini (Reference Mellini1982) that Nb and Ca are ordered on their respective sites (Fig. 2). The apparent disorder is the result of the averaging of the intensity data from the two twin-related domains, in which the X4 and X6 site positions are mutually inverted.
Note that the chemical analysis shows the presence of approximately two F apfu in niocalite, while the end-member formula has only one F apfu. The bond valence analysis shows that only the W3 site, which is shared solely by Ca polyhedra, is entirely populated by F. The remaining F content is distributed randomly on the other W sites. The incorporation of F on the W sites results from the charge balance mechanism linked to the Ca2+ ↔ Na+ substitution, and to the partial replacement of Nb5+ by Ti4+ and Zr4+.
Wöhlerite
Wöhlerite, ideally Na2Ca4ZrNb(Si2O7)2O3F, was reported initially by Scheerer (Reference Scheerer1843) from the syenite pegmatites occurring on Løvøya island, Brevig area, Langesundsfjord, Norway. Wöhlerite is an abundant mineral throughout the Langesundsfjord (Andersen et al., Reference Andersen, Erambert, Larsen and Selbekk2010; Larsen, Reference Larsen2010; Sunde et al., Reference Sunde, Friis and Andersen2018), though also from other syenites and carbonatites around the world (e.g. Mariano and Roeder, Reference Mariano and Roeder1989; Keller and Williams, Reference Keller and Williams1995; Bellezza et al., Reference Bellezza, Merlino and Perchiazzi2012; Biagioni et al., Reference Biagioni, Merlino, Parodi and Perchiazzi2012; Melluso et al., Reference Melluso, Guarino, Lustrino, Morra and De’ Gennaro2017; Guarino et al., Reference Guarino, De’ Gennaro, Melluso, Ruberti and Azzone2019).
Wöhlerite is monoclinic, P21, with a = 10.823, b = 10.244, c = 7.290 Å and β = 109.00° (Mellini and Merlino, Reference Mellini and Merlino1979). Shibayeva and Belov (Reference Shibayeva1962) and Golyshev et al. (Reference Golyshev, Otroshchenko, Simonov and Belov1973) performed the first structure refinements on wöhlerite and showed the presence of four-columns-large octahedral walls interconnected by corner sharing and Si2O7 diorthosilicate groups. Mellini and Merlino (Reference Mellini and Merlino1979) provided a structure refinement of wöhlerite from Brevig, Norway, confirming the space group and showing that the structure is based on four independent Si sites, four Ca sites, two Na sites, one Zr site and one Nb site (Fig. 2). The bond-valence analysis indicates that only one anionic site is dominated by a monovalent anion. Biagioni et al. (Reference Biagioni, Merlino, Parodi and Perchiazzi2012) have refined the crystal structure of wöhlerite from Los Archipelagos, Guinea, which contains more Mn and F, and less Nb than the Norwegian material.
Chemical data provided by Mariano and Roeder (Reference Mariano and Roeder1989) on wöhlerite from different localities indicate that the chemical composition of wöhlerite is relatively consistent, and they note that the largest variations are observed for the Nb, Ti and F contents. According to the structural model they establish the coupled substitution Nb5+ + O2– ↔ Ti4+ + F–. However, the Ti increase is only half of the decrease of the Nb content, thus indicating a replacement of Nb by another chemical element. This is confirmed by subsequent crystal structure refinements in which the Nb site is populated by a significant amount of Mn or Mg (Bellezza et al., Reference Bellezza, Merlino and Perchiazzi2012; Biagioni et al., Reference Biagioni, Merlino, Parodi and Perchiazzi2012). Andersen et al. (Reference Andersen, Erambert, Larsen and Selbekk2010) and Sunde et al. (Reference Sunde, Friis and Andersen2018) showed only minor chemical variations in wöhlerite from different localities in the Larvik plutonic complex in Norway.
Janhaugite
Described from a sodium-rich alkali feldspar granite (ekerite) at Gjerdingen, Oslo region, Norway (Raade and Mladeck, Reference Raade and Mladeck1983), janhaugite, ideally Na3Mn3Ti2(Si2O7)2(OH)2OF, is an extremely rare mineral displaying some unique chemical features among the WGM. The Mn content in janhaugite (up to 2.4 apfu) is the highest recorded for any WGM. Electron microprobe analytical (EMPA) data show the presence of roughly one F apfu. Infrared spectroscopy confirmed the presence of OH groups, and the splitting of the O–H stretching frequencies (3550, 3510 and 3460 cm–1) may indicate that OH groups are distributed on three different crystallographic sites (Raade and Mladeck, Reference Raade and Mladeck1983).
Janhaugite is monoclinic, P21/n, with a = 10.668, b = 9.787, c = 13.931 Å and β = 107.82° (Annehed et al., Reference Annehed, Falth and Raade1985). The refinement of the crystal structure, coupled with the bond-valence analysis, indicate that only two W sites (W2 and W4) are populated mainly by monovalent anions (Fig. 2). While the sum of (OH + F) should be equal to three to keep the electroneutrality of the mineral, one can assume that the remaining monovalent anions are distributed randomly on the W1 and W3 sites. Considering the local environment of the W1–W4 sites, W2 and W4 are tri-coordinated, wheras W1 and W3 are four-coordinated, and it is most likely that the OH groups occupy the W2–W4 sites. In addition, there are H acceptors in the vicinity of W2–W4 (≈2.9 Å). Therefore, the end-member formula Na3Mn3Ti2(Si2O7)2(OH)2OF is proposed for janhaugite, in order to show the ionic distributions on both the X and W sites, and to follow the rules defined for end-member formula by Hawthorne (Reference Hawthorne2021).
Hiortdahlite
Hiortdahlite, Na2Ca4(Ca0.5Zr0.5)Zr(Si2O7)2OF3, one of the oldest WGM known, was described from the Langodden pegmatite occurring in the nepheline–syenite of Langesundsfjord, Norway (Brøgger, Reference Brøgger1890a, Reference Brøgger1890b). Hiortdahlite is observed in several localities across the world and has been studied from the Korgeredaba alkaline Massif, Tuva, Russia (Kapustin and Bykova, Reference Kapustin and Bykova1965); Jingera, Australia (Eggleton et al., Reference Eggleton, Halford and Beams1979) and Los Archipelago, Guinea (Biagioni et al., Reference Biagioni, Merlino, Parodi and Perchiazzi2012).
Hiortdahlite is triclinic, P $\bar{1}$, a = 11.015, b = 10.941, c = 7.353 Å, α = 109.35, β = 109.88 and γ = 83.43° (Merlino and Perchiazzi, Reference Merlino and Perchiazzi1985). In addition, in their work on the multi-domains phase called ‘guarinite’ from Monte Somma, Italy, Bellezza et al. (Reference Bellezza, Merlino and Perchiazzi2012) reported one domain isostructural with hiortdahlite (domain IV). In the first structural investigation of hiortdahlite, Merlino and Perchiazzi (Reference Merlino and Perchiazzi1985) identified three crystallographic sites [M (X7), NaCa (X8), and F3 (W2)] where chemical substitutions occur. The X8 and W2 sites show a mixed occupancy of (Na0.60Ca0.40) and (F0.60O0.40), respectively (Fig. 3). The cationic substitutions occurring on the X7 site are complex, with a broad range of cations that may be incorporated (Fe2+, Mn2+, Ca2+ and Zr4+).
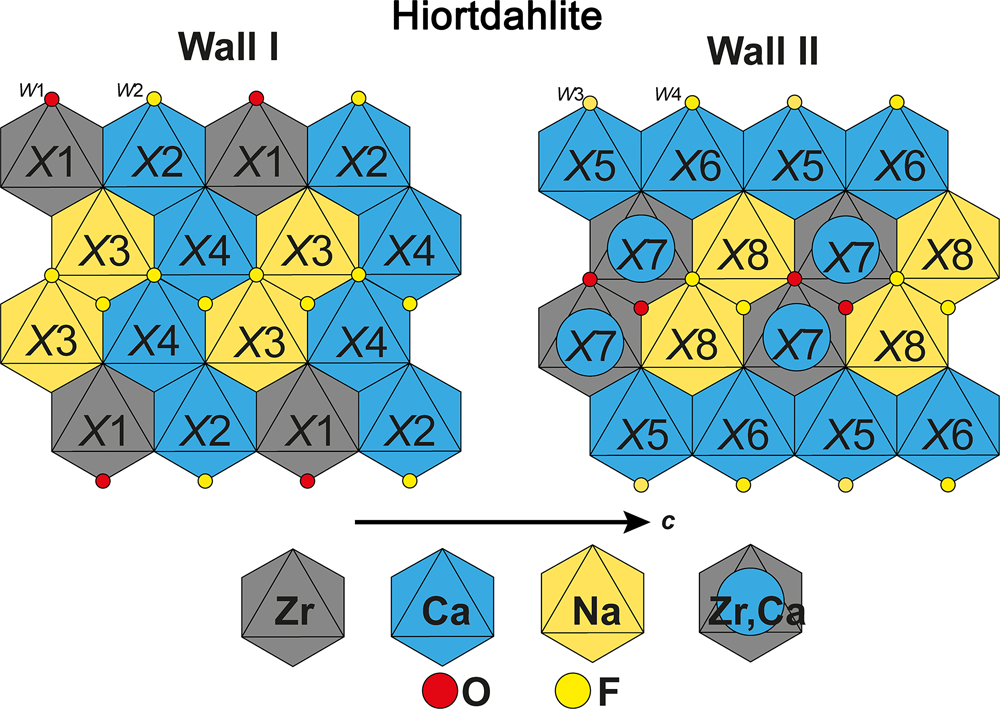
Fig. 3. Schematic and idealised representation of the cationic distribution in the walls of hiortdahlite.
In their model, Merlino and Perchiazzi (Reference Merlino and Perchiazzi1985), showed that the average charge of the X7 site is +3, and fixed the X7 site population to (Zr0.33Ti.16Ca0.16Mn0.16Fe0.16)Σ3+ according to the chemical data of Brøgger (Reference Brøgger1890a). Andersen et al. (Reference Andersen, Erambert, Larsen and Selbekk2010) have reanalysed a crystal fragment of the holotype sample (TYHIO sample, NRM no. 530976), and gave the formula Na1.74Ca4.60Mn0.10Fe0.10Y0.03Ce0.01Zr1.16Nb0.11Ti0.09Hf0.02(Si2O7)2O0.6F3.4, which is comparable to the chemistry of Brøgger (Reference Brøgger1890a), Na1.55Ca4.41Mn0.11Fe0.10REE0.03Ce0.01Zr1.32Ti0.14(Si2O7)2(OH)0.5O0.98F2.33 (with REE defining lanthanides). New crystallographic and chemical investigations have been performed on samples from the type locality, in order to determine accurately the cationic distribution in the crystal structure. These new data are presented below.
Moxuanxueite
Moxuanxueite, ideally NaCa6Zr(Si2O7)2OF3, was described recently from the alkaline syenites of the Gejiu intrusion in Honghe Hani and Yi, Autonomous Prefecture, Yunnan Province, China (Qu et al., Reference Qu, Dong, Li, Fan, Wang, Cheng, Jin, Sun, Zhao and Wang2020). The mineral is triclinic, P $\bar{1}$, a = 10.953, b = 10.929, c = 7.359 Å, α = 109.41, β = 109.89 and γ = 83.42°. Moxuanxueite is isostructural with hiortdahlite, with the X7 and X8 sites fully occupied by Ca (Table 2).
Table 2. Structural formula for the minerals of the wöhlerite group.

*X1–X4 and X5–X8 sites are part of the first and second wall, respectively. See references from Table 1 for the other minerals.
Chemical compositions of WGM and related species
Chemical classification of WGM
The members of the wöhlerite group show a range of compositions with the main cations being Na, Ca, Fe, Mn, Zr, Ti and Nb while the main anions are O, F and OH–. These elements are also the main components of other disilicates with similar optical properties common to alkaline rocks, e.g. rinkite-group minerals of the seidozerite supergroup (Sokolova and Cámara, Reference Sokolova and Cámara2017; Pautov et al. Reference Pautov, Agakhanov, Karpenko, Uvarova, Sokolova and Hawthorne2019). Most petrological studies of alkaline rocks do not utilise techniques other than chemical data to classify minerals. Therefore, we have explored the feasibility of (1) distinguishing wöhlerite-group minerals from related minerals and (2) classifying WGM down to species level solely based on EMPA data. We have used a total of 908 analyses of WGM and related minerals from our own work and the literature: Aarden and Gittins (Reference Aarden and Gittins1974); Ferguson (Reference Ferguson1978); Eggleton et al. (Reference Eggleton, Halford and Beams1979); Raade and Mladeck (Reference Raade and Mladeck1983); Hermezi et al. (Reference Hermezi, McKie and Hall1986); Marino and Roeder (1989); Merlino et al. (Reference Merlino, Perchiazzi, Khomyakov, Pushcharovsky, Kulikova and Kuzmin1990); Keller and Williams (Reference Keller and Williams1995); Sharygin et al. (Reference Sharygin, Stoppa and Kolesov1996a,Reference Sharygin, Stoppa and Kolesovb); Chao and Gault (Reference Chao and Gault1997); Jamtveit et al. (Reference Jamtveit, Dahlgren and Austrheim1997); Stoppa et al. (Reference Stoppa, Sharygin and Cundari1997); Atencio et al. (Reference Atencio, Coutinho, Ulbrich, Vlach, Rastsvetaeva and Pushcharovsky1999); Perchiazzi et al. (Reference Perchiazzi, McDonald, Gault, Johnsen and Merlino2000); Roda-Robles et al. (Reference Roda-Robles, Fontan, Monchoux, Sørensen and de Parseval2001); Shiraga et al. (Reference Shiraga, Kusachi, Kobayashi and Yamakawa2001); Federico and Peccerillo (Reference Federico and Peccerillo2002); Christiansen et al. (Reference Christiansen, Johnsen and Makovicky2003); Bellezza et al. (Reference Bellezza, Merlino and Perchiazzi2004a, Reference Bellezza, Franzini, Larsen, Merlino and Perchiazzi2004b); Mitchell and Belton (Reference Mitchell and Belton2004); Casillas et al. (Reference Casillas, Nagy, Demény, Ahijado and Fernández2008); Chakhmouradian et al. (Reference Chakhmouradian, Mitchell, Burns, Mikhailova and Reguir2008); Andersen et al. (Reference Andersen, Erambert, Larsen and Selbekk2010); Friis et al. (Reference Friis, Balić Žunić, Williams and Petersen2010); Owens and Kremser (Reference Owens and Kremser2010); Bellezza et al. (Reference Bellezza, Merlino and Perchiazzi2012); Biagioni et al. (Reference Biagioni, Merlino, Parodi and Perchiazzi2012); Chakrabarty et al. (Reference Chakrabarty, Mitchell, Ren, Sen and Pruseth2013); Chen et al. (Reference Chen, Simonetti and Burns2013); Melluso et al. (Reference Melluso, Morra, Guarino, De’ Gennaro, Franciosi and Grifa2014); Rønsbo et al. (Reference Rønsbo, Sørensen, Roda-Robles, Fontan and Monchoux2014); Comin-Chiaramonti et al. (Reference Comin-Chiaramonti, Renzulli, Ridolfi, Enrich, Gomes, De Min, Azzone and Ruberti2016); Melluso et al. (Reference Melluso, Guarino, Lustrino, Morra and De’ Gennaro2017); Chakrabarty et al. (Reference Chakrabarty, Mitchell, Ren, Pal, Pal and Sen2018); Sunde et al. (Reference Sunde, Friis and Andersen2018) and Guarino et al. (Reference Guarino, De’ Gennaro, Melluso, Ruberti and Azzone2019).
Before attempting a classification or discrimination of species based purely on chemistry, all data were recalculated on the basis of 18 anions. We have maintained the identification of each analytical point as given in the respective papers, i.e. we have not changed mineral identifications. The WGM typically have no substitution on the Si sites and the X sites are filled, i.e. there are no, or only limited, vacancies in fresh material. Therefore, only data where 3.9 < Si apfu < 4.1 and 7.8 < Σ X apfu < 8.2 should be treated. We allow for some variation from ideal stoichiometry due to the challenges of analyses some of these minerals. Of the 908 analytical points 258 did not fulfil these criteria and therefore the following is based on the remaining 650 analyses.
Keller and Williams (Reference Keller and Williams1995) used three ternary plots to classify WGMs, and we present our data in two of the same diagrams (Fig. 4). Contrary to the paper by Keller and Williams (Reference Keller and Williams1995) our data show significant overlaps between species. For example, hainite-(Y), kochite and rosenbuschite overlap in all plots and partly overlap with låvenite. Conversely, wöhlerite forms a distinct group in Fig. 4a,c,e,f. However, Fig. 4a shows that (i) janhaugite (WGM) overlaps with rinkite-group minerals grenmarite and seidozerite and (ii) låvenite (WGM) overlaps with rosenbuschite (rinkite group, seidozerite supergroup). Figure 4b,e show (i) a strong overlap between four minerals: wöhlerite-group minerals wöhlerite and hiortdahlite and rinkite-group minerals hainite-(Y), rinkite-(Ce) and rinkite-(Y).
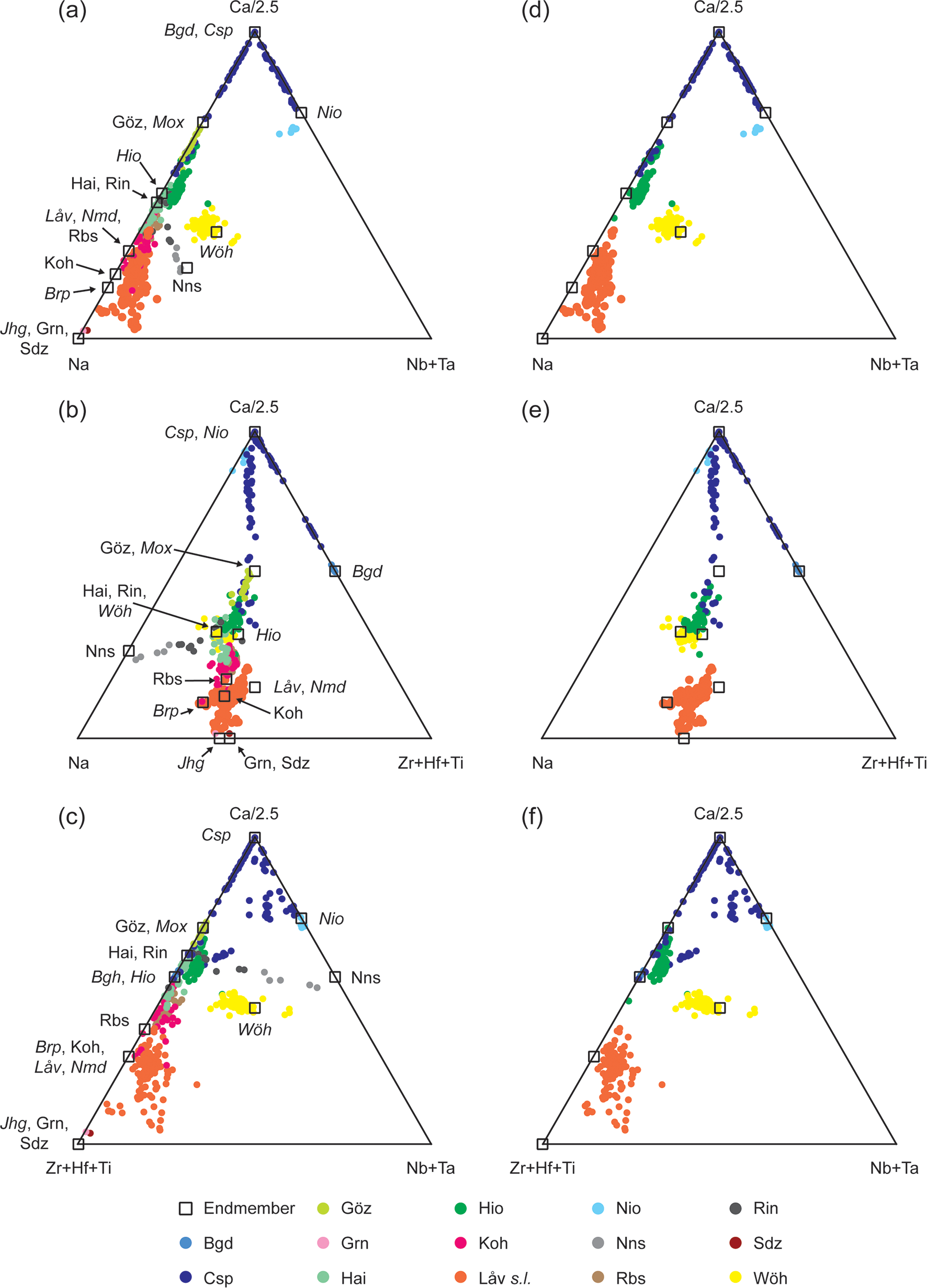
Fig. 4. (a–c) Ternary plots of 650 chemical analyses of WGM and related species from our data and the references listed in the text and (d–f) only WGM. Abbreviations: Baghdadite (Bgd); burpalite (Brp); cuspidine (Csp); götzenite (Göz); grenmarite (Grn); hainite-(Y) (Hai); hiortdahlite (Hio); janhaugite (Jhg); kochite (Koh); låvenite (Låv); moxuanxueite (Mox); niocalite (Nio); normandite (Nmd); nacareniobsite-(Ce) (Nns); rosenbuschite (Rbs); rinkite-(Ce) (Rin); seidozerite (Sdz) and wöhlerite (Wöh). In the figure the abbreviations are in italic for WGM.
Chakhmouradian et al. (Reference Chakhmouradian, Mitchell, Burns, Mikhailova and Reguir2008) plotted data for some WGM based only on divalent cations occupying the true octahedral sites. This method suffers from the same issues as Keller and Williams (Reference Keller and Williams1995) with large overlaps between different species, especially if data for hiortdahlite is included. Melluso et al. (Reference Melluso, Morra, Guarino, De’ Gennaro, Franciosi and Grifa2014) suggested other graphical methods to classify WGM and related species, but also concluded that the high degree of overlap between species and endmembers of species does not make these plots suitable for species determination.
The previously proposed methods for classification do not enable a satisfactory determination at species level from only chemical data. At first glance, the plots by Keller and Williams (Reference Keller and Williams1995) do seem to create some distinct groups and it may be possible to separate some species based on them. However, the data presented by Keller and Williams (Reference Keller and Williams1995) has in a sense already been filtered as they only presented WGM data. Therefore, these plots may help identify some WGM, but only when it is already known that the chemical data is actually from a WGM. Conversely, they fail when the mineral has not been identified, at least, to a group level. On the basis of the available chemical data it is not possible to make a graphic interpretation to identify WGM or distinguish them from related minerals solely based on chemical data. However, the plots may work if additional methods are applied, for example X-ray diffraction (XRD), to determine if the mineral is a member of the wöhlerite group or another chemically related group e.g. the seidozerite supergroup.
As the graphical methods do not enable distinction of WGM form related mineral groups, let alone identification at species level we have used the same chemical data to establish a workflow for treating chemical data. It must be stressed that the flow below requires the sequential removal of data so that the next step in the flow are criteria to be applied on the remaining data after samples have been removed by the previous step (Fig. 5).
1. Remove poor quality data.
2. Remove data with REE+Y > 0.5 apfu, which are REE-bearing rinkite-group minerals.
3. Remove data with sum Ti+Zr+Hf > 2.5 apfu. This removes seidozerite and grenmarite.
4. Remove data with Fe+Mn > 3 apfu, which will be janhaugite.
5. Remove data where Ca > 5.9 apfu. This step removes cuspidine–baghdadite–niocalite.
6. Remove samples with Ca < 2.9 apfu. This step removes låvenite, normandite and burpalite, but also some kochite.
7. Remove data with Nb+Ta > 0.5 apfu, which corresponds to wöhlerite.
8. Remove data with Ti/(Zr+Hf) < 0.2, which will separate hiortdahlite, moxuanxueite and one data point given as rosenbuschite in the literature.
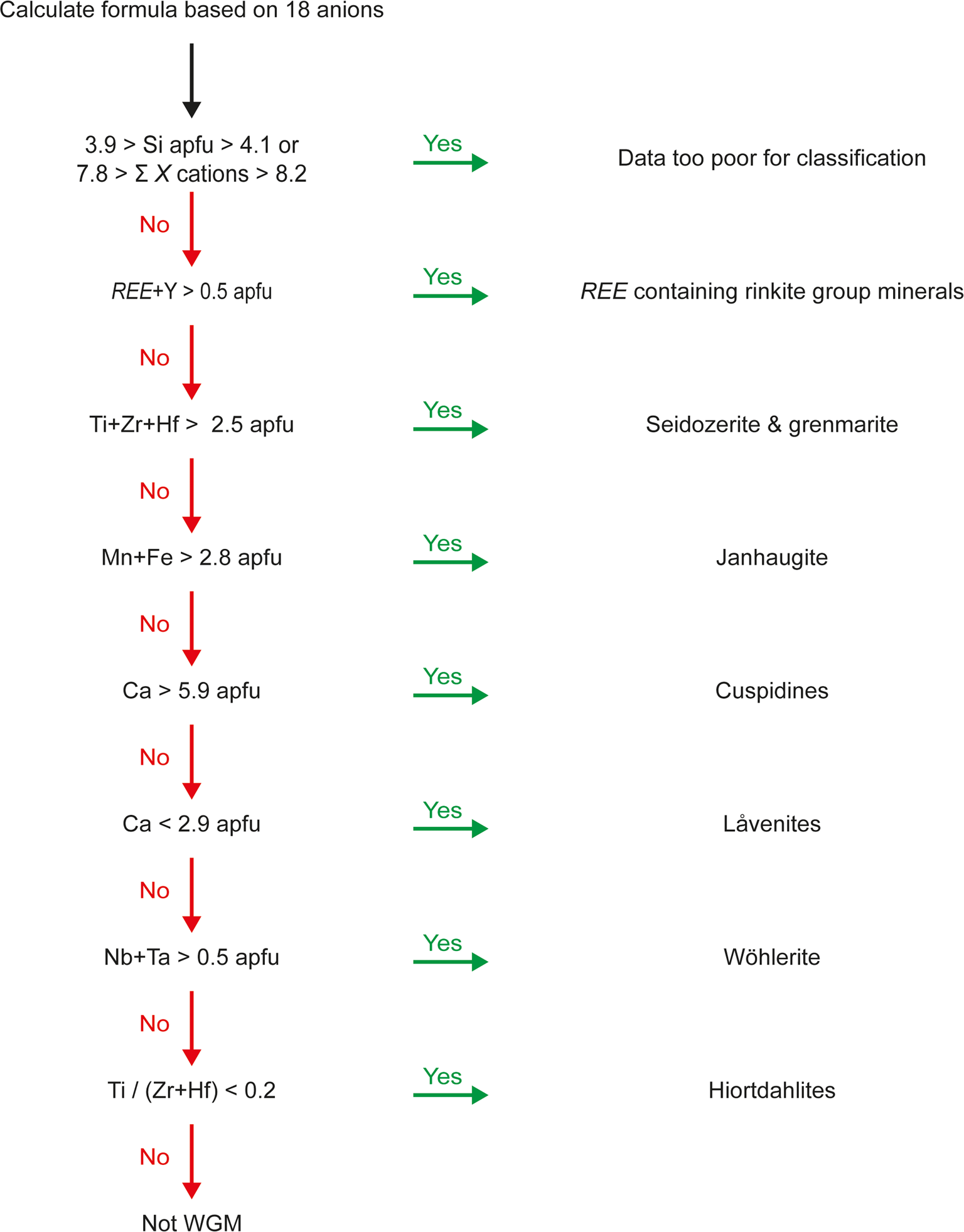
Fig. 5. Schematic workflow for discriminating chemical data into different disilicate groups.
After these steps a total of 122 data points remain corresponding to götzenite, hainite-(Y), kochite and rosenbuschite. In addition, one data point given as hiortdahlite and the Zr–Ti-cuspidine of Sharygin et al. (Reference Sharygin, Stoppa and Kolesov1996a) remains. The method above provides a good separation of WGM from seidozerite-group minerals.
Solid solution in WGM
The literature often refers to the WGM having a flexible structure resulting in large degree of solid solution (e.g. Perchiazzi et al., Reference Perchiazzi, McDonald, Gault, Johnsen and Merlino2000; Mitchell and Belton, Reference Mitchell and Belton2004; Chakhmouradian et al., Reference Chakhmouradian, Mitchell, Burns, Mikhailova and Reguir2008). Regardless of the diverse composition of the WGM, solid solutions are not as extensive as the chemical data may suggest. There seems to be a high degree of solid solution between cuspidine and niocalite, as well as between cuspidine and baghdadite, however, the solid solution between niocalite and baghdadite is limited (Fig. 4f). In baghdadite, the two Zr sites are edge sharing, resulting in a highly distorted site. If niobium completely replaces Zr, two Nb polyhedra would be edge sharing, which is highly unlikely to happen. Furthermore, a complete replacement of Zr by Nb is not possible as the additional two charges cannot be balanced becasue all anions in baghdadite are already oxygen. A full solid solution would be the coupled substitution 2Zr4+ + O2– ↔ Ca2+ + Nb5+ + F–, but this is not a simple substitution as baghdadite and niocalite are not isostructural, and it would lead to a major structural change (Fig. 2). However, it is likely that there is a limited solid solution between the two species as indicated by Casillas et al. (Reference Casillas, Nagy, Demény, Ahijado and Fernández2008). Keller and Williams (Reference Keller and Williams1995) suggested a degree of solid solution between niocalite and wöhlerite, but most of these data have more than 8.2 apfu X site cations and were removed by the above data processing. Wöhlerite and niocalite are not isostructural, therefore solid solution between the minerals not only requires substitution between Ca, Na, Zr and F, but also a change of the position of Nb in the structure between X5 and X6 (Tables 1,2; Fig. 3).
Sharygin et al. (Reference Sharygin, Stoppa and Kolesov1996a) investigated a series of cuspidine and götzenite minerals from Pian di Celle in Italy and suggested a partial solid solution between cuspidine and an end-member with the composition NaCa6Zr(Si2O7)2OF3. From powder XRD and Raman spectroscopy Sharygin et al. (Reference Sharygin, Stoppa and Kolesov1996a) showed this phase to be structurally more closely related to hiortdahlite than cuspidine, and in fact this composition corresponds to the recently approved mineral moxuanxueite (Qu et al., Reference Qu, Dong, Li, Fan, Wang, Cheng, Jin, Sun, Zhao and Wang2020).
Extended solid solution has been documented between låvenite and normandite (Perchiazzi et al., Reference Perchiazzi, McDonald, Gault, Johnsen and Merlino2000) as Zr in låvenite and Ti in normandite both occupy the X1 site and hence can replace each other with no additional structural modifications. Similarly, the Mn/Fe ratio differs in låvenite and as both these cations occupy the X3 site, it is likely that Fe-equivalent species may be found of both minerals.
In summary, solid solution in the WGM is controlled by the crystal structure and occurs where no major structural modifications are required, e.g. between låvenite and normandite, or between cuspidine, baghdadite and niocalite. Therefore, it is not uncommon to find several different WGM in the same rocks as small chemical changes favour the formation of multiple species rather than creating solid-solution series.
Nomenclature for wöhlerite-group minerals
Classification
The general formula for WGM can be expressed as X 8(Si2O7)2W 4, without any further distinction among the X and W sites. As shown in Fig. 2, a specific chemical element will have a different preferential X site in different WGM. For instance, high-field-strength elements (HFSE) such as Ti, Zr and Nb, are hosted on crystallographic sites located in different columns of the walls and on different cationic X sites. Consequently, there is no crystal-chemical feature to assign elements to specific X sites. As a rule, the topological representation of the cationic walls has been made by drawing the projection of the wall along the crystallographic c axis. The labelling of the sites always starts with X1, which is the smallest site belonging to outer columns of the wall. The site siting in the outer column and connected to X1 though edge-sharing is labelled X2. Afterwards the sites are labelled in succession, going from the outer to the inner columns of the wall, and according to the symmetry of the mineral. The crystal structure of hiortdahlite is characterised by two cationic walls, where wall I contains the HFSE-dominated site located in the outer column (Fig. 3). The anionic W sites correspond to the ligands that are not bonded to the disilicate groups. W1 and W2 are the anionic sites bonded to X1 and X2, respectively. Depending of the symmetry of the mineral, W3 and W4 are either belonging to the same outer column as W1 and W2, or to the second outer column of the wall. The W anionic sites occurring between the two inner columns are the symmetrical equivalents of the W sites defined previously.
The classification of WGM is based on the occupancy of the structural X and W sites, and the different combination of chemical elements on these sites are regarded as different mineral species. Considering only chemical data is in some cases insufficient to correctly identify WGM at a species level. Consequently, a structural refinement is needed for a complete characterisation. The sequential application of the dominant-valency and the dominant-constituent rules is suitable for the determination of the end-member formula (Hatert and Burke, Reference Hatert and Burke2008; Bosi et al., Reference Bosi, Hatert, Hålenius, Pasero, Miyawaki and Mills2019b). However, due to the possible occurrence of heterovalent substitutions on both the cationic and anionic sites, in some cases this method may fail to provide an end-member formula fulfilling the end-member definition (Hawthorne, Reference Hawthorne2021; Hawthorne et al., Reference Hawthorne, Mills, Hatert and Rumsey2021). Therefore, we suggest using the site-total charge (STC) approach to define the end-member formula (Bosi et al., Reference Bosi, Biagioni and Oberti2019a, Reference Bosi, Hatert, Hålenius, Pasero, Miyawaki and Mills2019b).
The relative position of the cation or anion inside the structural walls is not a valid criterion to define a new species, as it would lead to a proliferation of the number of species. A mineral phase showing the same chemical composition as previously described species but with a different cationic ordering must be considered as an analogue to that species. Polytypism is likely to occur among WGM (Merlino and Perchiazzi, Reference Merlino and Perchiazzi1988), and polytypes are not considered as different mineral species (Nickel and Grice, Reference Nickel and Grice1998). The polytypes must be described according to the nomenclature proposed in the IMA–CNMNC guidelines (Guinier et al., Reference Guinier, Bokij, Boll-Dornberger, Cowley, Dŭrovič, Jagodzinski, Krishna, de Wolff, Zvyagin, Cox, Goodman, Th, Kuchitsu and Abrahams1984; Nickel, Reference Nickel1993; Nickel and Grice, Reference Nickel and Grice1998).
Name, prefixes and suffixes
All the wöhlerite-group minerals have a distinct name, with no prefix or suffix. Due to the relatively large number of structural sites that are able to host the same cation (e.g. Ca2+), we strongly discourage the use of a compositional suffix (e.g. calcio-, ferro-), as this is unclear to which structural site these prefixes will be related. For the same reason, we also discourage the use of prefix referring to the composition of anionic W sites. In the case of a species characterised by one crystallographic site dominated by Y or REE, we recommend using a new rootname as well as the Levinson suffix (Bayliss and Levinson, Reference Bayliss and Levinson1988).
Hiortdahlite end-member formula
In order to define the correct end-member formula of hiortdahlite new chemical data was collected and a crystal structure refinement was performed on a sample from the type locality, Langodden, Langesundsfjord, Norway (samples located in the NHM Oslo collections, catalogue number KNR 24099). The chemical data were acquired using the CAMECA SX100 electron microprobe housed at the Department of Geosciences, University of Oslo. The instrument was operated with a beam current of 15 nA and an acceleration voltage of 15kV, creating a 10 μm spot. The following natural and synthetic standards were used: albite (Na), zircon (Zr), wollastonite (Ca and Si), pyrophanite (Ti and Mn), REE orthophosphate (Y, La, Ce and Nd; Jarosewich and Boatner, Reference Jarosewich and Boatner1991), MgO (Mg), Fe metal (Fe), Nb metal (Nb) and fluorite (F). The intensity data were corrected for inter-element overlaps and matrix effects using the PAP routine (Pouchou and Pichoir Reference Pouchou and Pichoir1984). The chemical data are compared with those published by Andersen et al. (Reference Andersen, Erambert, Larsen and Selbekk2010) on type-locality material (Table 3).
Table 3. Chemical data for hiortdahlite based on the average of 26 point analyses.

S.D. – standard deviation
Single-crystal X-ray data were collected at room temperature with monochromated MoKα radiation (λ = 0.71703 Å – 50 kV and 1 mA) on a Rigaku Synergy-S diffractometer equipped with a HyPix–6000He detector housed at NHM Oslo. The instrument has Kappa geometry and both data collection and subsequent data reduction, together with face-based absorption corrections were carried out using the Rigaku CrysAlisPro software. The details of the data collection and refinement are provided in Table 3. The initial structure solution in space group P $\bar{1}\;$was determined by the charge flipping method using the Superflip algorithm (Palatinus and Chapuis, Reference Palatinus and Chapuis2007), and the structural model was subsequently refined on the basis of F 2 with the Jana2006 software (Petříček et al., Reference Petříček, Dušek and Palatinus2014). All atoms were refined with anisotropic thermal parameters. The details of the refinement are provided in Table 4 and the atoms coordinates, anisotropic thermal parameters and detailed bond distances are provided in the Supplementary tables S1, S2 and S3. Free refinement of the site-scattering factors showed that the X3 (Na), X4 (Ca) and X6 (Ca) sites were fully occupied, and that X1 (Zr), X2 (Ca) and X5 (Ca) sites were slightly deviating from a full occupancy. To extract accurate site-scattering factors, mixed occupancies Zr + Ca (X7) and Ca + Na (X8) were refined. The empirical formula is recalculated on the basis of (O+F) = 18 apfu. The site-scattering factors and the established cationic distribution in the crystal structure are provided in Table 5 and the bond-valence table is provided in Table 6. The crystallographic information file has been deposited with the Principal Editor of Mineralogical Magazine and is available as Supplementary material.
Table 4. Data collection and structure refinement details for hiortdahlite from Langodden, Langesundsfjord, Norway.
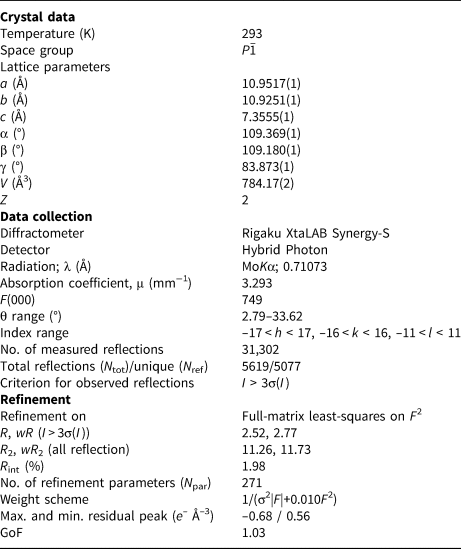
Table 5. Site population assignment and structural parameters for the crystal structure of hiortdahlite.

RSS: Refined site scattering (epfu); CSS: calculated site scattering (epfu); ABL: average observed bond-distances (Å); CBL: calculated averaged bond-distances (Å), using the ionic radii of Shannon (Reference Shannon1976).
Table 6. Detailed bond-valence table (vu) for the crystal structure of hiortdahlite.

Bond-valence parameters are recalculated according to the site occupancies and taken from Brown and Altermatt (Reference Brown and Altermatt1985) for the X–(O,F) distances and Gagné and Hawthorne (Reference Gagné and Hawthorne2015) for the Si–O distances. VS: bond-valence sums calculated according to the site occupancy.
As shown by Merlino and Perchiazzi (Reference Merlino and Perchiazzi1985), the main chemical substitutions occur on the X7 and X8 sites and the bond-valence analysis indicate a mixed O2– + F– occupancy on the anionic W1 site (Table 7). The refinement of the site scattering for the Ca1 (X2) and Ca3 (X5) sites shows a deficit and an excess of electronic density, respectively. Therefore, considering the average bond distances around these sites, Ca + Na and Ca + Y + REE have also been refined on these sites, respectively (Table 5). All Nb, Ti and Hf has been attributed to the Zr site (X1), which was then filled up with Zr. The remaining Zr was attributed to the X7 sites, along with all the Mn and Fe content. The X7 site was then filled with Ca. Refinement of the site scattering in the X8 position indicates an excess of electron density and therefore Ca was assumed to replace Na. The cationic distribution proposed is in excellent agreement with the different structural parameters and the bond-valence analysis (Tables 5, 6). Bond-valence sums show that the W2, W3 and W4 sites are populated by F– and that a substitution O2– ↔ F– is occurring on the W1 site (Table 6). Taking into account previous work and the new data presented here, the end-member formula of hiortdahlite is Na2Ca4(Ca0.5Zr0.5)Zr(Si2O7)2OF3, with a constrained mixed occupancy of Ca0.5Zr0.5 (M2+0.5M4+0.5)Σ3+, in order to obtain a charge-balanced formula.
Table 7. Site-population assignments in the crystal structure of hiortdahlite and hiortdahlite-related minerals.
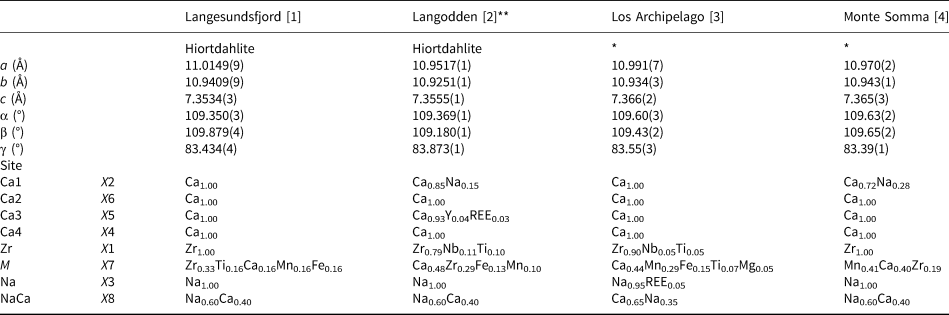
*Not hiortdahlite s.s. **Langodden (TL) – Structural formula: Na1.75Ca4.66Fe0.13Mn0.10Y0.04Ce0.03Zr1.08Nb0.11Ti0.10(Si2O7)2(O0.6F0.4)F3, Chemical formula: Na1.66Ca4.55Fe0.13Mn0.10Y0.04Ce0.03Zr1.08Nb0.11Ti0.10(Si1.98O7)2(O0.57F0.43)F3 (Table 3)
[1] Merlino and Perchiazzi (Reference Merlino and Perchiazzi1985); [2] this work; [3] Biagioni et al. (Reference Biagioni, Merlino, Parodi and Perchiazzi2012); [4] Domain IV, Bellezza et al. (Reference Bellezza, Merlino and Perchiazzi2012).
The refinement provided in this work is slightly different than the one proposed by Biagioni et al. (Reference Biagioni, Merlino, Parodi and Perchiazzi2012) on a mineral phase structurally related to hiortdahlite (Table 7). The incorporation of Ti4+ on the larger X7 site (<X7–O> = 2.233Å) instead of the smaller X1 site (<X1–O> = 2.082Å) is unlikely considering the ideal bond distance for octahedrally coordinated Ti (2.005Å, Shannon (Reference Shannon1976); Table 5, S3). The partitioning of Y and REE between the X5 and Na sites (X3) is not clear, however, we have not detected a refined site-scattering factor higher than 11 epfu for the X3 site in our investigations.
Hiortdahlite and moxuanxueite are the only approved WGM with a structure containing two topologically independent octahedral walls. The ideal compositions of the walls are given by wall-I[X 1ZrX 2CaX 3NaX 4Ca] and wall-II[X 5CaX 6CaX 7(Zr0.5Ca0.5)X 8Na]. The chemical compositions of the walls are similar, although in the first wall the Zr site is in the outer columns, while in the second wall the X7 site is in the central columns. Note that both walls in hiortdahlite are topologically and chemically unique among the WGM (Table 2).
Discreditation of marianoite
Marianoite was discovered from the silicocarbonatite Prairie Lake complex, Ontario, Canada (Chakhmouradian et al., Reference Chakhmouradian, Mitchell, Burns, Mikhailova and Reguir2008), and was considered the Nb-analogue of wöhlerite. Its simplified formula is Na2Ca4(Nb,Zr)2(Si2O7)2(O,F)4. Marianoite was described as monoclinic, P21, with a = 10.846, b = 10.226, c = 7.273 Å and β = 109.33°. The highest Nb content reported by Chakhmouradian et al. (Reference Chakhmouradian, Mitchell, Burns, Mikhailova and Reguir2008) for marianoite is 1.019 apfu, which is roughly 0.3 apfu more than in wöhlerite from the Langesundsfjord (Sunde et al, Reference Sunde, Friis and Andersen2018). The approval of marianoite as a valid mineral species was based on the assumption that both Zr and Nb are disordered on the two smallest octahedral sites [average bond lengths: 2.031 (X6) and 2.080 Å (X1)] occurring in the structure. As a result of the similar X-ray and neutron scattering characteristics of Zr and Nb, it is not possible to solve the ordering issue between these two chemical elements by using standard diffraction methods. Following the description of marianoite, Merlino and Mellini (Reference Merlino and Mellini2009) published a discussion arguing that in wöhlerite and marianoite there is an ordering of Zr and Nb, with Nb preferentially occupying the smallest site (X6) and Zr the second smallest octahedra (X1) of the structure. The same authors have proposed to solve this question through anomalous scattering using synchrotron radiation sources that will allow Zr and Nb to be distinguished. Bellezza et al. (Reference Bellezza, Merlino and Perchiazzi2012) and Biagioni et al. (Reference Biagioni, Merlino, Parodi and Perchiazzi2012) have used an ordered approach in their refinements. Readers are referred to Merlino and Mellini (Reference Merlino and Mellini2009) and Chakhmouradian and Mitchell (Reference Chakhmouradian and Mitchell2009) for more information on that discussion.
Following the classification system proposed herein for WGM, wöhlerite and marianoite are equivalent. If one considers a complete cationic ordering between Zr4+ and Nb5+, the resulting end-member formula for both wöhlerite and marianoite is Na2Ca4X 1(Zr)X 6(Nb)(Si2O7)2O3F. The maximum Nb content reported for marianoite is 1.02 apfu (associated with 0.85 Zr pfu) (Chakhmouradian et al., Reference Chakhmouradian, Mitchell, Burns, Mikhailova and Reguir2008), which is not enough to achieve Nb > Zr on both X1 and X6 sites and then define a Nb-dominant end-member. In the second case, if one considers that Zr4+ and Nb5+ are disordered, the X1 + X6 sites would have a total charge of +9, to keep the charge balance of the formula Na2Ca4(X1)(X6)(Si2O7)2O3F. The possible charge arrangements of the dominant cations R occupying the X1 and X6 sites are (i) X 1(R 4+) + X 6(R 5+) and (ii) X 1(R 4.5+) + X 6(R 4.5+). The charge arrangement (ii) is not valid, because it implies a double occupancy (Zr4+0.5Nb5+0.5) on two sites. The charge arrangement (i) leads to the end-member formula Na2Ca4X 1(R4+)X 6(R5+)(Si2O7)2O3F, and to the atomic arrangement Na2Ca4X 1(Zr4+)X 6(Nb5+)(Si2O7)2O3F. The atomic arrangement and the end-member formula are identical to those of wöhlerite. Consequently, marianoite must be considered equivalent to wöhlerite and is discredited.
Comment on the phase ‘hiortdahlite II’
‘Hiortdahlite II’ is not an approved mineral species, although Merlino and Perchiazzi (Reference Merlino and Perchiazzi1987) stated that the name ‘hiortdahlite II’ was approved by the IMA Commission on New Minerals and Mineral Names (merged with CNMNC in 2006), a subsequent new mineral proposal was never submitted. Hiortdahlite II was described by Aarden and Gittins (Reference Aarden and Gittins1974) in samples from the Kipawa River, Kipawa alkaline complex, Quebec, Canada. Roda Robles et al. (Reference Roda-Robles, Fontan, Monchoux, Sørensen and de Parseval2001) reported hiortdahlite II from the Ilímaussaq alkaline complex, Greenland, Tamazeght complex, Morocco, and Iles de Los, Guinea, based on chemical analyses and powder X-ray diffraction. We have analysed material from the same locality in the Ilímaussaq complex, and all of the supposed hiortdahlite II crystals have a unit-cell setting and a crystal structure identical to those of hiortdahlite. Therefore, it may be questionable that hiortdahlite II exists at these localities. This further emphasises the need for full crystal structure refinement to correctly identify WGM at a species level.
Chemical analysis on the ‘type’ material gave the formula (Na1.70Ca4.02Mn0.04Fe0.02Mg0.02Al0.02Y0.24REE0.08Zr1.16Nb0.04Ti0.02)Σ7.36(Si2.05O7)2O0.82OH0.36F2.68, and therefore hiortdahlite II was interpreted as a cationic-deficient analogue of hiortdahlite (Aarden and Gittins, Reference Aarden and Gittins1974). However, recent chemical analyses performed on the type material of hiortdahlite (Andersen et al., Reference Andersen, Erambert, Larsen and Selbekk2010) indicate roughly the same amount of Zr per unit formula than in the material described by Aarden and Gittins (Reference Aarden and Gittins1974). Note that hiortdahlite II contains up 0.24 Y apfu, while in hiortdahlite from Langodden, the Y content is below 0.05 apfu. The total REE content is also slightly larger in hiortdahlite II than in hiortdahlite.
Hiortdahlite II is reported as triclinic, P $\bar{1}$, with a = 10.95, b = 10.31, c = 7.29 Å, α = 90.19, β = 109.02 and γ = 90.05° (Aarden and Gittins, Reference Aarden and Gittins1974). The crystal structure refinement was performed on samples from Kipawa, and gave a structural model based on two independent topological octahedral walls (Merlino and Perchiazzi, Reference Merlino and Perchiazzi1987). The wall-I in hiortdahlite II has the same chemical composition as the wall-I in hiortdahlite, though the cationic distribution is not strictly equivalent (Fig. 3). The main difference between species is observed in the topology of wall-II, with a composition of [X5CaX6CaX7(Zr0.5Ca0.5)X8Na] and [X5YX6CaX7CaX8(Ca0.5Na0.5)] in hiortdahlite and hiortdahlite II, respectively. However, the difference between the crystal chemical formula provided by Merlino and Perchiazzi (Reference Merlino and Perchiazzi1987) and the chemical data reported by Aarden and Gittins (Reference Aarden and Gittins1974) is significant, for instance 1.76 Y apfu is reported in the structure while the chemical data indicate 0.32 Y + REE apfu.
Consequently, the presence of a Y-dominant site in the structure of hiortdahlite II is questionable, and new investigations must be performed on hiortdahlite II material to explore if it is a polytype of hiortdahlite or a distinct and valid mineral species. A new mineral proposal would still be required to be submitted to the IMA–CNMNC.
Conclusions
The general formula of the wöhlerite-group minerals is given by X 8(Si2O7)2W 4, where X denotes the cations occurring in the polyhedra building the four-column wall, and where W denotes the anionic sites that are not bonded to the disilicate groups. The crystal structure of WGM is characterised by ‘octahedral walls’ made of four columns of edge-sharing X sites. The symmetry of the different species can vary from monoclinic to triclinic, according to the cationic ordering on the X sites and the relative position of the disilicate groups. Distinction between the mineral species is made based on the dominant elements at the X and W sites, and different combinations of X and W constituents should be regarded as separate mineral species.
In addition to the classification scheme, the following changes have been approved by the IMA–CNMNC: (i) the end-member formula of hiortdahlite has changed to Na2Ca4(Ca0.5Zr0.5)Zr(Si2O7)2OF3, with a valency-imposed double-site occupancy of (Ca2+0.5Zr4+0.5)Σ3+ on the X7 site, and (ii) marianoite is discredited, as it is structurally and chemically equivalent to wöhlerite.
The chemical variation in WGM results in the formation of individual species rather than solid-solution series. The reason being that despite similar compositions between many of the members they are not isostructural, therefore, heterovalent substitutions typically require a complete reorder of the structure. Such reorders appear energetically unfavourable compared to the formation of another species, commonly resulting in rocks containing several WGM or even seidozerite supergroup minerals. Furthermore, the co-existence of different WGM and seidozerite-supergroup minerals in the same rock makes it a challenge for petrologists to identify minerals on a species level. We proposed a discrimination flow-chart for separating various WGM from chemically related species.
Acknowledgements
We thank Marco Pasero and all members of the IMA–CNMNC for their helpful suggestions and comments. Muriel Erambert is thanked for her assistance with the EMPA data collection of the hiortdahlite material. We wish to thank Qu Kai for the discussion around moxuanxueite. We are grateful to George Christidis and Patrick Woodward for Editorial handling and we thank Anton Chakhmouradian and three additional anonymous reviewers for their comments on the manuscript.
Supplementary material
To view supplementary material for this article, please visit https://doi.org/10.1180/mgm.2022.10