Introduction
The Ediacara biota represents an assemblage of large, soft-bodied, and structurally complex organisms of uncertain affinities (Laflamme et al., Reference Laflamme, Darroch, Tweedt, Peterson and Erwin2013; Droser and Gehling, Reference Droser and Gehling2015). Earliest attempts to classify these organisms, on the basis of morphological comparisons with modern taxa, resulted in their interpretation as primitive examples of crown-group animals (see Glaessner, Reference Glaessner1979); however, recent studies instead suggest that the Ediacara biota consists of a number of distinct groups rather than a single clade, thus shifting the debate to discussions of stem animals and crown animals and clades outside of Metazoa (Xiao and Laflamme, Reference Xiao and Laflamme2009; Erwin et al., Reference Erwin, Laflamme, Tweedt, Sperling, Pisani and Peterson2011; Laflamme et al., Reference Laflamme, Darroch, Tweedt, Peterson and Erwin2013; Dececchi et al., Reference Dececchi, Narbonne, Greentree and Laflamme2017; although see Budd and Jensen, Reference Budd and Jensen2015). Their disappearance prior to the diversification of complex metazoans has been proposed to represent the first biologically driven mass extinction event (Darroch et al., Reference Darroch, Sperling, Boag, Racicot, Mason, Morgan, Tweedt, Myrow, Johnston, Erwin and Laflamme2015, Reference Darroch, Boag, Racicot, Tweedt, Mason, Erwin and Laflamme2016; Schiffbauer et al., Reference Schiffbauer, Huntley, O’Neil, Darroch, Laflamme and Cai2016). The term ‘Ediacara biota’ is herein applied to the cast and mold impressions of soft-bodied organisms of uncertain affinity from the Ediacaran Period (MacGabhann, Reference MacGabhann2014).
Erwin et al. (Reference Erwin, Laflamme, Tweedt, Sperling, Pisani and Peterson2011) proposed a new classification for Ediacaran macrofossils, which utilizes features such as branching or segment architecture, body symmetry, associated trace fossils, and growth parameters, to erect distinct higher-level groupings based on shared (presumed derived) morphological, behavioral, and ontogenetic characters, while eliminating traditional groupings based on implied ecological similarities (see discussions in Laflamme and Narbonne, Reference Laflamme and Narbonne2008a, Reference Laflamme and Narbonneb). This classification restricts direct comparisons with modern taxa unless synapomorphies are shared. One such proposed clade, the Arboreomorpha, consists of fronds with typically bifoliate (possibly multifoliate) petaloids with parallel primary branches that are attached to a sheet and end at an outer rim (Erwin et al., Reference Erwin, Laflamme, Tweedt, Sperling, Pisani and Peterson2011; Laflamme et al., Reference Laflamme, Darroch, Tweedt, Peterson and Erwin2013; Dececchi et al., Reference Dececchi, Narbonne, Greentree and Laflamme2017). The Arboreomorpha are distinct from Rangeomorpha in lacking a self-repeating and modular branching pattern (Laflamme and Narbonne, Reference Laflamme and Narbonne2008a, Reference Laflamme and Narbonneb; Erwin et al., Reference Erwin, Laflamme, Tweedt, Sperling, Pisani and Peterson2011; Brasier et al., Reference Brasier, Antcliffe and Liu2012); instead, the secondary branches are globular and typically lack further subdivisions. A similar classification scheme proposed the “Frondomorpha” for Charniodiscus (in addition to other fronds and an assortment of likely holdfasts), defined as Ediacaran fossils “composed of three distinct parts: a large, relatively flattened foliate section, a central stem, and a holdfast or rooting anchor” (Grazhdankin, Reference Grazhdankin2014, p. 271–272). However, this definition would include Rangeomorpha such as Charnia, Beothukis, and Culmofrons and Erniettomorpha such as Swartpuntia (Dececchi et al., Reference Dececchi, Narbonne, Greentree and Laflamme2017); instead, it is most likely that the frond morphology is a shared morphological adaptation to elevating a feeding structure into the water column (Laflamme and Narbonne, Reference Laflamme and Narbonne2008a).
The Arboreomorpha are known from the Avalon and White Sea assemblages (Waggoner, Reference Waggoner2003; Boag et al., Reference Boag, Darroch and Laflamme2016), with specimens described from Newfoundland (Canada), Charnwood Forest (England), White Sea (Russia), Ukraine, Siberia, Wernecke and Mackenzie Mountains (northwestern Canada), the Yangtze Gorges area of South China, and the Ediacara Member of the Flinders Ranges of South Australia. All known Arboreomorpha are benthic epifaunal fronds that effectively partitioned the water column on a macroscopic level, resulting in complex tiered Ediacaran ecosystems (Clapham and Narbonne, Reference Clapham and Narbonne2002; Ghisalberti et al., Reference Ghisalberti, Gold, Laflamme, Clapham, Narbonne, Summons, Johnston and Jacobs.2014).
The limited taphonomic windows offered by most Ediacaran localities preserve impressions of two-dimensional casts and molds in medium to coarse sandstones (Narbonne, Reference Narbonne2005; Kenchington and Wilby, Reference Kenchington and Wilby2014), limiting morphological information to external structures. Taphonomic studies distinguishing between genuine structures and morphological “mistakes” resulting from bending, folding, or overlapping relationships have been essential in forwarding taxonomic and phylogenetic research (Laflamme et al., Reference Laflamme, Narbonne, Greentree and Anderson2007; Liu et al., Reference Liu, McIlroy, Antcliffe and Brasier2011; Brasier et al., Reference Brasier, Liu, Menon, Matthews, McIlroy and Wacey2013; Matthews et al., Reference Matthews, Liu and McIlroy2017). Rare glimpses into three-dimensional morphology are offered by exceptional sites in Namibia (Vickers-Rich et al., Reference Vickers-Rich2013; Ivantsov et al., Reference Ivantsov, Narbonne, Trusler, Greentree and Vickers-Rich2016), Newfoundland (Narbonne, Reference Narbonne2004; Narbonne et al., Reference Narbonne, Laflamme, Greentree and Trusler2009), and the White Sea (Grazhdankin, Reference Grazhdankin2014; Ivantsov, Reference Ivantsov2016).
The discovery of several exquisitely preserved, three-dimensional examples of the classic Ediacaran frond Arborea (previously Charniodiscus, see systematic taxonomy) from the Ediacara Member, Rawnsley Quartzite of South Australia, allows for the reevaluation of this genus and adds insight into the internal construction and likely external morphology of this frond. Preservation of inferred internal anatomy, combined with detailed preservation of the branching architecture, also helps refine the classification of Arboreomorpha.
Geological setting and stratigraphy
The Ediacaran assemblages found within the Flinders Ranges of South Australia contain some of the highest diversity of Ediacara biota (Droser et al., Reference Droser, Gehling and Jensen2006; Droser and Gehling, Reference Droser and Gehling2015), the broadest range of occupied ecological niches (Bambach et al., Reference Bambach, Bush and Erwin2007; Bush et al., Reference Bush, Bambach and Erwin2011; Laflamme et al., Reference Laflamme, Darroch, Tweedt, Peterson and Erwin2013), and the first probable examples of stem-group Bilateria anywhere in the world (Fedonkin and Waggoner, Reference Fedonkin and Waggoner1997). Ediacaran fossils in South Australia are typically restricted to the Ediacara Member of the Rawnsley Quartzite (Gehling, Reference Gehling2000; Gehling and Droser, Reference Gehling and Droser2013) in the upper portion of the Pound Subgroup, Wilpena Group, which overlies the global Marinoan glacial tillite that marks the base of the Ediacaran Period (Knoll et al., Reference Knoll, Walter, Narbonne and Christie-Blick2006). Volcanic ash layers are unknown from the Flinders Ranges; however, paleobiological studies have linked the Flinders Ranges with the White Sea assemblage of Russia (Waggoner, Reference Waggoner2003; Boag et al., Reference Boag, Darroch and Laflamme2016), which has been radiometrically dated at 555 Ma (Martin et al., Reference Martin, Grazhdankin, Bowring, Evans, Fedonkin and Kirschvink2000).
For over a decade, diligent excavations of complete Ediacaran surfaces from the National Heritage Listed Ediacara Fossil Site at Nilpena (herein referred to as Nilpena) in the Flinders Ranges of South Australia (see reviews in Droser et al., Reference Droser, Gehling and Jensen2006; Gehling and Droser, Reference Gehling and Droser2013; Droser and Gehling, Reference Droser and Gehling2015) have uncovered a diverse assemblage of Ediacara biota occupying specific ecological niches and representative sedimentary facies including shoreface sands, wave-base sands, delta-front sands, sheet-flow sands, and mass-flow sands. Although Arborea is known from all these facies, wave-base and sheet-flow sands offer the greatest abundance of specimens (Gehling and Droser, Reference Gehling and Droser2013); however, the mass-flow sands facies has been responsible for exquisite, three-dimensional preservation of large Arborea fronds allowing for unprecedented levels of morphological detail. For our purposes, 3D preservation is distinguished from typical Ediacaran two-dimensional preservation as representing moldic sand infills that can be isolated and removed from external molds in the surrounding matrix.
Taphonomy
The influence of taphonomy on Ediacaran taxonomy is profound. Although emerging taphonomic studies demonstrate a common reliance on clays and bacterially mediated precipitation of iron sulfides associated with Ediacaran preservation (Gehling, Reference Gehling1999; Laflamme et al., Reference Laflamme, Schiffbauer, Narbonne and Briggs2011, Darroch et al., Reference Darroch, Laflamme, Schiffbauer and Briggs2012; Schiffbauer et al., Reference Schiffbauer, Xiao, Cai, Wallace, Hua, Hunter, Xu, Peng and Kaufman2014; Liu, Reference Liu2016), Ediacaran-type preservation spans a remarkably large lithological spectrum, resulting in an equally expansive range of taphomorphs (Grazhdankin et al., Reference Grazhdankin, Balthasar, Nagovitsin and Kochnev2008; Xiao et al., Reference Xiao, Droser, Gehling, Hughes, Wan, Chen and Yuan2013; Kenchington and Wilby, Reference Kenchington and Wilby2014). Distinct preservational styles (of Narbonne, Reference Narbonne2005) are typically limited to single localities, representing an intimate association between sedimentary facies, depositional context, and ambient water energy irrespective of the qualities of biological tissue constructing these organisms.
Preservation of the soft-bodied Ediacara biota from South Australia results from the intimate relationship between the organisms and expanses of seafloor microbial mats (Gehling, Reference Gehling1999; Gehling et al., Reference Gehling, Droser, Jensen and Runnegar2005; Gehling and Droser, Reference Gehling and Droser2009; Darroch et al., Reference Darroch, Laflamme, Schiffbauer and Briggs2012). The ‘death mask’ model (Gehling, Reference Gehling1999) predicts that after burial by storm deposits, new colonies of microbial mats restricted the transport of oxygenated pore waters into the underlying sands. In the presence of decaying organic material (i.e., buried microbial mats and Ediacara biota), sulfate trapped in the pore waters was reduced through the metabolic activities of sulfur-reducing bacteria, resulting in pyritic coatings that form the basis of Flinders-type preservation (Narbonne, Reference Narbonne2005). Evidence such as pyritized bacterial mats (Gehling et al., Reference Gehling, Droser, Jensen and Runnegar2005), ‘old elephant skin’ (Gehling, Reference Gehling1999), preserved microbial filaments (Gehling et al., Reference Gehling, Droser, Jensen and Runnegar2005; Callow and Brasier, Reference Callow and Brasier2009), and microbially induced sedimentary structures (Gehling and Droser, Reference Gehling and Droser2009) on the beds containing fossils all suggest a direct link between bacterial mats and Ediacaran preservation. Recently, it has been suggested that the relatively higher silica saturation state of Neoproterozoic oceans prior to the advent of abundant silica biomineralizers aided in rapid sediment lithification via early-stage precipitation of silica cements (Tarhan et al., Reference Tarhan, Hood, Droser, Gehling and Briggs2016).
New discoveries of exquisitely preserved Ediacaran fronds from Nilpena Farm in South Australia exhibit three-dimensional casting of soft-bodied Ediacaran organisms within fine-grained event beds resulting from storm or mass-flow events. The three-dimensional casting has been essential in differentiating multiple petaloids in multifoliate fronds such as Rangea and Swartpuntia (Narbonne et al., Reference Narbonne, Saylor and Grotzinger1997; Vickers-Rich et al., Reference Vickers-Rich2013) and typically results in finer-detailed preservation (Narbonne, Reference Narbonne2004; Narbonne et al., Reference Narbonne, Laflamme, Greentree and Trusler2009). It was proposed by Dzik (Reference Dzik1999) and Narbonne (Reference Narbonne2005) that Nama-style preservation may allow for the casting of internal rather than external features, therefore revealing hypothesized internal, soft-bodied organic skeletons serving as primary structural supports for epifaunal fronds.
The combination of a variety of preservational types of the same taxon within a single, temporally restrictive locality provides a unique opportunity to dissect the internal and external morphology of an Ediacaran organism and refines the interpretation of characters preserved in two-dimensional casts.
Frond morphology and the history of Charniodiscus and Arborea
Ediacaran fronds (Fig. 1) represent a convergent evolution toward a shape that elevates a foliate feeding structure higher into the water column (Laflamme and Narbonne, Reference Laflamme and Narbonne2008a, Reference Laflamme and Narbonneb) to effectively reduce competition with crowded lower tiers (Clapham and Narbonne, Reference Clapham and Narbonne2002; Ghisalberti et al., Reference Ghisalberti, Gold, Laflamme, Clapham, Narbonne, Summons, Johnston and Jacobs.2014). Arboreomorpha frond morphology consists of a basal anchoring structure typically circular to bulbous in shape, which was either buried beneath/within (Tarhan et al., Reference Tarhan, Gehling and Droser2010; Laflamme et al., Reference Laflamme, Schiffbauer, Narbonne and Briggs2011; Burzynski and Narbonne, Reference Burzynski and Narbonne2015) or stuck to the upper surface (Seilacher, Reference Seilacher1992) of benthic microbial mats. The anchoring disc was attached to a cylindrical stem from which emerged a bifoliate petalodium (possibly multifoliate; Brasier and Antcliffe, Reference Brasier and Antcliffe2009). Petaloids are composed of several primary branches that branch off directly from the central stalk.
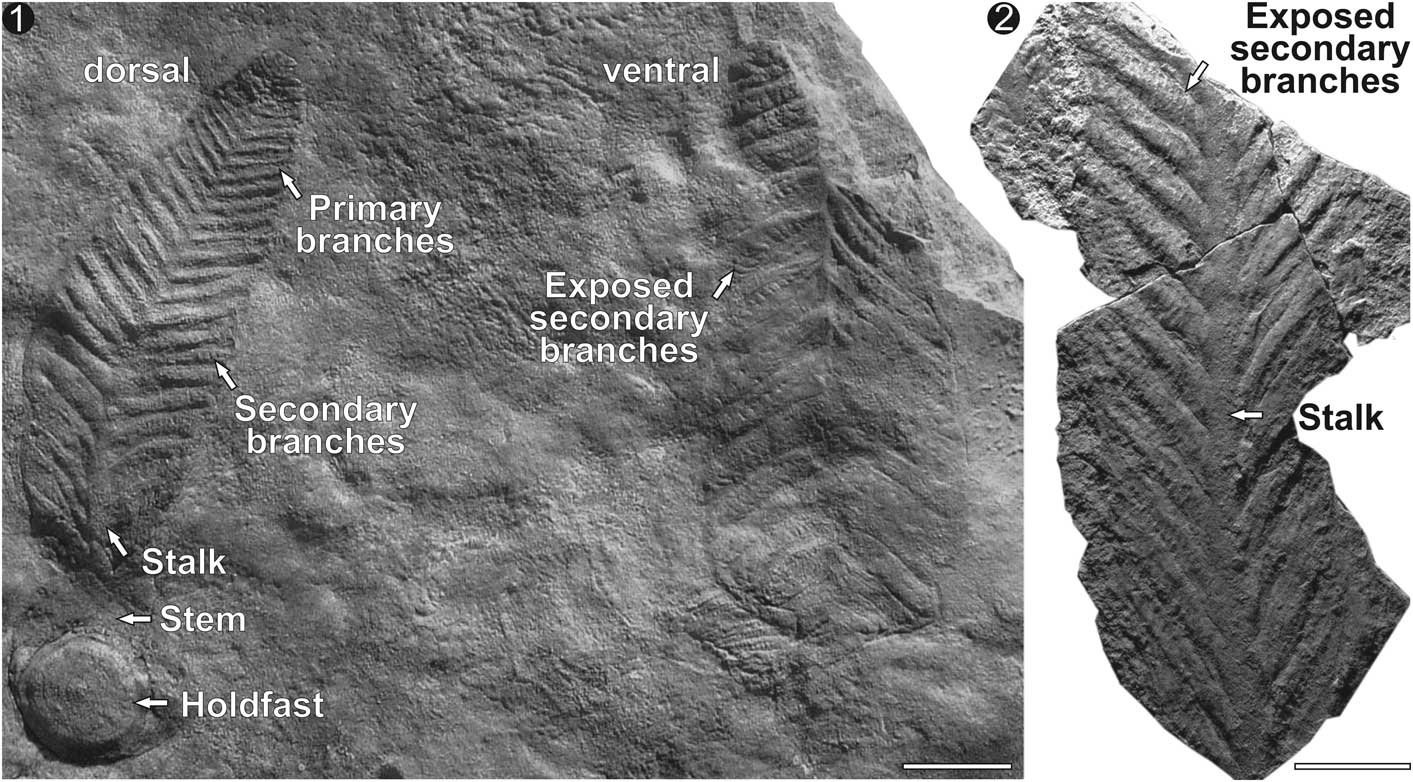
Figure 1 Frond morphology: (1) SAM P19690a, b representing dorsal and ventral sides, respectively, on the sole of a 30 cm thick sandstone bed from Bunyeroo Gorge. (2) SAM P49416. Each petaloid consists of numerous primary branches attached to a central stalk and composed of several secondary branches. Petalodium attached to a stem and anchored by a circular holdfast disc. Scale bar=5 cm.
The poor preservation of the holotype of Charniodiscus concentricus Ford, Reference Ford1958 makes it difficult to evaluate the nature of the petalodium. Brasier and Antcliffe (Reference Brasier and Antcliffe2009, fig. 12) suggested that C. concentricus could represent a multifoliate frond, consisting of several independent petaloids all circling a central stalk (“Laser scanning also suggests that Charniodiscus concentricus had three or more rows of segments [Dzik Reference Dzik2002], much like Rangea [Gurich 1929, 1930; Germs 1973; Jenkins 1985] and Swartpuntia [Narbonne et al. Reference Narbonne, Saylor and Grotzinger1997], rather than the two rows…”; Brasier and Antcliffe, Reference Brasier and Antcliffe2009, p. 372), and that much of the disorganization of the petaloidium could be explained by overfolded petaloids (“Interpretative drawing showing inferred presence of three or more rows of 1° branches” [note ‘1° branches’=primary branches, and ‘rows’=petaloids]; Brasier and Antcliffe, Reference Brasier and Antcliffe2009, p. 375). The multifoliate nature of the holotype was also proposed by Dzik (Reference Dzik2002), who suggested that the poor preservation may have resulted from the presence of “more than two vanes” (vanes=petaloids; Dzik, Reference Dzik2002, p. 322) and that “available evidence from Charniodiscus is compatible with the four-vane model of Rangea anatomy” (Dzik, Reference Dzik2002, p. 322). If true, the multifoliate petalodium in C. concentricus is strikingly different from Australian specimens presently referred to as C. arborea and C. oppositus Jenkins and Gehling, Reference Jenkins and Gehling1978, which display clear bifoliate symmetry with only two petaloids. We propose that this represents a fundamental difference in construction, and as a result we follow the recommendations of Brasier and Antcliffe (Reference Brasier and Antcliffe2009) and Dzik (Reference Dzik2002) in referring to C. arborea and C. oppositus as Arborea Glaessner and Daily, Reference Glaessner and Daily1959.
Another contentious aspect concerns the architecture of the primary branches in Charniodiscus and Arborea. Brasier and Antcliffe (Reference Brasier and Antcliffe2009) interpreted the primary branches in the holotype of C. concentricus as repeatedly branched elements that resemble the fractal branching of rangeomorphs such as Charnia and Rangea (Narbonne, Reference Narbonne2004; Dececchi et al., Reference Dececchi, Narbonne, Greentree and Laflamme2017), albeit not as well preserved. When investigating the secondary branching in the holotype, Brasier and Antcliffe (Reference Brasier and Antcliffe2009) concluded that “Laser profiles confirm that these arcuate 1° branches are further subdivided into numerous 2° branches. Their rangeomorph structure is not displayed. No examples of 3° or 4° branches have yet been seen in the holotype” (Brasier and Antcliffe, Reference Brasier and Antcliffe2009, p. 372). This condition was further explored by Brasier et al. (Reference Brasier, Antcliffe and Liu2012), who applied the term ‘furling’ to account for the difference in structure between typical rangeomorphs and Charniodiscus: “rangeomorph units furled but undivided at first-order level, so that second-order subdivisions cannot be seen (cf. some examples of Charniodiscus)” (Brasier et al., Reference Brasier, Antcliffe and Liu2012, p. 1111). By contrast, Laflamme et al. (Reference Laflamme, Narbonne and Anderson2004) and Laflamme and Narbonne (Reference Laflamme and Narbonne2008a) argued that Arborea from Australia were composed of a series of parallel, peapod-like primary branches that housed several oval secondary branches that lacked modular subdivisions; this branching was termed Arborea-type branching (Laflamme and Narbonne, Reference Laflamme and Narbonne2008a). The crux of this distinction in branching, either a furled rangeomorph branch (Brasier et al., Reference Brasier, Antcliffe and Liu2012) or a morphologically (and evolutionarily) distinct arboreomorph branch (Laflamme and Narbonne, Reference Laflamme and Narbonne2008a), will play heavily in our continued attempts to decipher the natural history of these Ediacaran clades. The debate can be summarized as the explanation of the simplified branching morphology seen in Charniodiscus (holotype) and Arborea—does it represent a modified (or poorly preserved) rangeomorph branch, thus allying them with Rangea and Charnia, or does it represent an independent ancestry, thus justifying Arboreomorpha as a clade (Dececchi et al., Reference Dececchi, Narbonne, Greentree and Laflamme2017)?
In summary, assuming that: (1) the petaloid arrangement in Arborea from Australia is distinct from the structure in Charniodiscus from England (multifoliate), and (2) the primary branching architecture (rangeomorph vs. arboreomorph) may also be distinct, we recommend the use of the traditional Australian designation of Arborea to represent bifoliate fronds with Arborea-type primary branching from Australia, while Charniodiscus is kept for multifoliate frondose forms from Charnwood Forest England. Considering these new diagnostic structures, a revision of Newfoundland Charniodiscus material is warranted but beyond the scope of this study. Ultimately, the present study of exceptionally preserved Arborea allows for a refined description of Arboreomorpha primary branches (Dececchi et al., Reference Dececchi, Narbonne, Greentree and Laflamme2017) as presented in the Systematic Paleontology.
Materials and methods
With the discovery of multiple, three-dimensionally preserved Arborea fronds from Nilpena in the Flinders Ranges of South Australia, the architecture of the primary branches can be thoroughly evaluated. Furthermore, by comparing various taphomorphs of Arborea from South Australia, it is possible to differentiate between dorsal and ventral sides of the petalodium and isolate internal structures that are preserved as composite molding.
Repository and institutional abbreviation.–In the following section, a new description of Arborea is based on 84 specimens from the South Australian Museum (SAM).
Systematic paleontology
Clade Arboreomorpha Erwin et al., Reference Erwin, Laflamme, Tweedt, Sperling, Pisani and Peterson2011
Remarks
Currently there is no agreed-upon taxonomic hierarchy for Ediacara biota above the rank of genus. For this reason, the term “Clade” is applied to indicate a group of closely related Ediacara biota. See Erwin et al. (Reference Erwin, Laflamme, Tweedt, Sperling, Pisani and Peterson2011) and Laflamme et al. (Reference Laflamme, Darroch, Tweedt, Peterson and Erwin2013) for discussion and rationale.
Phylum, Class, Order, and Family indeterminate
Genus Arborea Glaessner and Wade, Reference Glaessner and Wade1966

Figure 2 Circular Arborea holdfasts attached to cylindrical stem. (1) Specimen P14186 with large central boss and several radial striations most likely representing soft-tissue folding during compression. (2) Specimen P40332 with deflated disc similar in size to the stem. Scale bars=1 cm.

Figure 3 Stalk: taphonomic variations in stalk morphology. (1) Specimen P13800 with cylindrical stalk and peg-like primary branches originating from within the boundaries of the stalk. (2) Specimen P12895 with parallel-sided stalk and zigzagging central axis resulting from alternating primary branching architecture. (3) Specimen P40952 with alternate branching to form a zigzagging central axis but lacking the outline of the cylindrical stalk seen in (2). (4) Specimen P40444 displaying all three (A–C) stalk morphologies. Letters along stalk refer to taphomorphs highlighted in (1–3) (A=1, B=2, C=3). (5) Interpretive diagram of the three stalk taphomorphs with letters for reference. Compaction results in flattening of the stalk and composite molding of the primary branches. (1–4) Scale bars=1 cm.

Figure 4 Primary branching morphology in Arborea from the Ediacara Member of the Pound Subgroup. Branches arranged in increasing quality of preservation, from poorest-preserved branches to highest-quality preservation with secondary and possibly tertiary branching exposed. (1) Specimen P34499 displaying parallel rectangular primary branches with possible secondary branching structures perpendicular to the primary branches. (2) Specimen P13801b with peg-like margins to the primary branches that pass beyond the margin of the stalk, suggesting the anchoring site is internal to the stalk and preserved as a composite mold. (3) Specimen P14307 with primary branches composed of peg-like structures. (4) Specimen P40775 displaying an arched primary branch with distinct secondary branching emerging from the base of the branch and overlying the base of the subsequent adjacent branch. (5) Specimen P40776 with well-defined cylindrical primary branch stalks and rounded to globular secondary branching. Secondary branching begins in the middle of the primary branch, attached to the primary branching stalks. (6) Specimen P40786, with higher-relief primary branches and with evidence of an organic sheet behind the branches, attaching them together and preventing significant branch movement. All scale bars=1 cm.

Figure 5 Secondary branches in SAM P35702 from Nilpena Farm: (1) Part (P) and counterpart (CP) of specimen preserved in three dimensions, displaying prominent central stalk and several primary and secondary branches. (2) Close-up of two primary branches (A) displaying the secondary branches (SB) stemming from the primary branch stalk (PBS) and crossing over the furrow between the primary branches. (3) Same primary branches as (2) but in counterpart specimen (B). (4) Top view of broken piece of primary branch displaying secondary branching (C). (5) Same piece as (4) (C’) but rotated on its side to show side profile of peg-like secondary branches. Scale bars=1 cm or 1 cm increments.

Figure 6 Specimen P49364a, b from Nilpena Farm: (1) Specimen preserved in three dimensions. Presumed location of central stalk according to branching orientation. (2) Primary branch displaying secondary branches (SB) stemming from the primary branching stalk (PBS). (3, 4) Part and counterpart of a (A) cross section through a primary branch broken away from adjacent branches and displaying (B) teardrop secondary branches. Black arrow indicates location of displaced secondary branch. Scale bars=1 cm or 1 cm increments.

Figure 7 SAM P35704a, b from Nilpena Farm: (1) Part (P; facing downward) and counterpart (CP; facing upward) of SAM P35704a, b. (2–4) Secondary branches stemming from primary branching stalk and oriented in the opposite direction (directed proximally toward the base of the petaloid; arrows) from typical orientation. This suggests that the secondary branches are free and, under specific taphonomic settings, can come to rest in the opposite direction. Diameter of Australian $1 coin=2.5 cm. Stalk, stem, and disc not preserved. Scale bars=1 cm or 1 cm increments.

Figure 8 SAM P35703a, b from Nilpena Farm. (1) Part (P) and (2) counterpart (CP) of SAM P35703a, b with peapod-shaped primary branches and distinct central ridges representing primary branching stalks. (3) Side profile of primary branches indicating minimal frond thickness. White arrow indicates branch imbrications/overlap. (4) Branch overlapping resulting from displacement of a primary branch (white arrow). (5) Side profile demonstrating lobate distal terminations of primary branches and absence of a rim. All scale bars=1 cm.
1959 Rangea arborea Glaessner in Reference Glaessner and DailyGlaessner and Daily, pls. 43.1–3, 44.1–3, 45.1, 2.
1966 Arborea arborea Reference Glaessner and WadeGlaessner and Wade, p. 623, pl. 102, figs. 1, 2.
1978 Charniodiscus arboreus; Reference Jenkins and GehlingJenkins and Gehling, p. 350, fig. 3.
1978 Charniodiscus oppositus; Reference Jenkins and GehlingJenkins and Gehling, p. 204, pl.3, fig. 4.
2013 Charniodiscus sp.; Reference Gehling and DroserGehling and Droser, p. 449, fig. 2c.
Type species
Rangea arborea Glaessner in Glaessner and Daily, Reference Glaessner and Daily1959.
Diagnosis
New. Frond with ovate bifoliate petalodium composed of prominent stalk and multiple parallel primary branches stemming between 45° and 90° from stalk. Petalodium tapers distally. Primary branches sinusoidal to rectangular. Primary branching stalk runs the entire length of the branch. Secondary branches oval, broaden distally and taper proximally, where they are anchored to the primary branching stalk. Secondary branches lack branching subdivisions.
Occurrence
Ediacaran of Russia, Ukraine, Australia, England, China, and Canada.
Arborea arborea Glaessner in Glaessner and Daily, Reference Glaessner and Daily1959
1959 Rangea arborea Glaessner in Reference Glaessner and DailyGlaessner and Daily, pls. 43.1–3, 44.1–3, 45.1, 2.
1966 Arborea arborea Reference Glaessner and WadeGlaessner and Wade, p. 623, pl. 102, figs. 1, 2.
1978 Charniodiscus arboreus; Reference Jenkins and GehlingJenkins and Gehling, p. 350, fig. 3.
1979 Charniodiscus arboreus; Reference GlaessnerGlaessner, p. 100, fig. 12.2c.
1996 Charniodiscus arboreus; Reference JenkinsJenkins, p. 36, fig. 4.2a, b, 4.3.
2001 Charniodiscus arboreus; Reference Narbonne, Dalrymple and GehlingNarbonne, Dalrymple, and Gehling, p. 26, pl. 1d.
2004 Charniodiscus arboreus; Reference Laflamme, Narbonne and AndersonLaflamme, Narbonne, and Anderson, p. 832, fig. 4.5.
2013 Charniodiscus sp.; Reference Gehling and DroserGehling and Droser, p. 449, fig. 2c.
Holotype.– P12891, South Australian Museum.
Description
Holotype (P12891) incomplete. Petalodium incomplete, 15 cm long by 5.5 cm wide, with at least 20 primary branches. Probable secondary branches preserved on isolated branches. Stem and holdfast not preserved.
Remarks
Circular discs closely associated with stems and fronds (Fig. 2) are interpreted as rooting holdfasts. In relatively low-energy settings, frond specimens are typically attached to an anchoring disc (Laflamme et al., Reference Laflamme, Narbonne and Anderson2004). However, the higher-energy setting of Ediacara, South Australia, results in the overwhelming majority of circular holdfasts being disassociated from their respective fronds, having been presumably plucked away from the sediment, leaving distinct sedimentary structures in their place (Tarhan et al., Reference Tarhan, Gehling and Droser2010). Furthermore, several instances of surface expressions of collapsed craters with stalks likely represent deflated or collapsed holdfasts (Tarhan et al., Reference Tarhan, Gehling and Droser2010). Holdfasts were bulbous in shape, resulting in a prominent surface expression in addition to a significant portion of the holdfast that lay beneath (or within) the microbial mat, preferentially biasing its preservation over the complete frond (Laflamme et al., Reference Laflamme, Schiffbauer, Narbonne and Briggs2011). To date, no known trace fossils have been found directly associated with the discs (although see Liu et al., Reference Liu, McIlroy and Brasier2010; Menon et al., Reference Menon, McIlroy and Brasier2013), so whether the frond was capable of burrowing with its rooting holdfast is difficult to speculate.
The stem in Arborea (Figs. 1, 2.2) is typically flattened and rectangular in most specimens, although it is interpreted as being originally cylindrical in shape. The stem was most likely composed of a structurally rigid material that restricted compaction during fossilization (Laflamme et al., Reference Laflamme, Narbonne and Anderson2004). In South Australia, frond stems are uncommon but represent the basal extension of the stalk (Fig. 2.1).
The stem continues into the petalodium to form the stalk (Fig. 1). The stalk varies in shape, from strongly parallel-sided (Fig. 3.1) to an alternating, sinuous (zigzagging) central axis (Fig. 3.2–3.4). The stalk is typically preserved in positive relief, suggesting it may be constructed of similar material as the stem. The variation in stalk morphology has been suggested as a means of taxonomically differentiating Arborea species (Jenkins and Gehling, Reference Jenkins and Gehling1978), although reevaluation of specimens from South Australia reveals a seamless transition between specimens representing a parallel-sided cylindrical stalk and those with a sinuous, alternating stalk (Fig. 3.5). In specimens with a parallel-sided stalk (Fig. 3.1), the primary branches appear to terminate at the stalk boundary, whereas in specimens with a sinuous stalk, the primary branches go beyond the boundary of the central stalk and meet at the central axis (Fig. 3.2). In these cases, the outer margin of the central stalk appears sinuous in shape due to the alternating branching pattern along the central axis. It is possible that the cylindrical and sinuous stalks represent the dorsal and ventral aspects of the fronds, respectively. This hypothesis would imply that the anchoring points of the primary branches, whether they be found on the ventral or dorsal side, are only preserved when the respective side of the frond is preserved. This may be the case in some instances; however, specimen P40444 (Fig. 3.4) showcases an alternative interpretation. This specimen shows a transition between end members: a cylindrical (Fig. 3.4A) to a sinuous (Fig. 3.4C) stem. We propose that the difference in stalk shape could represent a taphonomic artefact resulting from compression of the cylindrical stem and the composite molding of the alternating primary branching stalks.
The primary branches are attached to the central stalk, while the smaller secondary branches stem perpendicularly from the primary branch stalk (Figs. 1, 4). Reported tertiary branches are typically poorly preserved and likely represent a taphonomic artefact resulting from wrinkling or folding of soft tissue. Presumed well-preserved examples of secondary branching lack any evidence of tertiary branching, and instead suggest a smooth, teardrop shape for the secondary branches (Figs. 5–7). Primary branches in Arborea are strongly parallel along their entire length, suggesting that they are attached to each other or to a dorsal sheet (Fig. 4.6) or strongly anchored to the stalk proximally, and distally connected to an outer rim (Fig. 1.1; although see Fig. 8 and discussion in the following). The primary branches vary in shape, from cylindrical (Fig. 4.1) to sinuous with strongly tapering proximal and distal terminations (Fig 4.6).
Secondary branches vary in shape, from straight to slightly curved structures emanating upward (rarely downward) from the primary branches (Figs. 5–7). In rare instances, the secondary branches are preserved as teardrops with the tapering end serving as the attachment structure to the primary branch stalk (Figs. 5.5, 6.2–6.4). Attachment of the secondary branches is limited to their proximal region only, allowing for the distal portions of the branches to be displaced and overlap with adjacent branches (black arrow in Fig. 6.3). In some instances, the distal portions of the secondary branches continue far beyond the boundaries of their primary branches and are preserved overlying the proximal portion of the (distally) adjacent primary branch (Figs. 5.2, 7.1–7.3). This implies that secondary branches are not contained within the primary branches like peas in a pod (as suggested by Jenkins, Reference Jenkins1996; Laflamme and Narbonne, Reference Laflamme and Narbonne2008a). Finally, secondary branches can pivot upward (most-typical orientation) and downward (less-typical orientation; Fig. 7).
Arborea in South Australia always possesses a bifoliate petaloid. A thick outer rim is typically preserved (Fig. 1.1); however, the rim can be indented and not uniformly preserved along the entirety of the petalodium (Figs. 1.1, 4.6) or even completely lacking. Specimens that preserve the distal termination of the primary branches are rounded (Fig. 8) and seemingly lack an attachment rim. These specimens suggest that the ‘rim’ most likely represents a preservational artefact resulting from the folding or rolling-up of the soft-bodied primary branches at their distal tips.
Comparisons
Arborea is most similar to Charniodiscus in construction, with both genera sharing an ovate petalodium attached to a stem and anchored to the substrate by a circular to bulbous holdfast. Arborea can be distinguished from Charniodiscus concentricus by the bifoliate petalodium and the smaller number of primary branches that are more uniform in size and shape.
Reconstruction of Arborea
A detailed reconstruction of Arborea is possible by comparing the best-preserved two-dimensional molds of Arborea with the newly discovered three-dimensional specimens. The most important change proposed by the reevaluation of Arborea concerns the morphology of the petalodium. The morphology of the stalk varies from a smooth, featureless rectangle to a zigzagging central axis, with numerous examples forming a transition between end members (Fig. 3). Cylindrical stalks are believed to represent instances in which the stalk was quickly filled with sediment thus resisting compaction or, alternatively, was buried rapidly and therefore did not undergo significant decay prior to burial, which would have compromised the strength of the integument. This morphotype typically lacks the preservation of the anchoring points of the primary branch stalks, presumably due to the difficulty in preserving composite molds when the stalk is sand-filled. The sinuous stalk is believed to represent the compaction of the stalk and the resulting composite molding of the alternating primary branch stalks as they overprint the central stalk (Fig. 3.5).
The primary branches also vary greatly in shape due to different degrees of preservation. For example, the gradation from rectangular primary branches that tend to lack secondary branches (Fig. 4.1) into sigmoidal branches with well-defined secondary branches (Fig. 4.4–4.6) can be explained through taphonomic variation. Cylindrical branches are typically associated with specimens in which the central stalk is also cylindrical (rather than sinuous). The strongly positive relief and well-defined boundaries of the stalk cut off the proximal portion of the branches, resulting in a more rectangular primary branch (Figs. 3.1, 4.1). By contrast, sinuous primary branches are typically found associated with zigzagging central stalks (Figs. 3.2–3.3, 4.5). As such, the sigmoidal shape of the primary branches most likely results from the preservation through composite molding of the alternating proximal attachment to the stalk.
The presence or absence of secondary branches is typically explained as a taphonomic variation, with well-preserved specimens displaying secondary elements (Jenkins and Gehling, Reference Jenkins and Gehling1978; Laflamme et al., Reference Laflamme, Narbonne and Anderson2004). Detailed study of numerous three-dimensional specimens preserving the secondary branches may offer an additional explanation. As demonstrated in Figure 6, the primary branches are sausage-shaped and lack secondary branching. However, when the most distal branch is broken away (Fig. 6.2), a series of secondary branches presumably lying under the overlying primary branch are exposed. Secondary branches can be seen in their entirety as downwardly directed and teardrop-shaped structures (Fig. 6.2–6.4). As secondary branches are basally anchored to the primary branch stalk, which forms a midline in the center of the primary branch, the distal ends of secondary branches come to rest overtopping subsequent primary branches (Fig. 5.1–5.2), similar to overlapping shingles on a house. It is proposed that primary branches lacking secondary branches represent the dorsal side of the petalodium, and that secondary branch preservation is dependent on which side of the frond is cast. A second specimen preserved as both part and counterpart showcases the featureless, presumed dorsal side of the petalodium in addition to the secondary branches. In Figure 7, the proximal portions of the primary branches lack any morphological detail until a break in the distal portion of the rock (arrow in Fig. 7.1 CP) reveals the ventral side of the primary branch, complete with well-defined secondary branches (Fig. 7.4). This apparent dorsal–ventral differentiation of the petalodium represents the simplest explanation for the preservation displayed in the three-dimensional specimens.
Restricting the secondary branches to a single side of the petalodium would have had significant consequences on the functional morphology of Arborea. It has been previously demonstrated that Ediacaran fronds in which the primary branches are all attached to one another (or to a sheet) could not have functioned in the same way as modern pennatulacean sea pens, which require a constant water flow between the primary branches to feed (Seilacher, Reference Seilacher1992; Williams, Reference Williams1997). Lacking the free-flow system between the primary branches, water currents would have reclined the fronds to varying degrees (Singer et al., Reference Singer, Plotnick and Laflamme2012), with the stems allowing for structural rigidity and preventing the petaloids from being toppled. Densely packed frond meadows would also have led to a canopy-flow regime and favored upward growth and greater sizes (Ghisalberti et al., Reference Ghisalberti, Gold, Laflamme, Clapham, Narbonne, Summons, Johnston and Jacobs.2014). Furthermore, as the secondary branches were not attached to one another, currents were able to flow between them (rather than between the primary branches). This feeding mechanism is similar in many respects to modern pennatulacean feeding that requires water flow between the primary polyp leaves; however, in the absence of any visible tentacles or other food-capturing structures, it is difficult to interpret how food capture was achieved in Arborea. Tiering is presumed to have mostly been achieved through differences in the length of the stem, which would have been significantly sturdier than the petalodium, and may have been a driving force in Ediacaran speciation and evolution (Laflamme et al., Reference Laflamme, Narbonne and Anderson2004; Laflamme et al., Reference Laflamme, Flüde and Narbonne2012).
Conclusions
Newly discovered three-dimensional specimens of Arborea arborea from Nilpena Farm in the Flinders Ranges in South Australia allow for the reinterpretation of the morphology and function of this Ediacaran frond. The bifoliate petalodium and distinct branching morphology allow for Australian specimens of Charniodiscus arboreus to be reassigned to Arborea arborea.
Combined with well-preserved two-dimensional specimens, it is possible to isolate the morphological variation associated with the taphonomic process responsible for the preservation of soft-bodied Ediacara fossils and allow for a reconstruction of Arborea that can account for the variation in morphology between various taphomorphs. Arborea-type branching architecture highlights teardrop-shaped secondary branches on one side of the petalodium that were most likely the primary loci for nutrient absorption. Secondary branches were not attached to one another, which allowed for water to flow between these branches. Since the primary branches were likely stitched together or attached to a sheet, an epifaunal, recumbent life habit is implied for these fronds, allowing water currents to travel along the entire length of the petalodium where secondary branches could have absorbed dissolved organic nutrients directly from the water column.
Acknowledgments
Support to Laflamme was provided by the Natural Sciences and Engineering Research Council of Canada Discovery Grant (RGPIN 435402). Several key specimens were discovered by SA Museum volunteers M. Fuller, D. Rice, C. Armstrong, J. Perry, and S. Lange. Field work was supported by an Australian Research Council Discovery Grant (DP0453393) to Gehling. Excellent reviews were provided by A. Liu and an anonymous reviewer.