Introduction
The sustainable intensification of land-use practices has never been more important to ensure the food security of a growing world population. Currently, the global challenge is to increase food production for an increasingly growing population while preserving the environment (Gu et al., Reference Gu, Zhang, Bai, Fu and Chen2019). Meeting this demand without cultivating new land and without degrading existing land is a key point for sustainable agriculture (Reis et al., Reference Reis, Kamoi, Latorraca, Chen, Michetti, Wruck, Garrett, Valentim, Rodrigues and Rodrigues Filho2019).
Within this context, the integrated crop–livestock system is a production strategy that consists of the diversification and integration of different production, agricultural and livestock systems within the same area while striving for environmental preservation and meeting the global demand for food. When done under careful management, the integration of crops in no-till systems can benefit from the complex synergism between soil, plants and animals due to its unique characteristics of diversification of activities and promotion of complex functions of the agroecosystem, which ensure greater productivity in smaller areas, with greater profitability and sustainability (Carvalho et al., Reference Carvalho, Barro, Barth Neto, Nunes, Moraes, Anghinoni, Bredemeier, Bayer, Martins, Kunrath, Santos, Carmona, Barros, Carvalho, Peterson, Nunes, MartinS, Souza Filho, Bertolazi, Kunrath, Moraes and Anghinoni2018).
Among integrated systems, forage succession is an advantageous technique for the use of pasture to feed cattle during the dry season, after which biomass is formed for the no-till system for the next crop (Naudin et al., Reference Naudin, Bruelle, Salgadi, Penot, Scopel, Lubbers, Riider and Giller2015; Andrade et al., Reference Andrade, Borghi, Bortolon, Bortolon, Camargo, Avanzi, Guarda, Cunha, Silva and Fidelis2020). The amount of biomass and the long half-life of the forages deposited on the soil surface contribute to water retention and reduce losses by evapotranspiration. In this way, the persistence of biomass in the soil is a determining factor that itself depends on climatic conditions and plant species (Zagato et al., Reference Zagato, Araujo, Santos, Ludkiewicz, Silveira and Santos2018). High temperature and humidity accelerate the biomass decomposition process on the soil surface (Costa et al., Reference Costa, Costa, Assis, Santos, Severiano, Rocha, Oliveira, Costa, Souza and Aquino2016).
The availability of nutrients for the successor crop is directly related to their availability in the soil and their rate of release from residues. Understanding the factors and processes that control the cycling of nutrients allows us to synchronize their availability with the demand of the successor crop. However, different crops have different abilities to accumulate nutrients in biomass, releasing them in the soil through the decomposition of crop residues (Oliveira et al., Reference Oliveira, Almeida, Junior, Reis, Souza and Favarin2019).
For the success of the integrated crop–livestock system, it is necessary to choose the forages most adapted to the edaphoclimatic conditions of the region, considering a balance between forage quality and yield. Currently, in Brazil, the most commonly used forage grasses in such systems are those of the genus Brachiaria (Guarnieri et al., Reference Guarnieri, Costa, Severiano, Silva, Oliveira and Santos2019; Silva et al., Reference Silva, Gonçalves, Costa, Flávio Neto, Brito, Silva and Severiano2019), and recently, due to the emergence of new Panicum maximum cultivars, this species has also been used (Machado et al., Reference Machado, Cecato, Comunello, Concenço and Ceccon2017; Dias et al., Reference Dias, Costa, Severiano, Bilego, Furtini Neto, Almeida, Brand and Lourival2020). Thus, it is necessary to determine the potential of new forage species within integrated crop–livestock systems to produce forage in the off-season. We must also consider the benefits that the animal component brings to the system in terms of biomass production and nutrient release, which favour soybean yield and contributes to the sustainability of agricultural systems.
The biomass produced by perennial tropical forages promotes large stocks of organic matter in the soil, which in turn contributes to nutrient cycling, especially nitrogen and carbon replacement and water maintenance, and improves soil properties (Ryschawy et al., Reference Ryschawy, Martin, Moraine, Duru and Therond2017). In contrast, the mulch biomass produced by maize grown in succession to soybean is inefficient for good soil cover and nutrient cycling for the subsequent soybean crop.
Therefore, the objective of this study aimed to evaluate soybean yield in an integrated crop–livestock system in comparison to soybean–maize succession system through the production, decomposition and nutrient accumulation in the biomass.
Materials and methods
Description of the area
This study was conducted at the Institute of Science and Technology Comigo in Rio Verde, State of Goias-GO, Brazil, from November 2017 to February 2019, at the coordinates 17°45′48″S and 51°02′14″W and at an altitude of 832 m. The relief of this region is not very wavy and helps to explain the advance of mechanized and intensive agriculture. The study area presented a 6-year history in the crop–livestock integrated under no-tillage system, with annual crops (soybean and maize) and forage for biomass formation (Brachiaria brizantha, Brachiaria ruziziensis and P. maximum).
The soil was classified as a typical Dystrophic Red Latosol according to the Brazilian classification system (Santos et al., Reference Santos, Jacomine, Anjos, Oliveira, Lumbreras, Coelho, Almeida, Araujo Filho, Oliveira and Cunha2018). The physical and chemical characterization of the soil in the 0–20 cm was carried out before the experiment was implemented, presenting clay, 351 g/kg; sand, 539 g/kg; silt, 110 g/kg; pH in CaCl2: 4.9; Ca: 2.5; Mg: 0.74; Al: 0.1; Al + H: 5.0; K2O: 0.43; cation exchange capacity: 8.6 in cmolc/dm3; V: 41% and P (mehlich): 33.7; B: 0.74; Cu: 1.2; Zn: 6.3; Cu: 1.23; Fe: 53.8 in mg/m3; organic matter: 27.18 g/kg.
First soybean crop establishment (2017/2018)
The soybean sowing was done on 11/07/2017 and the variety used was M7110PRO, with 0.50 m line spacing. For seed treatment, 200 ml of Standak Top was used for each 100 kg of seeds. Before the sowing, 1 t/ha of limestone and 1 t/ha gypsum were applied. The seed and monoammonium phosphate (MAP) were mixed together at a dose of 250 kg/ha (25 kg/ha de N and 115 kg/ha de P2O5) after which the mixtures were distributed over their respective areas via a Lancer spreader. At 15 days after sowing (DAS) 80 kg/ha of K2O was applied as potassium chloride.
The fungicide applications were performed at 40 DAS (dose of 0.3 litres/ha of Orkestra and 0.5 litres/ha of Assist), 60 DAS (dose of 0.2 kg/ha of Elatus and 0.6 litres/ha of Nimbus), 75 DAS (dose of 0.4 litres/ha of Fox and 0.3 litres/ha of Aureus) and 85 DAS (dose of 0.3 litres/ha of Orkestra and 0.5/ha of Assist). Soybean was harvested in February 2018, with an average production of 4339 kg/ha.
Statistical design, treatments and establishment of crops
The experimental design was a randomized complete block design with four replicates. The treatments consisted of the following cropping systems: integrated crop–livestock with Paiaguas palisadegrass (B. brizantha cv. BRS Paiaguas), integrated crop–livestock with Tamani guinea grass (P. maximum cv. BRS Tamani) and maize grown in succession to soybean.
The forages were implanted in succession to soybean on 28 February 2018, using 5 kg/ha of seeds for Paiaguas palisadegrass and 3.5 kg/ha for Tamani guinea grass, with 60 and 40% of cultural value (determines the germination and purity of the seed), respectively. The seeds were sown in the respective areas with the aid of the seed drill, with 0.45 m of spacing between lines. The area of each forage plot was 0.24 ha.
The monoculture maize was sown on 1 March 2018 using the hybrid AG 7098 PRO2, in an area of 0.24 ha, aiming to reach a final population between 250 and 300 thousand plants per ha with base fertilization of 300 kg/ha of the fertilizer formulation 08-20-18 (N-P-K) applied in the furrow of planting. In the phenological stage V6, 90 kg/ha of N (urea source) was applied. The harvest of maize for grain was carried out at 156 days, on 3 August 2018, with an average production of 4200 kg/ha.
Animal performance
After the development of the forages, at 84 DAS, cattle of the Nelore breed were inserted in the area, with an average age of 13.2 ± 0.7 months, with an average initial body weight of 239.43 ± 29.98 kg. For animal performance, the animals entered the area in the month of May and were removed in the month of August, remaining for 3 months, in rotating pasture, being 7 days of occupation and 28 days of rest for regrowth of forage. The rate of occupancy was variable and adjusted, whenever necessary, throughout the experiment, according to the availability of forage. The average stocking rate was 2.71 and 2.25 UA/ha (animal unit) for Paiaguas palisadegrass and Tamani guinea grass, respectively.
The animals were removed from the area on 23 August 2018 and the forages were at rest for 41 days for regrowth (development), for subsequent desiccation (dead) to form biomass for soybean sowing. Desiccation was performed on 25 September 2018 with the application of glyphosate herbicide in a dose of 4 litres/ha (522 g/l of i.a.), with a syrup volume of 150 litres/ha.
Assessment of mulch biomass and total nutrient
To quantify the biomass production from Paiaguas palisadegrass, Tamani guinea grass and maize, eight samples were collected from a 1 m2 area randomly selected within each plot. The grasses were cut 21 days after desiccation and the maize 67 days after the grain harvest. The plant material was cut at ground level. The cut material was weighed, the samples were placed in a forced-air oven at 55°C until they reached a constant weight, and were transformed into kg/ha.
Mulch decomposition was evaluated for dead forage material collected in 25 × 30 cm litter bags made of a 2 mm mesh (Thomas and Asakawa, Reference Thomas and Asakawa1993). These bags, containing biomass samples of each species in amounts proportional to the dry mass produced per hectare, were placed in direct contact with the soil. For each time (30, 60, 90 and 120 days), four bags were used per treatment.
At 30, 60, 90 and 120 days after the bags were placed on the soil, one litter bag was removed from each plot to evaluate the remaining amount of mulch biomass and to determine mulch decomposition throughout the 120-day period (which ended at soybean harvest). On the basis of the initial mass (kg/ha) in the systems, the loss percentage was calculated as the ratio of the mass of the litter bags (kg/ha) to the initial production.
At each evaluation time point, the material was subsequently sent to the laboratory. The soil debris was removed by washing the material with running water until all the debris was gone, after which the material was dried in an oven at 55°C until it reached a constant weight, according to the methods of Costa et al. (Reference Costa, Costa, Severiano, Santos, Rocha, Souza, Brandstetter and Castro2017). The biomass samples were ground (2 mm) in a mill to determine the carbon (C), nitrogen (N), phosphorus (P), potassium (K), calcium (Ca), magnesium (Mg) and sulphur (S) contents according to the methods of Malavolta et al. (Reference Malavolta, Vitti and Oliveira1997). The C/N ratio of the material was subsequently calculated. To evaluate the nutrient contents, the macronutrient concentrations were multiplied by the dry mass production, and the results were expressed as kilograms per hectare.
The N, P2O5 and K2O fertilizer equivalents of the mulch biomass of the forage and maize cropping systems were determined considering the atomic mass of the elements according to analytical chemistry conventions and the N, P and K concentrations of the biomass analysed (Dias et al., Reference Dias, Costa, Severiano, Bilego, Furtini Neto, Almeida, Brand and Lourival2020).
Establishment of soybean in the 2018/2019 crop and evaluation of agronomic characteristics
The soybean (variety M7739) seed was sown on 20/10/2018 in rows spaced 0.50 m apart in the biomass of Paiaguas palisadegrass, Tamani guinea grass and maize. At the time of sowing, the soybean was inoculated with Gelfix (0.6 litres/ha) and fertilized with 100 kg/ha of P2O5 monoammonic fertilizer (MAP) and 100 kg/ha FTE BR 12 (Mo + B + Zn + Cu + Mn) applied in the planting furrow. At 15 DAS, 80 kg/ha of K2O was applied (potassium chloride source).
The fungicide applications were performed at 40 DAS (dose of 0.3 litres/ha of Orkestra and 0.5 litres/ha of Assist), 60 DAS (dose of 0.2 kg/ha of Elatus and 0.6 litres/ha of Nimbus), 75 DAS (dose of 0.4 litres/ha of Fox and 0.3 litres/ha of Aureo) and 85 DAS (dose of 0.3 litres/ha of Orkestra and 0.5 litres/ha of Assist).
The evaluations of the agronomic characteristics of soybean were performed on 15 February 2019 (119 DAS), on the day of harvest. Ten plants distributed in the useful area of the plot were evaluated: height of plants (measured as the distance between the soil surface and the apical end) and insertion height of the first and last pod (between the soil surface, the insertion of the first and last pod in the main stem), number of pods per plant (counting all the pods with grains in ten plants in the useful area of the plot, calculating the average of pods per plant), weight of 1000 grains and productivity in kilograms per hectare.
During the experiment, the monthly rainfall and average temperature data were recorded (Fig. 1). Figure 2 shows a diagram of the cropping systems comprising Tamani guinea grass and Paiaguas palisadegrass in an integrated crop–livestock system and a system with maize in succession to soybean. This system was designed to diversify activities, maximizing land use throughout the year.

Fig. 1. Monthly rainfall and mean daily temperatures recorded from November 2017 to February 2019 in Rio Verde-GO, Brazil.
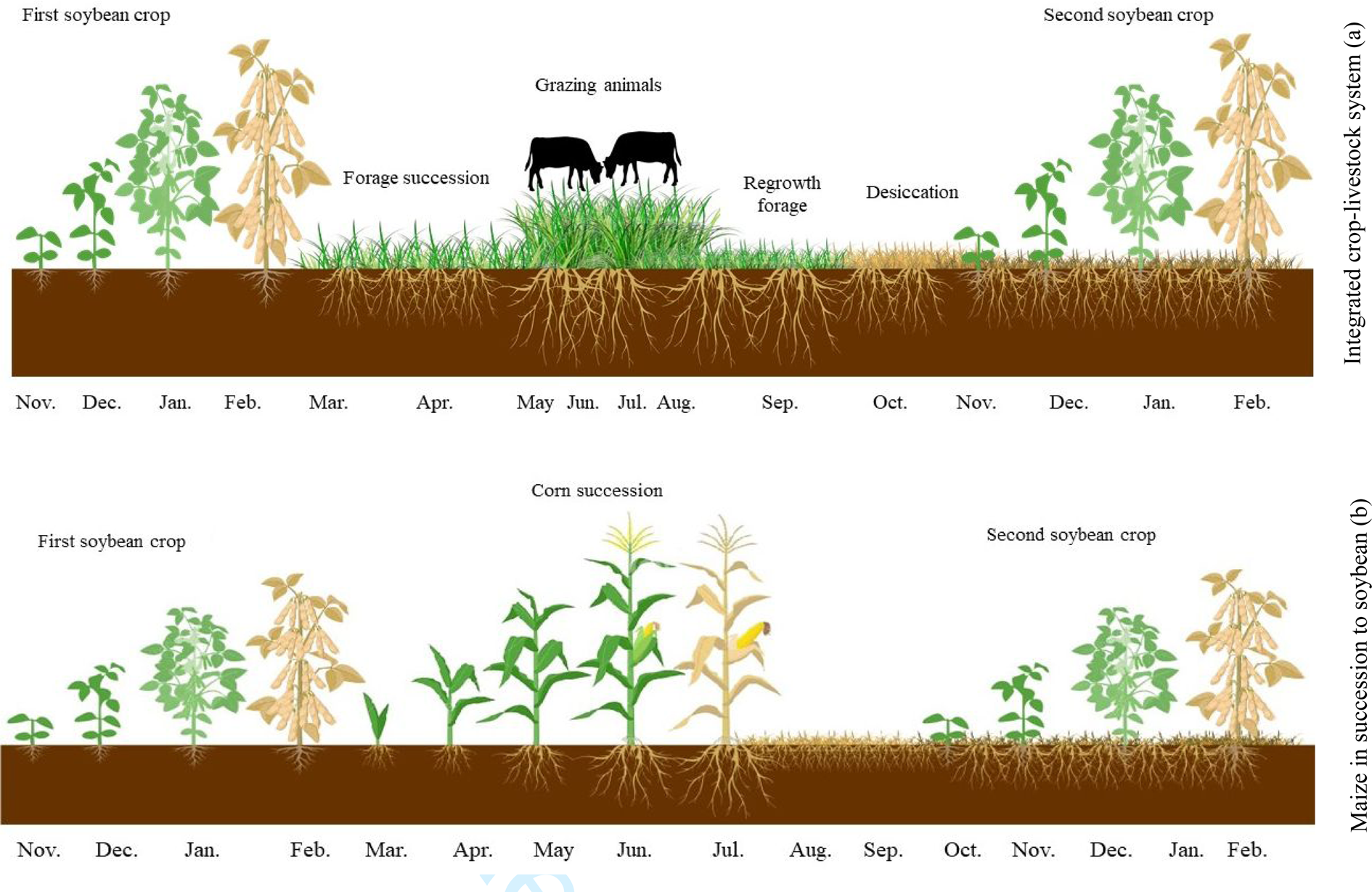
Fig. 2. Colour online. Diagram of the cropping systems comprising Tamani guinea grass and Paiaguas palisadegrass forage species in an integrated crop–livestock system (a) and a system with maize in succession to soybean (b), covering all evaluation stages.
Statistical design and analysis
To describe the decomposition of the mulch biomass and the nutrient contents, the data were fitted together with the standard error to an exponential mathematical model (y = aekx) and, for the C/N ratio, to a linear model (y = a + bx) via Sigma Plot software, where the coefficients ‘a’ of the equations represent the amount of nutrient and C/N ratio accumulated and the angular coefficient ‘b’, the decrease of this ratio. The estimated equations were compared according to a procedure described by Snedecor and Cochran (Reference Snedecor and Cochran1989); this method tests the homogeneity of the data (F) and the significance of the slope (0.4343k) and intercept (log a) of a linear equation (log y = log a + 0.4343kx).
To calculate the half-life (t 1/2), i.e. the time required for 50% of the remaining biomass to be decomposed, the equation proposed by Paul and Clark (Reference Paul and Clark1989) was used, namely, t 1/2 = 0.693/k, where t 1/2 is the half-life of the dry biomass and k is a constant of the dry biomass decomposition.
The data concerning the total nutrient, fertilizer equivalents, soybean agronomic traits and grain yield were analysed by analysis of variance by the use of R software version 3.1.1 (2014); specifically, the ExpDes package was used (Ferreira et al., Reference Ferreira, Cavalcanti and Nogueira2014). The means were compared according to Tukey's test at a significance level of 5%.
Results
Biomass production, C:N ratio, nutrient concentration and accumulation
There was a significant effect of cropping system on biomass production, remaining biomass, C:N ratio, and nutrient concentration and accumulation (Tables 1 and 2, Figs 3 and 4). The highest amount of biomass on the soil surface at the time of soybean sowing was obtained from Paiaguas palisadegrass (4898 kg/ha), followed by Tamani guinea grass (4302 kg/ha). Maize had the lowest biomass production (3409 kg/ha). This same pattern was observed for the remaining biomass until the final soybean development cycle (Fig. 3(a)). At the end of the evaluations, the total remaining residues were 1498, 1076 and 930 kg/ha for Paiaguas palisadegrass, Tamani guinea grass and maize, respectively, with biomass losses of 70.7, 74.4 and 72.7% in the final soybean development cycle. On the other hand, according to the comparison between the regression equations for decomposition rate and C:N ratio (Snedecor and Cochran, Reference Snedecor and Cochran1989), Table 2 showed significance for the angular coefficient (b), with values for maize > Tamani guinea grass > Paiaguas palisadegrass.
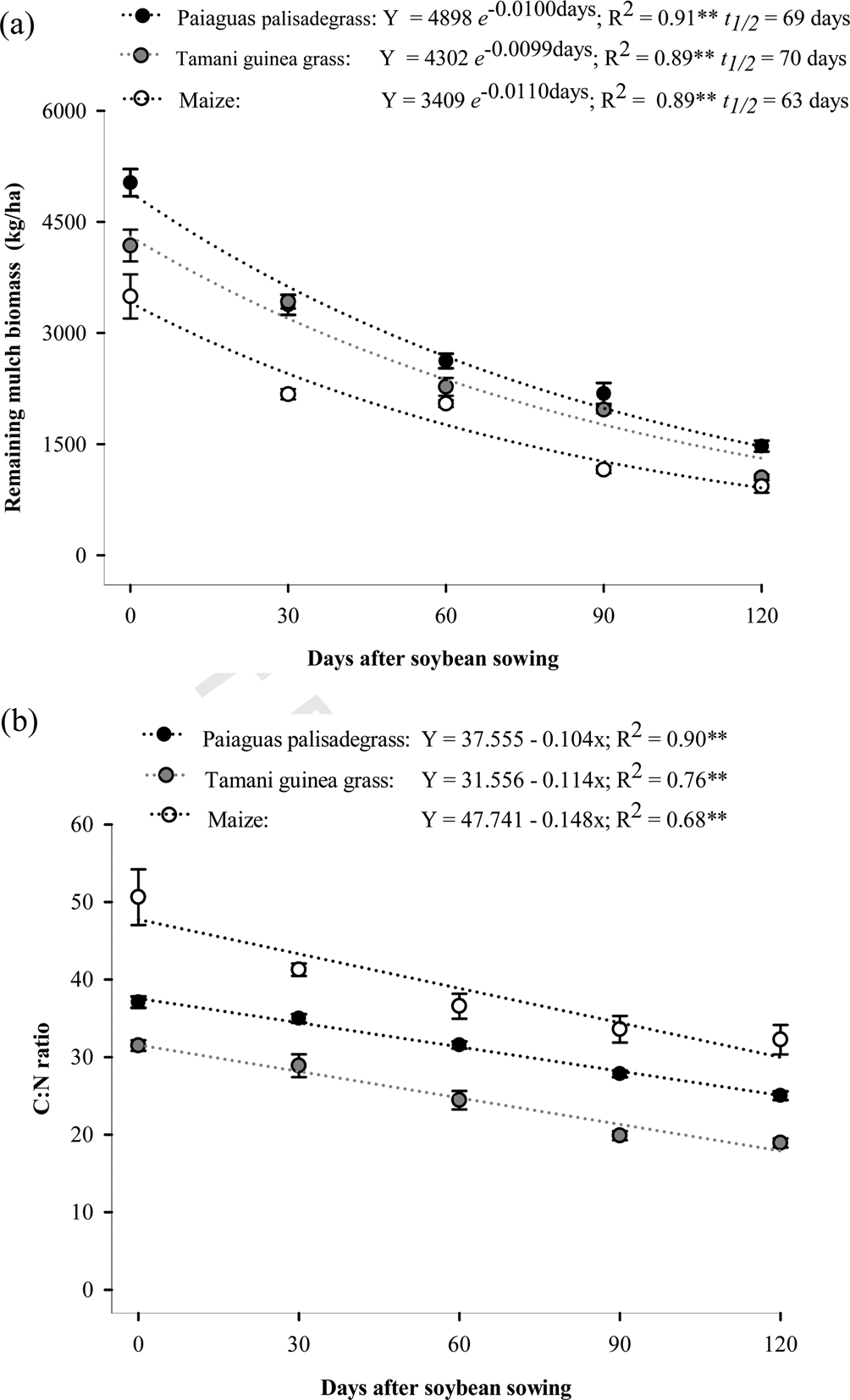
Fig. 3. Remaining biomass (a) and C:N ratio (b) of Paiaguas palisadegrass, Tamani guinea grass and maize during soybean cropping (0–120 days). Error bars represent ±standard error of means. **Significant at 1%.

Fig. 4. Accumulation of nitrogen (a), phosphorus (b), potassium (c), calcium (d), magnesium (e) and sulphur (f) in the biomass of Paiaguas palisadegrass, Tamani guinea grass and maize during soybean cropping (0–120 days). Error bars represent ±standard error of means. **Significant at 1%.
Table 1. Initial biomass production and nutrient concentration of Paiaguas palisadegrass and Tamani guinea grass in an integrated system of maize in succession to soybean

s.e.m., standard error of mean.
Means followed by different letters differ by Tukey's test at 5% probability.
Table 2. Comparison of linear regressions of remaining biomass (log y = log a + 0.4343kx) and C:N ratio of different cropping systems with Paiaguas palisadegrass, Tamani guinea grass and Maize, according to Snedecor and Cochran (Reference Snedecor and Cochran1989)

NH, non-homogeneous; H, homogeneous; ns, non-significant.
a F tests data homogeneity.
*Significant at 5%; **Significant at 1%.
The half-lives of the Paiaguas palisadegrass and Tamani guinea grass mulch biomasses were similar, for the Paiaguas palisadegrass it was 69 days and for Tamani guinea grass it was 70 days, whereas for maize the half-life was shorter (63 days) (Fig. 3(a)). There was an influence of the cropping system on the C:N ratio (Fig. 3(b)). At all evaluated times, maize had a higher C:N ratio than the forage plants, and its initial value was 47.74 (Fig. 3(b)).
For the initial concentration of nutrients, forages showed higher values for nitrogen, phosphorus, potassium, calcium, magnesium and sulphur, differentiating from maize with a lower value (Table 1). The nitrogen and potassium obtained in the biomass of forages were the nutrients with the greatest prominence in relation to the difference in concentration in the maize biomass. There was an exponential reduction in nutrient accumulation for all biomasses of the cropping systems (Fig. 4).
Nitrogen and phosphorus accumulation after 30 days were similar between the forages but was lower in the maize group at all decomposition times. Comparing the initial nitrogen and phosphorus accumulations with the values reached after 120 days of management, a release of 80.5, 81.4 and 75.3% nitrogen and 94.1, 96.6 and 97.7% phosphorus was observed for Paiaguas palisadegrass, Tamani guinea grass and maize, respectively.
Paiaguas palisadegrass and Tamani guinea grass at 0, 30, 60 and 90 days showed higher potassium accumulation than maize (Fig. 4(c)). At 120 days, the values were similar between the cropping systems: Paiaguas palisadegrass, Tamani guinea grass and maize released 94.1, 96.6 and 97.7% potassium, respectively.
For calcium, magnesium and sulphur, the highest nutrient accumulations at 30–120 days were obtained in Paiaguas palisadegrass, followed by Tamani guinea grass, with maize having the lowest potential to accumulate these nutrients at all soybean cropping times. At 120 days, Paiaguas palisadegrass, Tamani guinea grass and maize had respective release rates of calcium of 86.5, 84.4 and 83.8%; of magnesium, 81.7, 82.3 and 78.7%; and of sulphur, 85.1, 86.1 and 85.2%.
Of all the nutrients, potassium had the shortest half-life (Paiaguas palisadegrass: 19 days; Tamani guinea grass: 21; maize: 34). Tamani guinea grass yielded the longest half-lives of nitrogen, phosphorus, calcium, magnesium and sulphur, indicating slower release rates. Conversely, the potassium half-life was the longest in maize biomass. The Paiaguas palisadegrass biomass had the shortest half-lives of nitrogen, potassium, calcium and magnesium, indicating faster rates of nutrient release. The maize biomass had the shortest half-lives of phosphorus and sulphur.
Evaluating the fertilizer equivalent, Table 3 shows that Paiaguas palisadegrass and Tamani guinea grass provided greater amounts of nitrogen, phosphorus and potassium equivalents than maize. The release of nutrients from the straw of these grasses has the potential to save 207 kg of urea, 128 kg of single superphosphate and 170 kg of potassium chloride.
Table 3. Equivalent contents of N, P2O5 and K2O in the mulch biomass of different cropping systems

s.e.m., standard error of mean.
Means followed by different letters differ by Tukey's test at 5% probability.
Agronomic characteristics and soybean yield
The soybean plant height and first pod insertion height were similar (P > 0.05) between the biomasses of the cropping systems, with respective means of 64.5 and 14.9 cm (Table 4). The number of pods/plant and number of grains/pod of soybean grown in Paiaguas palisadegrass biomass were similar to those of Tamani guinea grass and higher than those of maize. The weight of 1000 soybeans in the average of the Paiaguas palisadegrass and Tamani guinea grass cultivation systems was 183.8 g, when compared to 157.2 g of the maize cropping system. As for the grain yield, 5217 kg/ha were found in the average of the systems with forages, 12.8% higher than the maize cropping system that obtained 4623 kg/ha soybean yield (Table 4).
Table 4. Plant height, first pod insertion height, number of pods/plant, number of grains/pod, 1000-grain weight, grain yield of soybean grown in biomass of Paiaguas palisadegrass, Tamani guinea grass and maize

s.e.m., standard error of mean.
Means followed by different letters differ by Tukey's test at 5% probability.
Discussion
The higher biomass production of Paiaguas palisadegrass is due to the high potential of this forage to develop even under low rainfall (Fig. 1), because of its vigorous and rapid regrowth. The Paiaguas palisadegrass has thin stems and high productivity (Epifanio et al., Reference Epifanio, Costa, Severiano, Souza, Teixeira, Silva and Aquino2019), providing excellent soil cover (Costa et al., Reference Costa, Costa, Severiano, Santos, Rocha, Souza, Brandstetter and Castro2017). Similar characteristics are also observed in Tamani guinea grass, which is a short-stature forage with a thin and short stem and high forage production (Machado et al., Reference Machado, Cecato, Comunello, Concenço and Ceccon2017; Maciel et al., Reference Maciel, Braga, Guimarães Junior, Ramos, Carvalho, Fernandes, Fonseca and Jank2018; Martuscello et al., Reference Martuscello, Rios, Ferreira, Assis, Braz and Vieira Cunha2019; Dias et al., Reference Dias, Costa, Severiano, Bilego, Furtini Neto, Almeida, Brand and Lourival2020) but has lower drought tolerance than Paiaguas palisadegrass. Studies conducted by Santos et al. (Reference Santos, Costa, Oliveira, Severiano, Costa, Silva, Guarnieri and Silva2016), Guarnieri et al. (Reference Guarnieri, Costa, Severiano, Silva, Oliveira and Santos2019) and Santos et al. (Reference Santos, Costa, Souza, Silva, Silva, Oliveira and Brandstetter2020) showed that Paiaguas palisadegrass is one of the most suitable forages for crop–livestock integration, with satisfactory results in productivity, especially when water is scarce, and is an attractive option for biomass production (Costa et al., Reference Costa, Costa, Assis, Santos, Severiano, Rocha, Oliveira, Costa, Souza and Aquino2016) and nutrient accumulation (Costa et al., Reference Costa, Costa, Severiano, Santos, Rocha, Souza, Brandstetter and Castro2017) for soybean no-till systems (Oliveira et al., Reference Oliveira, Costa, Assis, Severiano, Dias and Santos2020).
During the off-season in this study, the forages were grazed for 84 days under high stocking rates even in the water-scarce period of the year. After removal of the animals, these forages showed rapid regrowth at the beginning of the rainy season, favouring initial biomass production (Table 1), which corresponds to ‘zero time/sowing of soybean’ (Fig. 2) for the soybean no-till system. Another reason for the high biomass production was the soil fertility associated with the deposition of animal excrement by grazing. In integrated systems with tropical forages, it is possible to place animals into the area and utilize their excreta, which constitutes a nutrient return pathway to the soil (He et al., Reference He, Pagliari and Waldrip2016), contributing to soil fertility and changing the dynamics of mineralization. It is also noteworthy that animal grazing stimulates the vigorous regrowth of forages, favouring growth for biomass production (Dias et al., Reference Dias, Costa, Severiano, Bilego, Furtini Neto, Almeida, Brand and Lourival2020).
Grazing animals in an integrated crop–livestock system can modify nutrient and plant residue cycling rates, changing the biochemical form of nutrients, their spatial distribution within the pasture, and their time of release or availability (Assmann et al., Reference Assmann, Martins, Anghinoni, Denardin, Nichel, Costa, Silva, Balerini, Carvalho and Franzluebbers2017) via their return in manure and urine. In this sense, animals act as nutrient recycling agents (Carvalho et al., Reference Carvalho, Anghinoni, Moraes, Souza, Sulc, Lang, Flores, Lopes, Silva, Conte, Wesp, Levien, Fontaneli and Bayer2010), which may result in a lesser need for fertilizer for the next crop and maintenance of production sustainability over time (Costa et al., Reference Costa, Costa, Assis, Santos, Severiano, Rocha, Oliveira, Costa, Souza and Aquino2016).
The lower production of maize biomass (3409 kg/ha) was due to its early decomposition in the off-season, in which the material remained in the soil for a longer time than the forages. However, the biomass produced by maize does not provide good soil cover due to the large amount of stems, resulting in less biomass on the soil and reducing its contact with the surface. In a study of maize biomass production, Ferreira et al. (Reference Ferreira, Braz, Assis, Costa, Silva and Torres2016) measured a biomass production of 6117 kg/ha, with 43% biomass loss relative to the initial biomass.
Even in the final soybean cycle, Paiaguas palisadegrass had an average production of 1498 kg/ha, 39% higher than that of Tamani guinea grass (1076 kg/ha) and 61% higher than that of maize (930 kg/ha), and it remained longer on the soil surface and ensured cover for a prolonged time. This result is relevant and very important in the Cerrado region of Central Brazil, where the high temperatures throughout the year accelerate the decomposition of the material, which hinders the permanence of the biomass on the soil surface (Costa et al., Reference Costa, Costa, Assis, Santos, Severiano, Rocha, Oliveira, Costa, Souza and Aquino2016; Zagato et al., Reference Zagato, Araujo, Santos, Ludkiewicz, Silveira and Santos2018). In addition, this region often has short-term droughts, hindering crop development. With the soil protected by biomass, moisture loss is reduced, ensuring greater stability for production in any climatic conditions.
The high percentages of biomass loss that we observed, 70.7 for Paiaguas palisadegrass, 74.4 for Tamani guinea grass and 72.2 for maize, indicate the amount that was mineralized, with a consequent contribution to the cycling of nutrients and organic matter in the soil. In addition to the benefit of nutrient cycling, biomass favours microbiological activity, improves soil properties, is a source of nutrients and energy for microorganisms (Malhi et al., Reference Malhi, Légère, Vanasse and Parent2018) and leaves the soil covered during the off-season. In the long term, an integrated crop–livestock system promotes greater activity of microorganisms at depth, improves the quality of soil chemical attributes and contributes to carbon accumulation in the soil, leading to total organic carbon values close to those obtained in native Cerrado (Soares et al., Reference Soares, Ramos, Marchão, Maciel, Oliveira, Malaquias and Carvalho2019).
The higher C:N ratio of maize at all evaluated times is explained by the higher proportion of recalcitrant material (Miguel et al., Reference Miguel, Pacheco, Carvalho, Souza, Feitosa and Petter2018), such as stalks, cobs and husks. More lignin is concentrated in these plant structures, giving this material greater resistance to the action and penetration of decomposing microorganisms, which hinders its decomposition. The highest concentrations of carbon among the fractions of the maize plant are usually found in the ears and shanks, followed by leaves, tassels and husks (Barbosa et al., Reference Barbosa, Ferreira, Santos, Motta, Prior and Gabardo2016). Dias et al. (Reference Dias, Costa, Severiano, Bilego, Furtini Neto, Almeida, Brand and Lourival2020) evaluated the biomass and C:N ratio in forages in integrated crop–livestock and second-crop maize systems; they noticed that maize had a greater C/N ratio, but the forage grasses also showed high potential by protecting the soil.
The lower C:N ratios of the forages are due to their higher leaf blade:stem ratios, which promote more intense degradation than in maize. However, both forages had good soil coverage, formed by the litter present in the area. It is worth noting that the possible reason for the reduction in the C:N ratio of residues over time is due to the leaching of nitrogen from the leaves. Herrera et al. (Reference Herrera, De Mello, Apolinário, Dubeux Júnior, Da Silva, Dos Santos and Da Cunha2020), evaluating the decomposition of senescent leaves in Brachiaria decumbens, also found a reduction in the C:N ratio over time.
The decomposition rate of the remaining biomass determines the permanence time of the mulch on the soil, which is influenced by the lignin content and the C:N ratio in the residues. Forage grasses generally produce relatively high amounts of biomass, also characterized by a high C:N ratio, and contribute to a strong persistence of soil cover (Oliveira et al., Reference Oliveira, Almeida, Junior, Reis, Souza and Favarin2019). C:N ratios between 12 and 25 contribute to mineralization, and values greater than 50 contribute to the immobilization of nutrients in the soil. The ideal is the equilibrium between the two processes, that is, a ratio between 25 and 30 (Truong and Marschner, Reference Truong and Marschner2018).
In the final soybean development cycle (120 days), the C:N ratio of Paiaguas palisadegrass was 27.5, and that of Tamani guinea grass was 19.8. These results show that Paiaguas palisadegrass maintained an adequate C:N ratio (above 25), which was closer to immobilization than to mineralization, indicating that the residues are highly recalcitrant. For the Cerrado region of Central Brazil, this result is of paramount importance, as temperatures are typically high, favouring biomass decomposition.
The higher nitrogen accumulation in Paiaguas palisadegrass and Tamani guinea grass can be attributed to the higher biomass production of these forages, as well as to the higher production of leaves in these grasses, where greater amounts of nutrients are concentrated, favouring nitrogen cycling. Another factor that may have contributed to the greater nitrogen accumulation is the return of nutrients through the excretion of manure and urine, increasing nutrient recycling (Carvalho et al., Reference Carvalho, Anghinoni, Moraes, Souza, Sulc, Lang, Flores, Lopes, Silva, Conte, Wesp, Levien, Fontaneli and Bayer2010).
Considering that part of the nitrogen accumulated in the studied biomasses will be deposited in the soil, it can be inferred that the biomass of Paiaguas palisadegrass and Tamani guinea grass contributed significantly to the supply of nitrogen to soybean in the initial phase, a period in which biological nitrogen fixation is not effective. Maize biomass had an initial nitrogen accumulation of 34.7 kg/ha, which is insufficient to meet the demand of soybean. Thus, it is possible that part of the nitrogen accumulated in the maize biomass was incorporated into the soil or lost. Soybean uptakes an average of 190–372 kg/ha of nitrogen, 65–85% of which comes from the symbiotic fixation of atmospheric N2, and the rest is provided by the soil (Oliveira Junior et al., Reference Oliveira Junior, Castro, Oliveira and Klepker2020).
Nitrogen and potassium are the two nutrients most absorbed by forage plants (Costa et al., Reference Costa, Costa, Assis, Santos, Severiano, Rocha, Oliveira, Costa, Souza and Aquino2016, Reference Costa, Costa, Severiano, Santos, Rocha, Souza, Brandstetter and Castro2017), and in the present study, they were found in greater abundance in the Paiaguas palisadegrass and Tamani guinea grass biomasses. The phosphorus, potassium and sulphur accumulation was satisfactory in the biomass of the Paiaguas palisadegrass and Tamani guinea grass cropping systems for the uptake required by soybean as a successor crop, showing that these forages can release amounts close to the crop needs. Calcium and magnesium accumulation in the biomass was lower than the soybean demand. Soybean uptakes were 14–34 kg/ha phosphorus, 95–250 kg/ha potassium, 50–118 kg/ha calcium, 29–46 kg/ha magnesium and 11–19 kg/ha sulphur kg/ha (Oliveira Junior et al., Reference Oliveira Junior, Castro, Oliveira and Klepker2020).
The initial accumulation of all nutrients in the maize biomass was significantly lower than in the Paiaguas palisadegrass and Tamani guinea grass. One of the explanations may be that the maize was harvested 68 days before the first analysis of nutrient concentration in the biomass, a period in which nutrients were lost from the biomass. Despite this initial contrast, during the evaluation period, the differences between cropping systems were considerably low.
Oliveira et al. (Reference Oliveira, Almeida, Junior, Reis, Souza and Favarin2019) evaluated the nutrient cycling of maize intercropped with Brachiaria species and found that the highest nutrient accumulation was recorded for B. brizantha, followed by B. ruziziensis, and that the intercropping between maize and B. brizantha during the regular season was the best option to increase biomass production and nitrogen, phosphorus and potassium cycling.
The amount and release of nutrients from residues of previously cultivated biomass are highly important in nutrient management from the perspective of cycling in production systems. In this sense, the period of greatest plant demand should coincide with the period of greatest nutrient release from the biomass. As a way to rationalize nutrient management, the contribution of biomass to nutrient supply should be considered when calculating the fertilizer dose to apply (Santos et al., Reference Santos, Albuquerque Filho, Vilela, Ferreira, Carvalho and Viana2014). High biomass production may lessen the need to add nutrients from external sources, reducing production costs and potentially reducing nutrient losses due to denitrification or leaching (Soares et al., Reference Soares, Ramos, Marchão, Maciel, Oliveira, Malaquias and Carvalho2019).
The highest macronutrient accumulation in soybean occurs between 82 and 92 days, and the highest absorption rate occurs between 39 and 58 days (Malavolta et al., Reference Malavolta, Vitti and Oliveira1997). In the present study, the half-life of nutrients was on average 46 days, except for potassium, and greater release of nutrients was observed up to 85 days, showing the potential of grasses as a reserve and supply of nutrients for successor crops.
The longer half-life of phosphorus is due to the large amount of it that is associated with the structural components of plants (Malhotra et al., Reference Malhotra, Vandana Sharma and Pandey2018), and its release depends on the microbial decomposition of the residue. In an evaluation of phosphorus cycling in an integrated crop–livestock system, Assmann et al. (Reference Assmann, Martins, Anghinoni, Denardin, Nichel, Costa, Silva, Balerini, Carvalho and Franzluebbers2017) found faster phosphorus release under an intensive grazing system because the greater amount of cattle manure changes the dynamics of soil microbiological activity. Grazing can be considered a regulator of labile phosphorus, and the effect of this process depends on pasture consumption and urine and manure recycling.
Potassium has a shorter half-life because it is an element that is not part of any structure or organic molecule in the plant, rather being predominantly found as free cations with high mobility in plants, which are easily washed away by rainwater after the rupture of plasma membranes (Taiz et al., Reference Taiz, Zeiger, Møller and Murphy2017). Thus, potassium is readily available and released with little dependence on microbial processes (Assmann et al., Reference Assmann, Martins, Anghinoni, Denardin, Nichel, Costa, Silva, Balerini, Carvalho and Franzluebbers2017). Miguel et al. (Reference Miguel, Pacheco, Carvalho, Souza, Feitosa and Petter2018) observed accelerated release of potassium from the biomass to the soil, especially in the first 30 days.
The accumulation of nutrients and the half-lives of calcium, magnesium and sulphur show the importance of nutrient cycling by Tamani guinea grass and Paiaguas palisadegrass for the supply of these nutrients to the soybean crop. Sulphur and magnesium are released into the soil faster than calcium because they are part of sulfonated amino acids. Conversely, calcium is a predominant constituent of calcium pectates in plant tissue. Pectates are more insoluble and take longer to be decomposed by microorganisms (Buchelt et al., Reference Buchelt, Teixeira, Oliveira, Rocha, Prado and Caione2020).
The order of nutrient accumulation of the remaining biomass of Paiaguas palisadegrass and Tamani guinea grass was N > K > Ca > S > Mg > P. For maize biomass, it was N > K > S > Ca > P > Mg. The nutrients with the highest accumulated concentrations in the biomass were nitrogen and potassium. Phosphorus, calcium, magnesium and sulphur had the lowest accumulated concentrations; however, these values are also indicative of nutrient cycling, since smaller amounts of these nutrients are required by successor crops.
The data on fertilizer equivalents show the importance of forages to the nutritional balance of plants in integrated production systems. The values of fertilizer equivalents (N, P2O5 and K2O) in the forage plants were higher than those found in maize biomass due to the higher biomass production and nutrient release from plant residues, which is interconnected with the use of animal excreta that constitute a nutrient return pathway in the soil, improving the dynamics of mineralization and releasing nutrients more efficiently to the system (Dias et al., Reference Dias, Costa, Severiano, Bilego, Furtini Neto, Almeida, Brand and Lourival2020).
Tanaka et al. (Reference Tanaka, Crusciol, Soratto, Momesso, Costa, Franzluebbers, Junior and Calonego2019) concluded that forages (especially B. brizantha), when used as plant mulch, promote greater nutrient cycling and allow farmers to produce sustainably, corroborating the results of the present study. Assmann et al. (Reference Assmann, Martins, Anghinoni, Denardin, Nichel, Costa, Silva, Balerini, Carvalho and Franzluebbers2017) emphasize the importance of considering the nutrients from biomass in integrated systems in the calculation of fertilizer recommendations because a large proportion of these nutrients return to the soil. This higher amount of equivalent nutrients from the forages led to higher yield in successor crops and decreased the cost of purchasing chemical fertilizers. Thus, when considering the high cost of fertilizers, it is possible that the continuous use of cover crops in agricultural soybean production systems allows the most efficient use of nutrients available in the soil at lower production costs and maintains greater sustainability in production.
The mean height of the soybean plants was 64.5 cm, which is in agreement with the results of Machado et al. (Reference Machado, Cecato, Comunello, Concenço and Ceccon2017). The mean plant height and first pod insertion height are traits that vary between soybean genotypes and show some plasticity in relation to environmental changes (Franchini et al., Reference Franchini, Balbinot Junior, Debiasi and Procópio2014). The effects of vegetation cover on the agronomic characteristics of soybean have also been previously evaluated. For example, Dias et al. (Reference Dias, Costa, Severiano, Bilego, Furtini Neto, Almeida, Brand and Lourival2020) evaluated the sowing of Tamani guinea grass in succession of soybean and obtained a mean soybean plant height of 67.3 cm, which is similar to that observed in this study.
The mean first pod insertion height of the cropping systems was 14.99 cm, which is similar to those obtained in the studies of Franchini et al. (Reference Franchini, Balbinot Junior, Debiasi and Procópio2014) and Crusciol et al. (Reference Crusciol, Nascente, Mateus, Pariz, Martins and Borghi2014). The pod insertion height is directly related to grain yield (Carkner and Entz, Reference Carkner and Entz2017). A first pod height of 15 cm allows for efficient harvesting because lower values result in losses in harvesting due to the cutting height of the harvester platform (Rebilas et al., Reference Rebilas, Klimek-Kopyra, Bacior and Zajac2020).
The higher number of grains/pod in Paiaguas palisadegrass may be associated with its higher amount of biomass, which contributed to greater nutrient cycling. Machado et al. (Reference Machado, Cecato, Comunello, Concenço and Ceccon2017) reported that the fasciculated root system of tropical forages allows plants to explore a larger volume of soil and extract more water than a plant with pivoting roots, such as soybean. Tropical forages contribute to the stability of the particles in the aggregation, structuring and permeability of the soil, which may favour the development of the root system to deeper layers and allow greater exploitation of its profile and greater of soil, providing greater absorption of water and nutrients and improving the aeration of the layers explored by the roots, leading to a positive impact on crop production (Flávio Neto et al., Reference Flávio Neto, Severiano, Costa, Guimarães Junnyor, Gonçalves and Andrade2015).
The higher values observed for 1000-grain weight and grain yield obtained in forage mulch are related to the higher forage biomass production and nutrient accumulation and release and indicate the efficiency of adopting the integrated system for the production of biomass suitable for the no-till system in successor crops.
The adequate management of animals in an integrated crop–livestock system is important to maximize the benefits of nutrient cycling because adequate grazing increases the soil organic matter content (Assmann et al., Reference Assmann, Anghinoni, Martins, Costa, Kunrath, Bayer and Carvalho2014) via nutrient return through the excretion of manure and urine, and the animals act as nutrient recycling agents (Carvalho et al., Reference Carvalho, Anghinoni, Moraes, Souza, Sulc, Lang, Flores, Lopes, Silva, Conte, Wesp, Levien, Fontaneli and Bayer2010), benefiting the successor crop.
Higher soybean yield in integrated systems compared to maize in succession was also observed in the study by Dias et al. (Reference Dias, Costa, Severiano, Bilego, Furtini Neto, Almeida, Brand and Lourival2020), showing the importance of using the excreta of animals as a nutrient return pathway, the protection of the soil by forage plants and better nutrient cycling for the soybean crop.
Carvalho et al. (Reference Carvalho, Barro, Barth Neto, Nunes, Moraes, Anghinoni, Bredemeier, Bayer, Martins, Kunrath, Santos, Carmona, Barros, Carvalho, Peterson, Nunes, MartinS, Souza Filho, Bertolazi, Kunrath, Moraes and Anghinoni2018) highlighted that areas without grazing, where vegetation serves the sole purpose of producing biomass for the no-till system in succession, do not produce more than grazed areas and that the introduction of the animal component into the system brings several benefits for nutrient recycling. Thus, the main advantage of using the integration system in the off-season compared to the maize crop comes from the forages and animals.
Growing forages in succession to soybean is an option for diversifying activities (Fig. 2) and maximizing land use throughout the year. The soybean has contributed to the benefit of biological nitrogen fixation by a rhizobial bacterial strain (Bradyrhizobium japonicum) of soybean; this strain produces nodules in the soybean roots that can promote an appropriate environment for the conversion of atmospheric nitrogen into ammonium, which is the nitrogen form available for plant use, whereas the plant provides carbohydrates as an energy source for these bacteria (Ntambo et al., Reference Ntambo, Chilinda, Taruvinga, Hafeez, Anwar, Sharif, Chambi and Kies2017). This exchange contributes to improving soil fertility for the forages used in the system in succession. Integrated crop–livestock systems combined with no-till systems contribute to improving farm revenue (Crusciol et al., Reference Crusciol, Nascente, Mateus, Pariz, Martins and Borghi2014) and to greater sustainability.
Conclusions
The use of the integrated crop–livestock system in the form of forage succession provided greater soil cover and nutrient cycling as a result of the better utilization of the animal's excreta, than the cropping of maize in succession and resulted in higher soybean productivity, thus contributing to agricultural sustainability. Paiaguas palisadegrass and Tamani guinea grass showed a C:N ratio greater than 30:1, indicating slow decomposition of plant residues. The forages accumulated the amounts of nutrients in their biomass that met the soybean demand, resulting in higher grain yield.
Financial support
This work was supported by the Goiano Federal Institute (Instituto Federal Goiano).
Conflict of interest
None.
Ethical standards
Not applicable.