Introduction
Terrestrial analogues for off-Earth environments provide a natural laboratory for conducting experiments and testing instruments and operational practices for future space exploration missions. In addition to well-known analogue regions (Hipkin et al., Reference Hipkin, Voytek, Meyer, Leveille and Domagal-Goldman2013), efforts are being made to recognize unexplored and less studied parts of the world and introduce relevant sites to the astrobiology community (Preston and Dartnell, Reference Preston and Dartnell2014). The objective of this paper is to introduce Ladakh, India (Fig. 1), as a region of astrobiological significance by providing an overview of sites visited in August 2016. Ladakh is a cold, very high-altitude (~3000–5700 m above sea level; Fig. 2) Himalayan desert region located in the eastern part of the state of Jammu and Kashmir of Northern India. It offers a natural setting where a diverse range of mostly near-pristine extreme environments including glacial deposits, arid regions, dune fields and intra dune ponds, hot springs, saline lakes and permafrost regions can be found and accessed by road (1–2 days by car) from its capital city of Leh. Leh is directly accessible using commercial air carriers from Indira Gandhi International airport in Delhi. As a result of altitude, the region has lower atmospheric oxygen partial pressures, higher levels of UV irradiation and receives little to no rainfall. Such a rare setting is desirable for multidisciplinary astrobiology teams studying paleoenvironments, geobiology and those developing and testing life detection techniques and instrumentation for other worlds. Our expedition was principally a scouting expedition to the region (covered sites listed in Table 1). As a result, we focus on describing geological settings and gathering environmental data of the sites, while also investigating their level of accessibility and pristineness.

Fig. 1. Map of Ladakh, India visited sites circled in red (Image Courtesy: www.ladakhtours.com/ladakhmap.html).

Fig. 2. Digital elevation model of the area (modified from Phartiyal et al., Reference Phartiyal, Singh, Nag and Singh2014).
Table 1. Location type, elevation and coordinates of the expedition sites

Astrobiologically relevant sites
The visited sites are introduced section-wise with a brief overview of the previous investigations in the region, the geological setting and our observations describing the site's potential to support astrobiology research. A summary of the investigations conducted at some of the site types (glacial high passes, sand dunes and hot springs) is given. The relevant activities carried out per site (landform mapping, comparative planetology, instrument testing, life detection protocol study, biomass distribution, metabolism study) is presented in a table (Table 2) to bring out the ‘astrobiology relevance’ of the sites.
Table 2. To highlight the ‘astrobiological relevance’ of the region, a list of activities is presented against the sites covered in the 2016 pathfinding expedition

Glacial high passes
The high passes of Khardung La and Taglang La crest at altitudes of 5359 and 5328 m, respectively. Although the two passes have similar altitude, their geomorphology and geology are very different. These high passes are unique in their high altitude, with rocks exposed to higher UV-A doses (~10× greater than sea level; Dvorkina and Steinberger, Reference Dvorkina and Steinberger1999), melt water pools at glacier terminuses and the presence of permafrost. These high passes present several analogue features for early Mars, given their combination of low atmospheric pressure, high UV (greater UV-A doses than experienced on Mars in the present day; Cockell et al., Reference Cockell, Catling, Davis, Snook, Kepner, Lee and McKay2000), permafrost, active periglacial activity, glaciers and glacier melt. The atmospheric density at 5300 m is approximately 0.64 kg m−3, compared with 1.2 kg m−3 at sea level. Hesperian Mars is estimated to have had an atmospheric density of at least 0.4 kg m−3 (Manga et al., Reference Manga, Patel, Dufek and Kite2012). Noachian pressures may have been much higher (Cockell et al., Reference Cockell, Catling, Davis, Snook, Kepner, Lee and McKay2000). Preservation of biomarkers is another avenue of research in these high passes. Indeed, taphonomic pathways involved in the preservation of organic matter in these environments could help inform the preservation potential of biomarkers in soils, sediments and weathering profiles on early Mars. Such information is relevant for future Mars exploration instrumentation.
Site description
Khardung La Pass
Khardung La has been strongly eroded by glacial activity to the north and south, with steep U-shaped glacial valleys eroded into the granites of the Ladakh batholiths (late Cretaceous–Palaeocene epoch, Weinberg et al., Reference Weinberg, Dunlap and Whitehouse2000). The glacier on the south side is the Khardung glacier, while the smaller glacier and glacial remnants on the north and west sides (Fig. 3(a)) are unnamed. The pass itself is formed by a col, with the ridge east and west steepened by erosion into arêtes. The slopes are mantled by large blocks, forming a thick mantle talus. Down slope movement of the blocks is a frequent occurrence, lubricated by snow melt. The informally-named north Khardung glacier has a prominent recent moraine. Relatively flat surfaces in the valley floor to the north of the pass show development of polygonal ground and stone stripes, interspersed with the oriented boulders of block streams (Fig. 3(b) and (c)). One moraine (34°17.321′N, 77°35.714′E; altitude: 5098 m) possesses patterned ground, a typical characteristic feature of periglacial regions. This pattern is developed due to alternate freezing and thawing of the soil. The sides of the valley towards the locality known as North Pulu show well-developed talus cones of probable periglacial origin, while the valley floor, currently occupied by the headwaters of the Khardung Rong stream, shows a succession of terminal moraines. Age of deglaciation is unclear, however, the lake sediments in the valleys date post the Last Glacial Maximum (LGM) and the pro-glacial lake sediments date around Mid Holocene (Phartiyal et al., Reference Phartiyal, Sharma and Kothyari2013; Nag and Phartiyal, Reference Nag and Phartiyal2015; Phartiyal et al., Reference Phartiyal, Singh and Kothyari2016). Features of potential astrobiological interest of Khardung La would include the rock surfaces exposed to a high UV flux and melt water pools at the terminus of the north Khardung glacier. Permafrost is likely to occur at a shallow depth throughout Khardung La (with layers at least 40 cm deep) by analogy with Chang La pass (5360 m, Pant et al., Reference Pant, Phadtare, Chamyal and Juyal2005). However, the very coarse nature of the surface mantle and the shallow bedrock would make it difficult to access.

Fig. 3. (a) Snout of the small glacier, west of Khardung La. (b) Sorted margin of the stone polygon in the alpine meadow to north of Khardung La. (c) Coring soils in the alpine meadow, north of Khardung La. (Photo credits: Dr Jonathan Clarke).
Taglang La
The bedrock at Taglang La is composed of metamorphosed sediments. The landscape is less steep than Khardung La, though still composed of the U-shaped valleys characteristic of glacial erosion (Fig. 4(a)). The smooth slopes suggest that the entire area was overridden by glacial ice in the past. Higher altitude glacial remnants are preserved at elevations of 5600 m, 2.5 km to the southwest of the pass. The pass occurs near the contact between two different rock types, carbonaceous phyllites and fine-grained quartzites to the west and coarse-grained white marbles to the east (Fig. 4(b)). The contact between the two lithologies runs almost north-south through the pass. The phyllites and quartzites are exposed only in roadside cuttings whereas the marbles form prominent knolls and steep-sided peaks. Fuchs and Linner (Reference Fuchs and Linner1995) date these sediments as Palaeozoic, possibly Permian. The regolith mantle over the phyllites is largely continuous, consisting of poorly sorted silt-to-pebble sized fragments and occasional large boulders of quartz, which are possible glacial erratics. The regolith is both riled and terraced, probably by runoff and solifluction, respectively. The regolith mantle over the marbles is discontinuous, with extensive areas of bare rock. Where present it consists of coarse sand to gravel-sized carbonate fragments.

Fig. 4. (a)View from Taglang La looking south. (b) North-facing slope at Taglang La showing the contrast between dark-toned quartz-rich regolith on carbonaceous sediment in the foreground and barren, light-toned carbonaceous (limestone) outcrops in the background. Moss colonized terracettes and solifluction lobes visible in the foreground (Photo credits: Dr Jonathan Clarke).
Features of potential astrobiological interest at Taglang La would include the exposed rock surfaces, with the added contrast between the metamorphic fine-grained sediments and carbonates. Permafrost is also likely to occur at a shallow depth throughout Taglang La by analogy with Chang La (Pant et al., Reference Pant, Phadtare, Chamyal and Juyal2005), and the very finer-grained nature of surface mantle may make access via surface excavation more practical than at Khardung La. West-facing shadowed areas in road cuts below the pass are marked by well-developed icicles. Permafrost is likely very close to the surface.
Development of regolith-landform maps for glacial high passes
Regolith-landform mapping is an approach to describing the Earth's surface in terms of both landforms and the surface materials. These could include transported material, weathered rocks, duricrust, or fresh bedrock (Pain et al., Reference Pain, Chan, Craig, Gibson, Kilgour and Wilford2007; Pain, Reference Pain2008). Techniques of regolith-landform mapping have proved an important part of any field study on Earth involving surface materials, landscape, biota and hydrology. The methodology can be extended to Mars analogue locations, and to the interpretation of Mars surface features (Clarke and Pain, Reference Clarke, Pain and Cockell2004). Regolith-landform mapping methodologies have not previously been applied to regolith and landforms of terrestrial cold climates. Ladakh provided an opportunity to develop such a methodology. An example of regolith-landform mapping in a cold climate is shown in Fig. 5. Figure 5(a) shows an interpreted ground-level image of a steep, glacier-headed valley not far from Khardung La, and Fig. 5(b) is an interpreted Google Earth image of the same area. The units are consistent with each other. Each unit consists of regolith defined by a single landform and is a homogeneous composition at the scale of mapping. Regolith-landform units are characterized by three properties: a landform descriptor in lower case letters forming a prefix, a regolith material descriptor in upper case letters with lower case letters in brackets to describe interstitial ices, salts and carbonates (if present) and a numerical modifier for periglacial modification (if present) as a suffix. Each descriptor is unique. The units in Fig. 5 are described in Table 3. Results from the regolith mapping exercise are still being integrated with data from elsewhere. However, the results from the ground mapping at Khardung La closely match those made from aerial photographs and show the utility of the technique in the characterization of landforms and regolith materials in periglacial environments. One limiting factor identified in the methodology was in the use of aerial or satellite images in that the regolith materials could not be accurately predicted where there was a significant change in texture just below the resolution of the imagery. A prime example of this is the south-facing rock glaciers in the valley between Tso-Kar and Puga, the surface of these appears relatively smooth in Google Earth images, apart from furrows and ridges and occasional large boulders. Those examined on the ground, however, were largely covered by boulders that were smaller than the image resolution (0.7 m).

Fig. 5. (a) Site-scale regolith-landform units mapped onto a ground-level photograph from Phyang glacier terminus, Ladakh. (b) Regolith-landform map of the Phyang glacier in Ladakh on Google Earth base image. Location 34°17′33.66″N, 77°33′12.69″E (Image credits: Dr Jonathan Clarke).
Deliquescent melting of calcium chloride at Khardung La
One of the goals of the campaign was to validate, in a representative environment, the operation of the HabitAbility: Brines, Irradiance and Temperature (HABIT) instrument. HABIT is part of the ExoMars Surface Platform of the European Space Agency (ESA) and the Russian Federal Space Agency (Roscosmos). HABIT is a cutting-edge, multipurpose instrument composed of: (i) ENVPACK, a surface environmental package devoted to evaluating the habitability of the landing site; and (ii) BOTTLE (Brine Observation Transition To Liquid Experiment), an In-situ Resource Utilization instrument for future Mars exploration. The goal of the field-site validation was to demonstrate the basic functional principle of HABIT for liquid brine production, namely the water absorption from the atmosphere on salt support. Other goals were to validate the time response of the salts to ambient conditions, the efficiency of air flow through the HABIT-lid and the role of the lid in shadowing and protecting the salts, and the phase change of non-conducting salts to conducting hydrates and then to liquid brine. Figure 6 shows an open view of the HABIT field-site prototype. Both parts were covered by lids, except for vessel 6 of the BOTTLE container unit. Vessels 1 and 2 were filled with sodium perchlorate, vessels 3 and 4 were filled with calcium perchlorate and vessels 5 and 6 were filled with calcium chloride salts. Models can predict what the state of the salt is in, assuming equilibrium with the atmosphere and under a given set of ambient temperature (T) and relative humidity (RH). The phase diagram of each tested salt can be used to interpret the change of conductivities, depending on the measured RH and T values, at each one of the measured sites. The ambient conditions at different sampled sites are shown in Fig. 7 against the phase diagram of calcium chloride. Figure 8 shows an example of the HABIT measurements at Khardung La, here shown only for calcium chloride. The conductivity of a salt solution requires AC (alternating current) excitation, to avoid the polarization of the solution and the corrosion of the electrodes. Additionally, the AC current needs to operate at high frequencies since a salt solution behaves like a resistance + capacitor circuit. For this specific case, the prototype of HABIT operates, with a square wave of 5 V amplitude, at 10 kHz. Previous measurements of electrical conductivity on Mars by the Thermal and Electrical Conductivity Probe (TECP) of the Phoenix lander were implemented similarly, with a square wave running at 1 kHz and an input voltage of 2.5. These environmental measurements informed that at Khardung La, environmental conditions were such that calcium chloride should hydrate and then melt into the liquid phase by deliquescence.

Fig. 6. Open view of the HABIT prototype for the field-site campaign, including the six vessels of the BOTTLE container unit (in white), with the conductivity pins at three different heights and the electronic unit (in green) (Photo credit: HABIT team/LTU).

Fig. 7. Phase diagram of calcium chloride and measured ambient conditions in Ladakh compared to measurements taken in Iceland. The ambient conditions at Khardung La are clearly compatible with a transition from crystalline hydrated phase to liquid brine phase.

Fig. 8. Example of the observations of HABIT prototype at Khardung-La: change of electrical conductivity over time (shown in volts) for the CaCl2 containers and the corresponding ambient RH and T. Here example of the signal observed in the calcium chloride samples of a covered-compartment (left) and an open-air compartment (right) and simultaneous ambient temperature and RH.
Sand dunes
Aeolian features are common in Ladakh, especially along valley floors and the lee side of lakes where there are locally abundant supplies of sand-sized materials. Landforms include dunes (Pant et al., Reference Pant, Phadtare, Chamyal and Juyal2005) and sand ramps (Kumar et al., Reference Kumar, Bora and Sharma2016), and sand sheets associated with lakes such as Kyagar Tso (Section ‘Kyagar Tso’). The expedition team carried out several investigations at the Hunder dunes where the dunes provide useful analogues for small Martian valley dunes and the ephemeral pools in the swales and their deposits may be of astrobiological interest as similar pools could have conceivably existed on early Mars during the Noachian or even later during Martian history (e.g. Howard et al., Reference Howard, Moore and Irwin2005). Ancient inter-dune pools on Earth have been known to preserve fossils such as stromatolites (Eisenberg Reference Eisenberg2003). Similar deposits should be recognizable in ancient dune deposits on Mars and may preserve signs of ancient life. These features enhance the potential of this site as a test bed for astrobiology-relevant investigations.
Hunder dunes: site description and observations
The dunes at Hunder, with their short-lived pools and fluvial sediments in the inter-dune spacings (Fig. 9(a) and (d)), are easily accessible for exploration and sampling. These, along with other dunes in the Shyok and Nubra valleys (Fig. 2), are largely unstudied. Exposed rocks are categorized under the Shyok Group (Thakur, Reference Thakur1981), Tsoltak and Shyok Formation (Thanh et al., Reference Thanh, Itaya, Ahmad, Kojima, Ohtani and Ehiro2010), and Shyok Formation (Weinberg et al., Reference Weinberg, Dunlap and Whitehouse2000; Kumar et al., Reference Kumar, Bora and Sharma2016). Dortch et al., Reference Dortch, Owen and Caffee2010 describe glacially derived landforms, e.g., moraines and roche moutonnées around the area, formed during the Deskit 1 and Deskit 2 glacial stages. The rapidly declining elevation of these landforms near the Hunder village is considered an indicator for the termination of glacial ice. Pant et al. (Reference Pant, Phadtare, Chamyal and Juyal2005) reported that the dune fields are the only Quaternary deposits that can be observed downstream of the River Shyok. All these dunes are probable results of climatic events during Holocene time. However, Phartiyal and Sharma (Reference Phartiyal and Sharma2009) describe the whole area as having once existed under a massive lake system which extended for ~40–50 km upstream and into the Nubra valley during late Quaternary. The Hunder dune field lines the south-western side of the Shyok valley between Diskit and Hunder. Each of these towns is situated atop a large alluvial fan, which acts to shelter the dune field from intense winds blowing up or down the valley. The dune field consists of three distinct areas, separated by a freshwater creek and an anabranch of the River Shyok. Rippled sedimentary deposits indicate that water has flowed over the dune field site at some time. However, the timeframe of these events is unclear. Construction of rose diagrams using QGIS software indicated a seasonal trend in dune orientation and wind direction, unusual for barchan, transverse or barchanoid dunes but can be explained by the mild weather variation in the region, with the wind direction changing within 60° (McGuirk et al., Reference McGuirk, Clarke, Strong and Mills2018).

Fig. 9. (a) Wind eroded inter-dune sediments in Hunder (Photo Credit: Dr Jonathan Clarke). (b) Inter-dune Pond, Hunder (Photo Credit: Dr Jonathan Clarke). (c) For comparison, a dune field inside Endurance crater on Mars (Photo credit: Opportunity rover NASA/JPL Caltech). (d) Stack inverted dune swale deposits (Photo Credit: Dr Jonathan Clarke).
Transverse-barchanoid dunes at Hunder indicate a consistent north-westerly wind direction. Many dunes in the westerly area of the dune field have a steep slip face with angles up to 35° recorded (equivalent to the angle of repose of loose sand). A variety of inter-dune surfaces including rocky ridges, layered sand swales and mud-cracked clays are present. These are shifting dunes due to wind funnel activity in this region. Recently, they have become very sparse but many are found on the left bank of the wide confluence (~10 km) of the Shyok and the Nubra rivers. Inter-dune ponds of small-to-moderate size mark hollows between some dunes. One of the ponds most closely examined was 20 cm deep at the deepest point and situated 2–3 m above the level of the creek. Some swale deposits have been inverted by aeolian erosion, exposing stacked cycles of fluvial and pond sediments capped by mud-cracked horizons. The age of these sediments is not known, but they are presumed to be from the Holocene. Hunder's ephemeral ponds (pH 6.0–6.5) (Fig. 9(b)) are an intriguing Mars analogue site for the study of clay formation in a relatively dry, and relatively small dune field environment, accessible on foot. The ponds can be different with respect to vegetation distribution, depth and relationship with the surrounding clay beds. Some of the ponds contain clear water with more suspended particles, due to organic enrichment from plant debris and local fauna visitation when water becomes available. No recent river sand or flood deposits are apparent around the ponds, indicating the pond water is likely sustained by a high groundwater table, and/or recharging by rainstorms. It is likely that the ponds are recharged by rainwater seeping through the sand and collected over non-permeable beds of clay sediments.
The Hunder dune field could represent a suitable analogue model for understanding a desiccating early Mars (Fig. 9(c)), perhaps punctuated by short-lived liquid water, still supporting ephemeral life. Hunder's dry climate is ideal to assess how absorption and preservation of organic matter in clays could be related to the putative preservation potential of organics in Martian clay beds (e.g., Hamilton et al., Reference Hamilton, Morris, Gruener and Mertzman2008). On Earth as well as on Mars, the reactivity between regolith's minerals and organics affects preservation and detection of organic matter and thus the direct evidence of life (e.g., Klein et al., Reference Klein, Horowitz, Levin, Oyama, Lederberg, Rich, Hubbard, Hobby, Straat, Berdahl, Carle, Brown and Johnson1976; Navarro-González et al., Reference Navarro-González, Vargas, de la Rosa, Raga and McKay2010). Organics are poorly preserved in oxidising environments (Davila et al., Reference Davila, Gross, Marzo, Fairén, Kneissl, McKay and Dohm2011), but can be preserved in clay-rich deposits against oxidation (e.g., Hedges and Keil, Reference Hedges and Keil1995). Dissolved organics adsorbed on mineral surfaces can be preserved against microbial decay (e.g., Aggarwal et al., Reference Aggarwal, Li and Brian2006; Yu et al., Reference Yu, Dong, Jiang, Guo, Eberl, Li and Kim2009). Therefore, Hunder, akin to Martian dune fields and inter-dune clay deposits, could represent an intriguing target to seeking mineral, sedimentary and preserved molecular evidence of life.
Hot springs
Hot springs serve as valuable off-Earth analogue sites due to the presence of extreme conditions for the survival of life. These include high temperatures, extremes of pH, the paucity of dissolved oxygen and limited nutrients. Although not conducive for most of the life forms, hot springs host diverse microbial communities. Studies of these extremophilic microorganisms help understand adaptations of life to extreme environments that may have relevance to the early Earth and Mars. The hot springs in Ladakh are potentially unique study sites for astrobiological research because of their high elevation. The regional geology comprises three tectonic belts. The Northern Belt consists of the Indus Group sedimentary sequence and the Ladakh granites, and hosts the Chumathang geothermal system (Craig et al., Reference Craig, Absar, Bhat, Cadel, Hafiz, Hakhoo, Kashkari, Moore, Ricchiuto, Thurow and Thusu2013). The Central Belt consists of the Indus Suture Zone (ISZ), an area subjected to intense tectonic activity and uplift. The Southern Belt, containing the Puga Formation, is a succession of sedimentary and metasedimentary units consisting of Precambrian paragneiss, schist and phyllites, with interbedded layers of limestone and sections of locally intrusive Tertiary granites (Singh et al., Reference Singh, Tiwari and Singh2005). Because Ladakh lies in the rain shadow region of the Great Himalayas, the geochemistry of these hot springs is minimally influenced by atmospheric precipitation. These springs consist of moderately hot to very hot water (60–75°C; water boils at temperature 90°C at 3000 m altitude and 86°C at 4500 m altitude) of moderately alkaline pH (<9). The presence of calcium sulphates at some Ladakh springs suggests an analogy to primitive Martian environment where gypsum has been detected (Squyres et al., Reference Squyres2004) and may help unravel putative Martian geomicrobiological processes (Shukla et al., Reference Shukla, Ray and Bhattacharya2017).
Site description
Panamik springs
The Panamik area (Fig. 10) exposures rocks of the Karakoram Plutonic Complex (Thakur, Reference Thakur1981) including granites, granodiorites and leucogranites. In these rocks, various minerals such as plagioclase, quartz, biotite, amphiboles, along with accessory magnetite, chalcopyrite, galena and zircon are reported (Thanh et al., Reference Thanh, Itaya, Ahmad, Kojima, Ohtani and Ehiro2010). The Karakoram Plutonic Complex is described as a sequence of Eocene–Miocene age (Thakur, Reference Thakur1981). K-Ar biotite methods date the plutons at Panamik to 95.7 ± 2.1 and 96.7 ± 2.1 Ma before present (Thanh et al., Reference Thanh, Itaya, Ahmad, Kojima, Ohtani and Ehiro2010).

Fig. 10. (a) Panamik Hot Spring site. (b) Differing colouration of the biomass at hot spring source and along the flow (Photo Credits: Mr Rakesh Rao and Dr Mukund Sharma).
The hot springs at Panamik are located on a steep upward incline behind public baths and are easily accessible from the road. The active area measures ~250 m × 100 m, with several sites of upwelling (mainly on the lower third). Upwelling sites are small and of low discharge. The hill was covered with grass with almost no vegetation immediately around the hot springs. Water was analysed from five sites. Site PU1 hosted green coloured biofilm, while site PU2 hosted orange-yellow biofilm with temperatures of around 62°C and a pH of 8.5. Sites PU3, 4 and 5 were hotter at around 74°C, with a pH of around 7.7 with minimal or no visible biofilm. Outflow of water was continuous and a slight smell of sulphide was present.
Chumathang springs
The Chumathang Hot Spring site (Fig. 11) (Craig et al., Reference Craig, Absar, Bhat, Cadel, Hafiz, Hakhoo, Kashkari, Moore, Ricchiuto, Thurow and Thusu2013) is located along the Indus River behind an active complex of buildings. Some discharge sites closer to the complex are contaminated with outwash and seepage from the complex. The least impacted site was a cyclically geysing spring erupting every 8–9 min located by the concrete rectangular water tower (33°21′36.10″N, 78°19′26.79″E). This site seemed the most pristine (T = 72–8°C, pH = 8.3–8.8). Another site boiling more vigorously is present by the concrete wall on the river's edge, but plastic detritus littered it. The outcrops and grounds around the springs are colonized by endolithic and chiasmolithic microorganisms penetrating within the crystal grains. The Indus river completely submerges the springs during high discharge events.

Fig. 11. (a) Chumathang Hot Spring site. (b) Collecting hot spring water samples using a peristaltic pump (Photo Credits: Dr Sanjoy Som).
Puga springs
The Puga Hot Springs (Fig. 12) are a boron-rich geothermal system (Ghosh et al., Reference Ghosh, Mallick, Haldar, Pal, Maikap and Gupta2012) located at the mouth of the Puga Valley in the north-western part of the Himalayas (Saxena and D'Amore, Reference Saxena and D'Amore1984). Boron has been proposed as a key element in the prebiotic formation of RNA, a molecule suggested as the hypothetical link between prebiotic chemistry and modern biochemistry (Orgel, Reference Orgel2004). Boron has been found associated with one of the oldest evidence of life, the 3.48 Ga Dresser formation in Australia (Djokic, Reference Djokic2015).

Fig. 12. (a) Puga Hot Spring crusts sample site. (b) Soluble puffy white crust in Puga Valley. (c) Differing coloration in biofilm mats at sampling sites. (d) White crust deposits in Puga Valley. (e) Algal bioherm collected from Puga Hot Springs (Photo Credits: UNSW ACA and BSIP).
Like most major continental borate deposits (Grew et al., Reference Grew, Bada and Hazen2011), the Puga system lies within a down-faulted block in an active collisional zone, with the nearby ISZ marking the boundary between the Indian and Asian continents (Craig et al., Reference Craig, Absar, Bhat, Cadel, Hafiz, Hakhoo, Kashkari, Moore, Ricchiuto, Thurow and Thusu2013). Tourmaline has not been found in any hot spring deposits at Puga, and boron-bearing minerals in the system are predominantly kernite, tincalconite and borax (Ghosh et al., Reference Ghosh, Mallick, Haldar, Pal, Maikap and Gupta2012). The hot springs occupy an area of 4 km × 1 km and exhibit a range of geothermal activity, including geysers; mud pools; and sulphur and boratic mineral deposits.
The Puga geothermal system is fault bounded (Craig et al., Reference Craig, Absar, Bhat, Cadel, Hafiz, Hakhoo, Kashkari, Moore, Ricchiuto, Thurow and Thusu2013), which restricts the geothermal activity to within the NNE–SS–trending Kyagar Tso Fault to the west and the NW–SE–trending Zildat Fault to the east, the latter representing part of the ISZ. Native sulphur and sulphate deposits along the north side of the valley suggest buried faults marking the northern boundary (Shankar et al., Reference Shankar, Padhi, Prakash, Thussu and Wangdus1976). The Puga valley is predominantly filled with alluvial cover, consisting of aeolian sand, fluvial sediments and glacial deposits encrusted with sulphur, borax and other geothermal minerals. This cover extends to depths up to 65 m, where it forms lithified breccia (Fig. 12(d)) due to mineral precipitation from the circulation of hydrothermal fluids (Singh et al., Reference Singh, Tiwari and Singh2010). Late stage magmatic activity has been suggested as the heat source for the Puga geothermal system (Chowdhury et al., Reference Chowdhury, Handa and Das1974; Saxena and D'Amore, Reference Saxena and D'Amore1984), due to high enrichments of Li, Rb and Cs in hydrothermal fluids, and results from magnetotelluric studies (Harinarayana et al., Reference Harinarayana, Azeez, Naganjaneyulu, Manoj, Veeraswamy, Murthy and Rao2004; Azeez and Harinarayana, Reference Azeez and Harinarayana2007). Despite the main road going along the Puga river valley, accessing many of the Puga spring sites is challenging because of the swampy (and potentially hazardous) nature of the area. We identified a site (4414 m) easily accessible from the road. The site (33°13′39.80″N, 78°20′50.80″E; Fig. 13) is an abandoned borehole. The fluid discharge site (15 cm diameter at the centre of a ~1.5 m diameter pool, T = 70°C) is open and amenable to sampling and flows into four different pools of three distinct temperature (70, 40, 20°C). The pH is neutral. Hot water runoff from the springs showed the presence of coloured (pink/yellowish orange/green/grey) microbial mats (Fig. 12(e)). The release of gas bubbles was observed when the underlying sediment was disturbed, possibly from hydrogen sulphide, since there was a high abundance of sulphur cyclers observed in the area. The smell of hydrogen sulphide gas, typically released by sulphate-reducing bacteria, was also observed close to the hot springs.
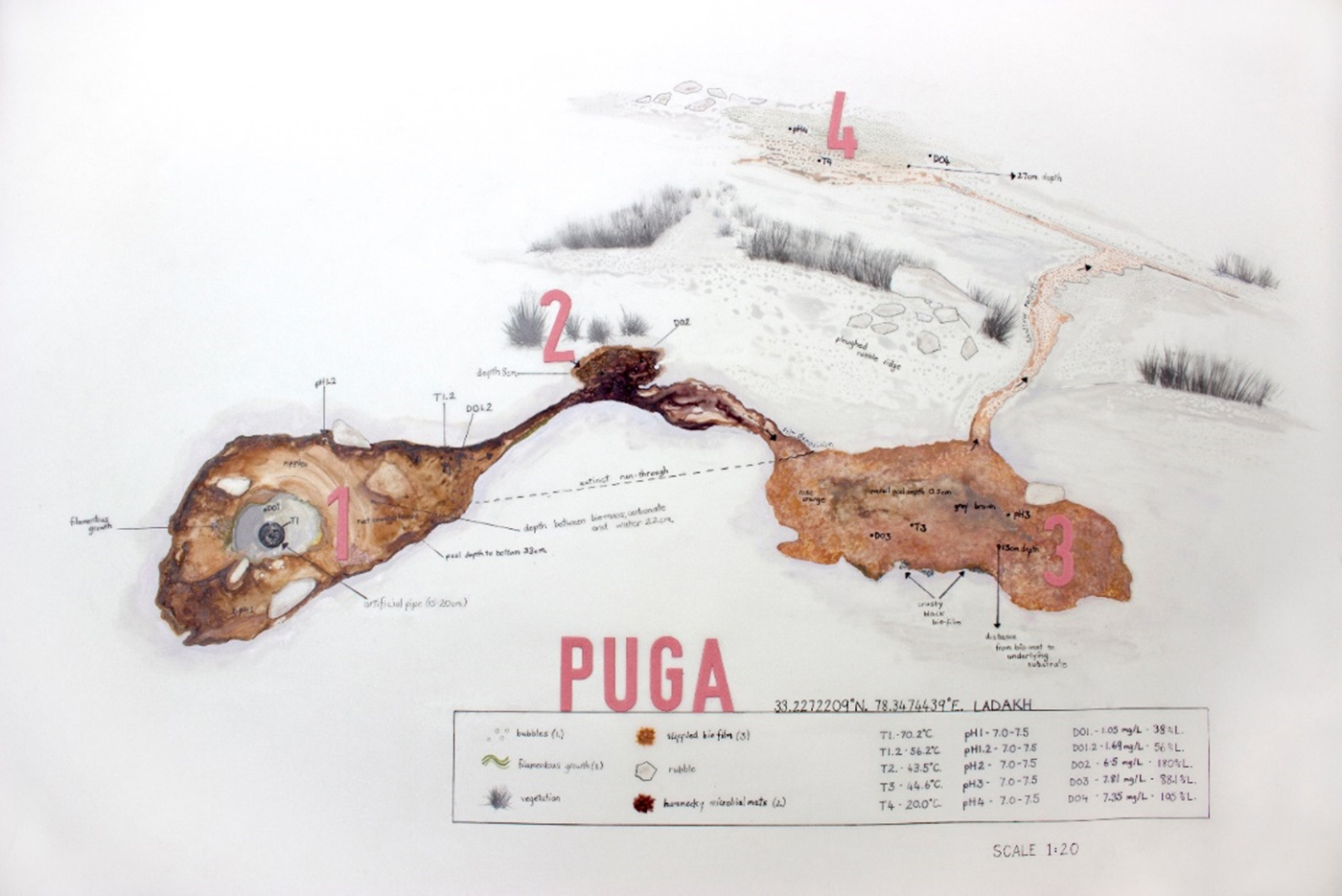
Fig. 13. Abandoned borehole discharging into four distinct pools at Puga (Art Credit: Ms Annalea Beattie).
Investigations and outcomes from the hot spring sites
Formation and stability of prebiotically relevant systems in hot springs
Current thinking about the emergence of early life assumes the emergence of primitive cells, which are comprised of two main components: an encapsulated genetic material surrounded by an encapsulating membrane boundary (Szostak et al., Reference Szostak, Bartel and Luisi2001). The primitive encapsulating membranes are thought to have been composed of simple amphiphilic molecules like fatty acids (Deamer, Reference Deamer2017). Different fatty acids have been shown to form vesicles at specific conditions. However, the stability of such vesicles at extreme conditions of pH, temperature and variable ionic concentration is not known. We explored the stability of vesicles in Ladakh spring waters. Water samples were collected at the three different hot springs in Ladakh, namely Panamik, Chumathang and Puga. Combinations of prebiotically pertinent fatty acids and their derivatives were evaluated for the formation of vesicles and reported in Joshi et al. (Reference Joshi, Samanta, Tripathy and Rajamani2017). The stability of these vesicles was characterized by multiple dehydration–rehydration cycles, at elevated temperatures. Mixtures of fatty acid and glycerol derivatives were found to be the most robust, also resulting in vesicles in all the hot spring waters that were tested. Importantly, these vesicles were stable at high temperatures, and this fatty acid system retained its vesicle-forming propensity, even after multiple cycles of dehydration–rehydration. The remaining systems, however, formed vesicles only in bicine buffer. The results suggested that certain prebiotic compartments would have had a selective advantage in terrestrial geothermal niches.
Carbon metabolism in hot spring thermophiles
Hot springs having temperatures >~70°C are non-conducive to photosynthesis. They are commonly inhabited by prolific biofilms of chemoheterotrophs, where researchers have had a limited understanding of how these communities derive carbon and energy, which is a requirement to maintain high levels of biomass (Schubotz et al., Reference Schubotz, Hays, D'Arcy, Gillespie, Shock and Summons2015). The objective of our study was to understand the carbon metabolism in thermophiles, especially those living above 70°C. Through a 13C-labelled glucose incubation experiment with thermophiles at the two thermally distinct hot springs (Panamik and Puga), we sought to understand the light hydrocarbon consumption at different temperature and pH levels. In these experiments, slurry thermophiles from each site were transferred into the five bottles and then each bottle was closed with a butyl rubber cap followed by crimping with tin caps to make the bottles gas tight. Then, bottles were injected with 13C-labelled glucose solution. These experiments were terminated at 0, 1, 2, 3, 4 h intervals. The results demonstrate high 13C-labelled glucose uptake at sites with higher temperature at Puga than at those with lower temperature at Panamik site. The rate of 13C-labelled glucose uptake by the microbes of Panamik Hot Spring was 3.74–96.5 mmole C g−1 of dry sediment per hour. It increased by about 2.5 times the maximum original value after 2 h. Furthermore, the 13C-labelled glucose uptake in these thermophiles was compared to microbes engaging in photosynthesis and metabolic uptake at room temperature and pressure. The results (Joshi et al., Reference Joshi, Samanta, Tripathy and Rajamani2017) demonstrated a high rate of heterotrophy, allowing the maintenance of significant biomass in springs, and it was noted that the rate of heterotrophy increased with increase in temperature.
Saline lakes
Several saline lakes present in Ladakh's high-altitude, rain-shadowed region are potential analogues to lakes that existed on early Mars (>3 Ga), formed by evaporation of briny surface lakes (Osterloo et al., Reference Osterloo, Hamilton, Bandfield, Glotch, Baldridge, Christensen, Tornabene and Anderson2008; Osterloo et al., Reference Osterloo, Anderson, Hamilton and Hynek2010) and/or groundwater upwelling (e.g., Glotch et al., Reference Glotch, Bandfield, Tornabene, Jensen and Seelos2010). Over 600 small (~<25 km2) individual light-toned chloride deposits have been observed across the surface of Mars and appear to be associated with the fine-scale polygonal pattern, topographic lows and fluvial channels. They are relevant to planetary geomorphologists studying early Mars and have astrobiology significance in relation to the habitability of groups studying microbial life in briny environments and salt deposits. More recently, chloride salt deposits have been observed by the MRO's High-Resolution Imaging Science Experiment (HiRISE) in the Sinus Meridiani region Opportunity rover's landing site, in relation to a lake formed ca. 3.4 billion years ago, when dry and cold conditions were already established on Mars (Hynek et al., Reference Hynek, Osterloo and Kierein-Young2015). Thus, these evaporitic deposits could represent the last standing habitable water environment on the onset of dry and cold conditions on Mars (Hynek et al., Reference Hynek, Osterloo and Kierein-Young2015).
Site description
Kyagar Tso
Lake Kyagar Tso is located to the south of the town of Sumdo (Fig. 14). The River Puga, together with an unnamed stream, drains into this lake (Hedrick et al., Reference Hedrick, Seong, Owen, Caffee and Dietsch2011). Geologically, the lake is situated in the Puga gneiss complex. The lake catchment is dominated by the rocks of the Puga Formation which constitutes the lower part of the Tso Moriri crystallines. The Tso Moriri crystallines are comprised of quartzo-feldspathic gneisses, schistose bands and lenticular bodies of garnet amphibolites and eclogitic rocks. Quaternary alluvial deposits dominate the area. Lunette-shaped dunes, alluvial fans and interglacial gravel terraces surround the basin. Periglacial features including solifluction lobes and patterned ground are also present in the surrounding slopes and plains. The shoreline sediments were found to be colonized by cyanobacterial biomass, just a few millimetres below the crust.
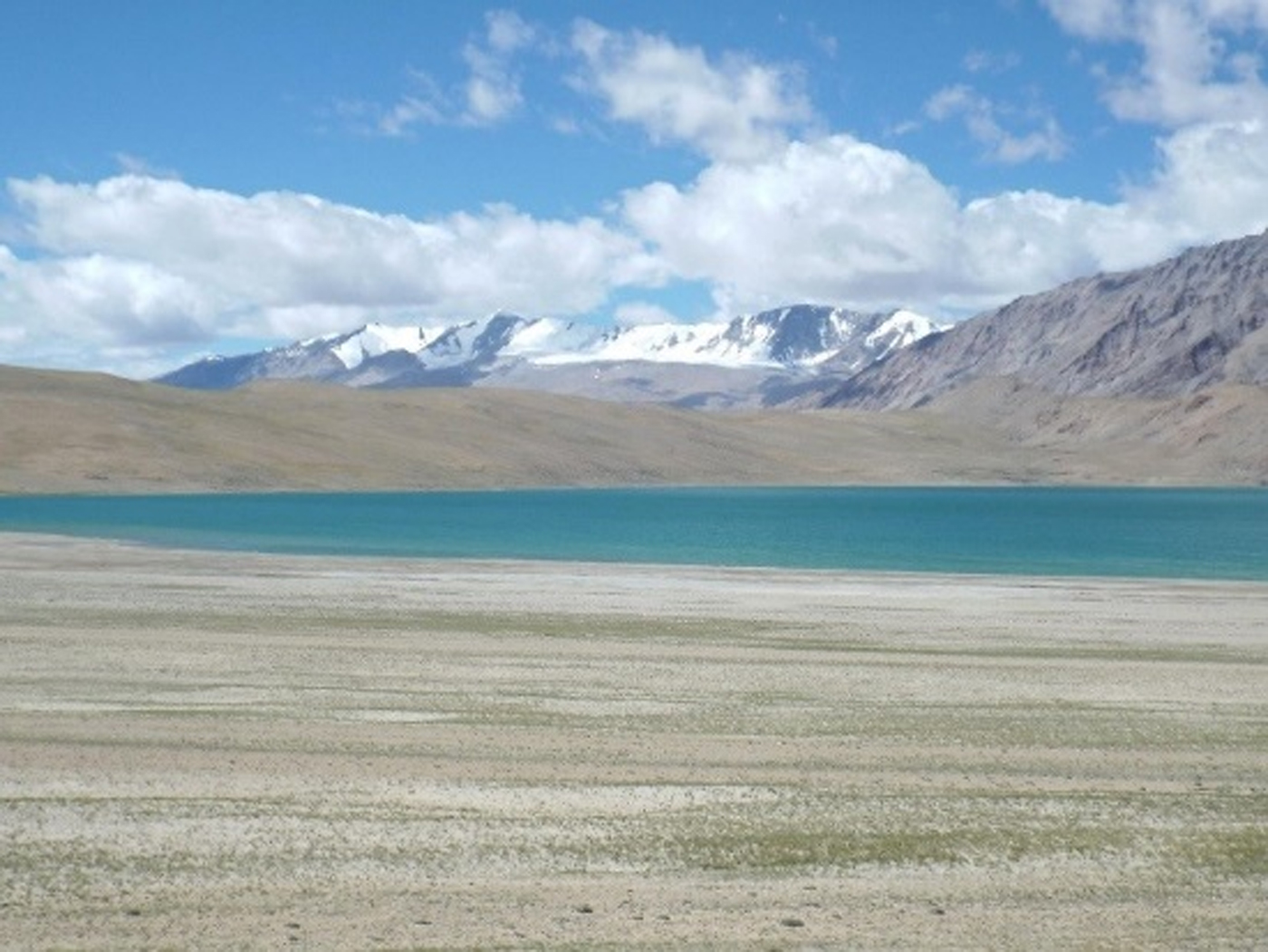
Fig. 14. Lake Kyagar Tso (Photo Credit: Dr Jonathan Clarke).
Tso-Kar
The region is characterized by extreme climatic conditions. Temperature varies from −40°C in winter to >30°C in summer, with extreme diurnal fluctuations, i.e. <0 to >30°C (Bhattacharyya, Reference Bhattacharyya1989; Philip and Mazari, Reference Philip and Mazari2000). Mean annual precipitation (rain or snow) is <90 mm. Tso-Kar Lake is a fluctuating shallow (1–3 m depth) salt lake characterized by high salinity with hypersaline (>50‰) extremes (TDS = 190.5 g L−1) of the Na–K–SO4-Cl type (Kramer et al., Reference Kramer, Kotlia and Wünnemann2014). Annual evaporation ranges from 400 to 600 mm year−1 (Yu et al., Reference Yu, Harrison and Xue2001) with dense brine observed in summer when evaporation is at its maximum (evaporation exceeds precipitation). The lake is inhabited by several species of ostracods with high tolerance to salinity extremes. This lake is situated within the Taglang La Formation of the Tso Moriri Crystalline Complex, assigned as an accreted unit of the ISZ (Fig. 15). The Taglang La Formation consists of metamorphosed calcareous, marly and argillaceous metasedimentaries together with concordant bands of amphibolites (Thakur, Reference Thakur1992). Thick piles of Quaternary lacustrine sediments are present as a cover deposit. The basin is tectonically bounded by the Tso-Kar fault to the west (N-S) and the Tso Moriri left lateral strike-slip fault (N-S) to the east. Lake Tso-Kar receives water through a shallow overflow of lake Startsapuk Tso, another river-fed lake, situated in the same catchment. Small glacial cirques at the north-eastern and south-western side of the basin, terminal moraines along the eastern side of the basin, a lateral moraine on the western side of the basin and hummocky landscape with polished and striated clasts are the distinct glacial landforms. Fluvial activity can be observed as the terminal moraines have the fluvial erosion signatures.

Fig. 15. (a) Tso-Kar Lake shoreline with abundant salt deposits (Photo Credit: Ms. Teresa Mendaza). (b) Tso-Kar salt flats (Photo Credit: Ms Anushree Shrivastava). (c) Pale pink-coloured microbial filaments on the Tso-Kar Lake shore. (d) Solifluction lobes on the hill to east side of Tso-Kar, zone of movement is 300 m wide. (e) Salt crust deposits near Tso-Kar. (f) Google Earth Image depicting rock glaciers on the north side of the valley between Puga and Tso-Kar (Photo Credits for c, d, e and f: Dr Jonathan Clarke).
Another striking feature is the raised palaeo-shorelines (highest +98 m from present lake level) at the northern and southern sides of the basin and surrounding the lake (Fig. 16(a)). They appear as elongated terrace-like ridges of a few decimetres in height, suggesting gradual shrinkage in lake level since its formation. The hydrological evolution and climate changes of the lake are very well studied and attributed to the interplay of climate and tectonics (Sekar et al., Reference Sekar, Rajagopalan and Bhattacharyya1994; Wünnemann et al., Reference Wünnemann, Reinhardt, Kotlia and Riedel2008, Reference Wünnemann, Demske, Tarasov, Kotlia, Reinhardt, Bloemendal, Diekmann, Hartmann, Krois, Riedel and Arya2010; Demske et al., Reference Demske, Tarasov, Wünnemann and Riedel2009). Thermokarst forms, frost mounds, possible 10 m high lithalsas, thermal contraction crack polygons, permafrost mounds with thick ice lenses and reticulate cryostructures are the features that demonstrate that this area undergoes geomorphic changes due to permafrost activity (Fig. 16(b)). However, investigations do support the existence of discontinuous permafrost due to the presence of many small- and medium-sized ponds near the frost mounds and the larger ones in isolated thermokarst depressions (Dimri et al., Reference Dimri, Baranwal and Biswas1983; Bhattacharyya, Reference Bhattacharyya1989; Wünnemann et al., Reference Wünnemann, Reinhardt, Kotlia and Riedel2008; Reference Wünnemann, Demske, Tarasov, Kotlia, Reinhardt, Bloemendal, Diekmann, Hartmann, Krois, Riedel and Arya2010).

Fig. 16. (a) Raised palaeo shorelines along the northern side of Tso-Kar Lake. (b) Thermokarst frost mounds near the northern side of Tso-Kar Lake (Photo Credits: Ms Johanna Bergstörm Roos).
Distribution of total active biomass along the Ladakh transect
The Ladakh transect was used as an opportunity to test life detection assays in a set of diverse extreme planetary-like environments. Life detection protocols are relevant for the preparation of future life detection missions to Mars, ocean Worlds and ice-covered Moons. Brines, waters and sediment/rock samples were co-analysed using the Adenosine 5′-triphosphate (ATP) Luminometry Assay and the Lymulus Amebocyte Assay (LAL) to determine the amount of lipopolysaccharides (LPSs). Both these biomarkers are proxies for recent biological activity and active biomass distribution across the study sites (Fig. 15).
ATP Luminometry Assay
This assay detects the total ATP, which is the universal energy carrier in nature, common to all living cells. Because ATP degrades rapidly after cellular death and disruption, if detected, ATP is a proxy for total active (or recently active) biomass. The assay is based on firefly (Photinus pyralis) luciferase that catalyses the oxidation of D-luciferin if ATP and oxygen are present.
ATP + d-Luciferin + O2 + Luciferase (Mg2 + )→Oxyluciferin + AMP + Pyrophosphate + CO2 + hv (560 nm)
The luminometer measures the light emitted by the luciferin–luciferase enzymatic reactions binding with the ATP released by living cells. Lighting events are translated into Relative Luminosity Units (RLUs) in proportion to the amount of ATP in the sample (DeLuca and McElroy, Reference Deluca and McElroy1978). The raw RLUs were converted into concentrations of ATP biomarker using a calibration curve and a Na2ATP salt standard (BioControl, N. 64004-25), e.g., 1 × 10−13 moles of lyophilized adenosine triphosphate is equivalent to 37 326– 42 151 RLU (N = 5). The sensitivity or limit of detection (LOD) of this assay is 1–1 000 000 fmole (1 fmole = 1 × 10–15 mole) (Hygiena comparison chart, 2006).
The ATP concentration can be further converted into total biomass based on the published values of ATP cellular equivalent. For instance, the minimum and maximum total cell abundance is calculated by assuming published values of 4 × 10−17 g ATP (Egeberg, Reference Egeberg2000). Field protocols were applied to both liquid (water and brines) and solid samples. The analysis of sediment particles involved direct loading of 5–25 mg of homogenized material onto the swab devices. Silt to clay-sized sample particles were swabbed from the internal surface of the sterile sampling bag till the entire sample was loaded onto the swab surface. These solid samples were re-analysed in the laboratory at Birbal Sahni Institute of Palaeosciences (BSIP), Lucknow.
For the analysis of liquid samples, 100 µL aliquots were directly pipetted into the liquid sampling device. To achieve the best signal/noise ratio, dilution protocols were applied to the analysis of brines and hot spring liquid samples, which were diluted 100–1000 times in doubled distilled water (DDW) to achieve the best signal/noise ratio. Triplicate ATP analyses were performed with appropriate control standard as well as positive and negative controls to mitigate false positives and false negatives. The analysis of liquid samples was performed in the field immediately, or shortly after collection.
LAL assay
The LAL assay detects LPSs, which are primary components in the cell walls of all Gram-negative-like microbes including active, dormant or dead cells. The LAL chromogenic assay has been extensively used for quality control of pyrogens (Lipid A) in drugs. More recent, the LAL assay has been applied to bioburden monitoring in spacecraft (NASA Planetary Protection standard practices, NPR 5340, 2007), during field astrobiology trials (Eigenbrode et al., Reference Eigenbrode, Benning, Tobler, Rodriguez-Blanco, Fogel, Amundsen, Callahan, Dworkin, Glamoclija, Glavin, Kerr, Kish, Mahaffy, McAdam, Steele and Voytek2010), and has been proposed for life detection sensors for future planetary missions. The assay has been applied in biologically low biomass rocks and minerals (≤102 cell equivalent g−1) as well as to biomass-rich brines, sediments and soils, i.e., ~109 cell equivalent g−1 (Bonaccorsi et al., Reference Bonaccorsi, McKay and Chen2010).
LPS samples (N = 25) were extracted under aseptic conditions; ~1 g of the mineral was homogenized and mixed with ~3.5 mL of DDW. The solution was subsequently vortexed (2 min), sonicated (10 min at 40°C) and centrifuged at high speed (6400 g) for 15 min. At the end of each extraction cycle, the LPS-enriched supernatant was transferred in a new 15 mL vial, while the LPS-leached solid residue (pellet) underwent further extraction. This procedure was repeated three times to ensure cells’ breakage, fragmentation of the LPS-bearing cells' membrane, the LPS dislodgement, its homogenization, thus to increase the analyte's concentration and maximize its detection. The final 11 mL solution was centrifuged one last time for 20 min to obtain a crystal-clear supernatant. Four 25 µL aliquots of this solution were pipetted into a laboratory-on-a chip cartridge (sensitivity range 0.5–0.005 and 1.0–0.01 EU mL−1) and analysed with a Portable Test System (PTS) spectrophotometer (405–410 nm). The chip has four ports receiving the liquid sample, two for spiked and two for non-spiked sub-aliquot samples. The resulting Endotoxin Unit (EU), 1EU = 1 × 105 cell equivalent mL−1 of Escherichia coli, is translated into nanograms of LPS (ng mL−1) using calibration curves built in the PTS's software. The current practical LOD for the LAL assay is 0.005 EUs, equivalent to (5 × 10−13 g of LPS) per mL of water or ca. 500 cells mL−1. These protocols have been developed by co-author Bonaccorsi during previous field expeditions to extreme environments (Bonaccorsi and Stoker, Reference Bonaccorsi and Stoker2008; Bonaccorsi et al., Reference Bonaccorsi, McKay and Chen2010).
Results and discussions
The LAL and ATP assays were successfully used in the field to determine the distribution of living biomass across the Leh–Ladakh system. Results are summarized in Fig. 17. Overall, 25 samples were assayed for LAL, while 29 were assayed for ATP. Only 16 samples out of a pool of 38 were co-analysed for both biomarkers. As showed in Fig. 17B, ATP correlates well with the LPS amount within each study environment for Hunder System, hypersaline lakes and shorelines, Tso-Kar Permafrost and hot springs (R2 = 0.5–1). However, there is no significant correlation between ATP and LPS (R2 = 0.045, N = 16) when considering the whole dataset. Furthermore, the variation of ATP and LPS biomarkers can be different such as across five different inter-dune ponds in Hunder; specifically, the ATP concentration was extremely variable (211 ± 202 fmol mL−1; N = 5) in contrast to the fairly constant LPS content in randomly sampled ponds (2.52 ± 0.09 ng mL−1; N = 3).

Fig. 17. (a) Concentration distribution of LPS and ATP biomarkers in collected samples. (b) Plot showing positive correlations between LPS and ATP biomarker pools for each assessed environment. Only 17 samples co-analysed for both biomarkers – out of the total dataset (Fig. 17(a)) – are plotted.
Overall, across the Ladakh transect, the LAL assay yielded values of lipid biomarker (LPSs) between 2.5 and ~5 × 103 ng mL−1 in water and brines and ~16–1.1 × 105 ng of LPSs per gram (ng g−1) in sediments and rocks. More specifically, the distribution of ATP biomarkers is mostly like the LPS one. The highest concentrations were measured in briny and clay-rich environments in the Tso-Kar region, particularly the brines from Tso-Kar Lake (104 fmol mL−1 ATP and 4.9 × 105 ng mL−1 LPSs), its cyanobacteria-rich sand-to-gravel supported shorelines (~5.0 × 105 fmol g−1 ATP and 1.11 × 105 ng g−1 LPS) and the clay-rich permafrost (104 fmol g−1 ATP and 4.33 × 105 ng g−1 LPS). The lowest concentration of total ATP was detected in a few hot spring water (test bed samples) and freshwater pond/stream environments (0.0–6.0 – 11 fmol mL−1 ATP and 2–3 ng mL−1 LPS) (Fig. 17(a)).
The lowest concentrations of measurable LPSs were found in the water samples from hot springs and freshwater ponds/stream environments; the highest concentrations were measured in the brines of the Tso-Kar Lake (i.e. 490 × 103 ng mL−1), the cyanobacteria-colonized shoreline (ca. 111 × 103 ng g−1 LPS) and the permafrost-associated ice-mud grains mix, particularly in the clay-supported rounded particles embedded in the permafrost's ice lenses (i.e. 433 × 103 ng g−1 LPS).
Glacial high passes
To explore the parametric biological content of the most extreme high elevation desert, representative aliquots of composite samples were assayed from two contrasting surface settings (0–2 cm-depth) on the North-facing slope at Taglang La. The first sampled site, a moss-free, dark-toned quartz-rich micaceous regolith from solifluction lobes (Fig. 5(b)) contained a 2.5-fold more lipid biomarker (39 ng g−1 LPS) than the carbonaceous (limestone) material (ca. 16 ng g−1, LPS) atop the light-toned vegetation-barren outcrops (Fig. 5(b)). It is unknown whether this difference is significant or related to actual environmental differences (nearby moss-colonized patches, higher ground moisture, different regolith's composition) rather than the non-systematic sampling effort (just one sample from each lithology was analysed). In any case, the hyper-arid contrasting soil types from Taglang La contain 39 ng of lipid biomarker per gram (ng g−1). The two different contrasting bedrock lithologies from Taglang La may contain a different living microbial ecology to be tested in future studies. In both cases, the low biomass measured was consistent with the aridity/(hyper-aridity?) observed at this location, i.e., vegetation-barren, mechanically weathered regolith with estimated top soil water content <2–5%, RH, air <5–10% associated. Interestingly, Taglang La's rocky desert might contain biomass levels comparable to those in oligotrophic deserts worldwide (Antarctic Dry Valleys, Atacama and the Mojave Desert). Specifically, similar levels of LPS were found by Bonaccorsi et al. (Reference Bonaccorsi, McKay and Chen2010) using similar LAL protocols: ~0.4–2.4 ng g−1 in arid and hyper-arid core of the Atacama Desert (precipitation ~2–11 mm year−1) and ~44–140 ng g−1 in volcanically-derived soil from the arid Death Valley (precipitation: ~40–250 mm year−1).
Hunder dunes and ephemeral ponds
The distribution of LPS and ATP biomarker was evaluated across several subsystem environments within the Hunder dune field. A total of 14 different samples (Fig. 17) were taken from dry sand dunes' crest and bottom (HU-Pond_sand, N = 3), inter-dune ponds (HU-Pond_W, N = 7), pond's underwater sediments (N = 1), wind eroded, dry mud-cracked horizons, soaked mud from the pond's shoreline (N = 2). The ATP biomarker across the six investigated ponds was quite variable (30–531 fmol mL−1), plausibly in relationship with the fluctuating level of active biomass present in the ephemeral water (i.e., clear water versus organic debris-rich water); in contrast, the Lipid A content resulted fairly constant across three different ponds (Fig. 17A). The lowest content of ATP biomass measured in pond samples was comparable to 32 fmol mL−1 ATP measured in the single sample from the Skyok River (HU_river_w) across the dune field. When considering the LPS variability across and individual pond system, Hunder dune's short-lived fresh water in pools contains ca. 2.5 ng of lipid biomarker per millilitre (ng mL−1) with LPS biomarkers concentrated in the underwater sediments (17 ng g−1 LPS and 823 fmol g−1 ATP). The surrounding clay beds preserve 1000 times more biomarkers (lipid biomarker concentrations of 9–165 × 103 ng g−1 LPS and 1329 fmol g−1 ATP) than the encasing sand dunes (~19–112 fmol g−1 ATP and ~4–8 ng g−1 LPS) (4.18.2 ng g−1) given the propensity for clay to preserve organics. On Earth as well as on Mars, the reactivity between regolith's minerals and organics affects preservation and detection of organic matter and the direct evidence of life (e.g., Klein et al., Reference Klein, Horowitz, Levin, Oyama, Lederberg, Rich, Hubbard, Hobby, Straat, Berdahl, Carle, Brown and Johnson1976; Navarro-González et al., Reference Navarro-González, Vargas, de la Rosa, Raga and McKay2010). Organics are poorly preserved in oxidising environments (Bonaccorsi et al., Reference Bonaccorsi, McKay and Chen2010; Davila et al., Reference Davila, Gross, Marzo, Fairén, Kneissl, McKay and Dohm2011), as well as porous and coarser-grained materials (sands, gravels, sandstones), but they are in clay-rich deposits against oxidation (e.g., Hedges and Keil, Reference Hedges and Keil1995; Bonaccorsi and Stoker, Reference Bonaccorsi and Stoker2008). Dissolved organics adsorbed on mineral surfaces (D'Acqui et al., Reference D'Acqui, Daniele, Fornasier, Radaelli and Ristorri1998; Kleber et al., Reference Kleber, Mikutta, Torn and Jahn2005; Kaplan et al., Reference Kaplan, Milliken, Knoll, Bristow and Knowlton2014) can be preserved against microbial decay (e.g. Mayer Reference Manga, Patel, Dufek and Kite1994; Bock and Mayer, Reference Bock and Mayer2000; Aggarwal et al., Reference Aggarwal, Li and Brian2006; Yu et al., Reference Yu, Dong, Jiang, Guo, Eberl, Li and Kim2009). Clay-bearing deposits, including the mid Hesperian Sheepbed unit (Thomson et al., Reference Thomson, Bridges, Milliken, Baldridge, Hook, Crowley, Marion, de Souza Filho, Brown and Weitz2011; Grotzinger et al., Reference Grotzinger, Sumner and Kah2014), have been targeted by the NASA Mars Science Laboratory Curiosity mission in Gale Crater for sampling, because of their expected high habitability potential (related to liquid water) and preservation of organics (e.g., Farmer and DesMarais Reference Farmer and DesMarais1999; Grotzinger et al., Reference Grotzinger, Sumner and Kah2014). It is thought that Sheepbed clay deposits would have formed in relatively pH-neutral surface freshwater during a period of increasing aridity, yet characterized by episodic rainfall (Barnhart et al., Reference Barnhart, Howard and Moore2009; Andrews-Hanna and Lewis, Reference Andrews-Hanna and Lewis2011). The role of multiple short-lived wetting events in the formation of sedimentary clay minerals is not well understood (e.g. Milliken and Bish, Reference Milliken and Bish2010; Ehlmann et al., Reference Ehlmann, Berger, Mangold, Michalski, Catling, Ruff, Chassefière, Niles, Chevrier and Poulet2013), but we know that terrestrial smectite clays can only form under dry, i.e., mean annual precipitation <50 cm year−1, and circum-neutral/alkaline, i.e., pH 6.5–7.8, conditions (Schiffman et al., Reference Schiffman, Howard, Southard and Swanson2000).
Hot springs
Because of high temperatures and the presence of geothermal minerals (e.g., Singh et al., Reference Singh, Tiwari and Singh2010) in situ life detection in hot springs environment can be very challenging and at the possible lowest end of the detection limit of any life detection instrumentation. Therefore, a few water, sediments and rock samples were analysed in order to develop protocols suitable for life detection of ultra-low biomass (oligotrophic) in hydrothermal mineral-rich environments, and water chemistries potentially interfering with the wet chemistry of the biological assays (low signal-to-noise ratio). The sample suite consisted of four water samples and one sediment sample from Panamik (PAK-W 1, 2 and 4; PAK-S-4); one water sample (CHUM-W-2) and a composite rock sample (CHUM-cyano-rock) gathered from outcrops nearby Chumatang Hot Springs; and a desiccated mineral deposit sample from the Puga compound (PUG-S-rich_grnd). The concentration of total ATP in four water samples from Panamik ranged from ca. 6.0 to 11 fmoles mL−1 in samples PAK-W-1 and PAK-W-2, respectively, with the exception of water taken from the hottest spot Site 4 (PAK-W- 4, N = 5), which yielded non-measurable ATP lipid (below LOD <0.2 fmoles mL−1). The same water sample, however, contained low but measurable LPS (2.90 ± 0.13 ng mL−1; N = 4), yet, one of the lowest concentrations detected across the entire transect. The above results, as well as other measurements from several sets of geological samples, demonstrate that the LAL assay can provide a reproducible estimate of LPS-based biomass in complex environmental materials. Interestingly, the associated sediment slur (PAK-S-4, N = 5) contained 2.5 ng g−1 LPS and 9.0 fmoles g−1 of total ATP, suggesting that living biomass (or residual ATP biomarker) is better concentrated/preserved in the muddy slur. This result is consistent with the lowest rate of 13C-labelled glucose uptake by the microbes of Panamik Hot Spring (3.74 mmole C g−1 of dry sediment per hour) (~74°C, pH = 7.7) co-analysed for ATP.
By comparison, the water sample from Chumatang yielded higher amounts of LPSs (13 ng mL−1) and ATP (33.2 fmol mL−1) than Panamik's and displayed the same range of biomass of the freshwater sample from the Shyok River (~33.4 fmol mL−1) in the Hunder region. The LPS content of outcrops and gravelly supported grounds around Chumathang Hot Spring was also high e.g. 7650 ng g−1, in relation to endolithic and chiasmolithic microorganisms colonising within the minerals' grains.
We did not attempt to perform systematic measurements of the biomarker distribution in the Puga Hot Spring, which is beyond the scope of this work; rather, we analysed sulphur-laden dirt as a test bed for possible life detection in relevant sulphur-rich planetary surfaces. This sulphur-rich ground sample (dry) yielded the lowest amount of measurable LPS (0.38 ng g−1). The attempted measurement was extremely challenging as it yielded several false negatives (N = 6), plausibly due to a combination of ultra-low biomass, originally present in the dry dirt, and sulphur chemistry interfering with the LAL assay.
Alkaline brine lakes
To preliminarily assess the total biomass amount distribution at the Tso-Kar Lake, we analysed six samples across a traverse from hyper saline-dominated to fresh water-dominated environments. The former, proximal to the shoreline, comprised brines, greenish-grey sand and dark grey mud at the interface with, or beneath the evaporitic crust (Fig. 15(b) and (e)). The latter, 200–300 m away from the shoreline, consisted of cyanobacteria-colonized glacial debris and glacial melt water-sourced ponds nestled in a field of moss-colonized bogs/peats. The biomarker concentration of Tso-Kar brines (2.5 × 103 ng mL−1 LPS; 3 × 104 fmol mL−1 ATP) resulted three to four orders magnitude higher than that of water samples (N = 2) from the Kyagar Tso/Sumbdo Lake, i.e, ~2 ng mL−1 LPS; 35–80 fmol mL−1 ATP (Fig. 17(a)). It is unclear whether or not the remarkable difference in biomarker content between the samples from the two lakes reflects the opportunistic sampling (not a significant set of samples to draft any conclusion) rather than (i) a higher preservation of ATP and LPS biomarker in brines versus salty water, (ii) an actual presence of living biomass or (iii) a combination of both. Interestingly, the LPS content of clays versus non-clay materials beneath the evaporitic deposits, sandy to silty-clay shoreline sediments ranges from ~23 ng g−1 (sand) to 6400 ng g−1 (mud), consistently with the preferential preservation of organics in clays. Further away from the shoreline, melt water (freshwater) flushed sediment contains the highest amount of biomarkers of the entire transect (~5.0 × 105 fmol g−1 ATP; ~105 ng g−1 LPS), plausibly in relation to the cryptic, but widely distributed thin layer of living cyanobacterial biomass hiding just a few millimetres below the periglacial debris. Comparatively, the cyanobacteria-colonized distal sands contained a three-order magnitude higher ATP (467 261 fmoles g−1, ca. 4.7 × 105) than the proximal shoreline sand (~700 fmoles g−1 ATP, or 7.0 × 102), or the freshwater ponds in the bogs around the lake (264 or 2.6 × 102 fmol mL−1 ATP).
Wet chemistry-based life detection assay of complex evaporite systems – including briny extreme salinity environments (TDS = 190.5 g L−1) (Kramer et al., Reference Kramer, Kotlia and Wünnemann2014), produced quite challenging results. First, hyper-saline environments can be poorly conducive to microbial life (refs. here), therefore, hosting low to very low levels of biomass (Fig. 15) requiring detection assays with high sensitivity (i.e., ultra low LOD). Second, independently by the specific content of microbial life in brines, life detection in evaporitic environments is jeopardized by possible chemico-physical interferences between the salts and the aqueous chemistry of the bioassays in question. The latter issue produced particularly relevant results during our first attempt to analyse evaporites containing obvious life, such as the pink coloured microbial filaments on the shoreline of Tso-Kar Lake (Fig. 14). Non-diluted Na–K–SO4-Cl dense brine samples from this environment yielded no biomarkers (false negatives), but the highest biomass was instead detected as soon as the same sample was diluted 100–1000 times with distilled water (Fig. 15(a)–(e)). The Tso-Kar Lake could represent a big sink system of organic matter and living biomass from distal regions around the basin and supported by glacier meltwater (source). This can be explained by the source to sink processes of cyanobacteria-associated biomarker. The Tso-Kar Lake ecosystem displayed very high levels of cyanobacterial biomass possibly supported by glacier meltwater. The habitability potential is enhanced by this source of freshwater flushing amounts of loose cyanobacterial biomass from the colonized shorelines into the Tso-Kar Lake basin. It is possible that the high level of total biomass we found in the lake brines – and the associated lacustrine mud – represents the sink of the freshwater-supported living biomass flushed into the lake by glacier meltwater. At this stage, it is not possible to determine whether the total ATP measured in the lake is ATP biomarker (extracellular free ATP) preserved in salts and clays, rather than microbial ATP from intact and/or living cells and spores (microbial ATP). Further investigation is warranted to address the distribution of living biomass in the brine ecosystem. Saline lakes existed on ancient Mars and their palaeo deposits may still preserve lipid biomarkers as signatures for ancient life.
Tso-Kar permafrost region
The permafrost mound along the Tso-Kar palaeo-shoreline included cryo structures such as clear lenses of ice, in a matrix of dark grey icy mud matted with patches of yellowish silt-clay–ice frozen mix. In each matrix, grey and yellowish frozen mud and ice lenses were sampled at dm to sub-cm scale and contained biomarkers concentrations ranging from 103 to 104 magnitude, respectively, i.e., 4.9 × 102–4.33 × 105 ng g−1 LPSs (N = 2), and 8.6–~2 × 104 fmol mL−1 ATP (N = 5). Specifically, the dark-grey muddy melt (Site 1B) contained five times lower amount of total ATP (85.6 fmol mL−1), compared to the clear ice melt water (441 fmol mL−1 ATP) from the dark grey permafrost matrix (Site 1D). The other material embedded in the permafrost matrix (N = 3) were yellowish mud grains with very high concentrations of ATP (~1 × 104–2 × 104 fmol g−1 N = 3) and LPS (433 350 ng g−1), which represent the highest amount measured across the whole transect (Fig. 17(b)). The analysis of two sub aliquots from the same subsample (TSO-KA-PMF_A_mud) yielded reproducible values (9820 and 9970 fmol g−1), while the assay of another whole individual grain yielded a twofold amount of ATP (20 260 fmol g−1). These mud particles differed from the embedding dark grey permafrost matrix by colour (yellowish), texture – high cohesive, retained after the permafrost melted up, and high content of living biomass, or preserved biomarkers. These mud particles resulted very similar to sub-cm semi-rounded particles, referred as at to Mud Grains, or MDGS founded ice-bounded and/or glaciogenic sediments and glacial-marine sediments scoured by glaciers/ice streams from the Antarctic subglacial interiors (e.g., Kluiving et al., Reference Kluiving, Bartek and Van der Wateren1999; Bonaccorsi et al., Reference Bonaccorsi, Burckle, Brambati, Piotrowski, Quaia, Finocchiaro and Piani2000; Reference Bonaccorsi, Burckle, Brambati, Piotrowski and Hoover2005, and refs. therein). Mud-supported particles were identified in the Ross Ice Shelf (RIS) sea-ice and sediment cores (Webb et al., Reference Webb, Ronan and Lipps1979; Zotikov and Jacobs, Reference Zotikov and Jacobs1985), ice cores from Antarctica and Greenland (Gow and Meese, Reference Gow and Meese1996) and Lake Vostok ice core's basal sediments (Jouzel et al., Reference Jouzel, Petit, Souchez, Barkov, Lipenkov, Raynaud, Stievenard, Vassiliev, Verbeke and Vimeux1999) as well. Overall, their unique features point to ‘freeze in’ processes aiding the concentration of organic biomarkers closely coupled to ice stream erosional/depositional processes occurring at the ice-sheet/sediment interfaces (Bonaccorsi et al., Reference Bonaccorsi, Burckle, Brambati, Piotrowski and Hoover2005), which could be also the case for the Tso-Kar cryostructures. Detecting biomarkers in ice-bound clays in permafrost's reticulate cryostructures, or other ice-bound environments, offers a significant opportunity to train for life detection missions to the polar and mid-latitude regions of Mars, or to the ice sheet-dominated surface regions on Ocean Worlds, where similar biomarker-concentrating ice–non-ice mix fine-grained materials could be accessed for sampling. Non polar ice deposits are present at mid-latitudes on Mars and appear more widespread and common that previously believed.
Education and public engagement
This expedition was a follow-up of the Spaceward Bound expedition series (initiated at the NASA Ames Research Center in 2006) regularly held within the USA and other countries with a special focus on education outreach and fieldwork training of participating international and local educators and students (e.g. Bonaccorsi et al., Reference Bonaccorsi, McKay, Mogul, Boston, Willson, Heldmann, Baker, Cowan, Pandey, Sharma, Sun, Blank, Stoker, Mogosanu, Campbell, Phartiyal, Rask and Clarke2017). The expedition facilitated collaboration between Indian and international research groups in the field and in the laboratory. As a key element of this expedition, the participants engaged in initial discussions towards the formation of an astrobiology research roadmap for India. Towards this, the team members engaged in end-of-day discussions conducted hands-on science projects with Ladakhi students from nomadic village primary schools, and interacted with undergraduate science and engineering students in New Delhi, at the end of the expedition.
Conclusions
The 2016 multidisciplinary expedition to explore Ladakh was fundamental in assessing the astrobiological potential of the visited sites. Several site-specific experiments were conducted, both during and after the expedition which added to our understanding of the region. The main outcomes of the expedition are listed below:
• The high passes of Ladakh, Khardung La and Taglang La are unique analogues for early Mars with their combination of low atmospheric pressure, high UV radiated soils, permafrost and active periglacial activity, glaciers and glacier melt water pools. The environment in Khardung La supported deliquescent melting of calcium chloride salts based on tests conducted with the HABIT instrument prototype, designed for the ExoMars 2020 mission. The cold, desert environment of these passes also provided an ideal setting to develop and implement regolith-landform mapping methodology for these terrains.
• The Hunder region with its valley dunes and ephemeral pools provides an early Mars-like (Noachian or later) setting, an ideal environment for preservation of microorganisms. Rose diagrams generated using QGIS software indicated an unusual trend of seasonal dependency on dune orientation and wind direction due to wind changes being within 60° and mild weather variation due to the region. Such a technique could prove useful in understanding wind–surface interactions for regions on Mars or Earth where local weather information is not available.
• Ladakh hot springs of Panamik and Puga showed higher heterotrophy rates with significant biomass for higher discharge temperatures. The water samples also showed robust combinations of pertinent prebiotic mixtures that were stable at high temperatures and retained their functionality after multiple cycles of dehydration–rehydration.
• The saline lake shoreline sediments of Kyagar Tso and Tso-Kar were colonized by cyanobacteria just a few millimetres below the crust. The Tso-Kar Lake could represent a big sink system of organic matter and living biomass from distal cyanobacteria-colonized around the basin sustained by glacier meltwater (source).
• Permafrost activity, a unique phenomenon seen on Mars and rarely found on Earth was observed in the area. Tso-Kar permafrost ‘granulated’ cryo structures contained an unusually high amount of biomass. ‘Freeze in’ processes at the ice–sediment interface might be responsible for the concentration and storage of organic biomarkers in glacial sediments of planetary environments; thus suggesting glaciated areas as a primary target for life detection.
• Life detection assay methodology (ATP and LAL assays) was tested across the entire Ladakh transect, but the analysis of hypersaline extremes and clay-rich environments was affected by false negatives, jeopardising scientific discovery. Analytical protocols tested during the expeditions, also including dilution, resulted critical to the correct evaluation of biomass content in these planetary-like geological environments.
• This analysis method is relevant to several future off-Earth missions to worlds (Mars, Ocean Worlds and Icy Moons) that could potentially harbour life.
• The co-analysis of ATP and LPS biomarkers provided the first known measurement of total active biomass in the region. Tso-Kar evaporites and permafrost ice-bound clays contained the highest biomass, hot spring water samples, sand dunes and surface regolith from Tang La glacial high pass, the lowest. The clay beds associated with the inter-dune ponds showed 1000 times higher LPS amounts than the surrounding sands, given the propensity for clays to preserve organics.
• In regions of cold arid environments, HABIT discovered the existence of the interaction of the diurnal water cycle with salts. These can serve as liquid water reservoirs and be comparable to areas where ice is melting. This was confirmed by life detection assays finding the highest amount of LPS-based biomass in the brines of the Tso-Kar Lake (~5 × 105 ng mL−1) and in the clay-supported permafrost (i.e. ~4 × 105 ng g−1 LPS). Finally, the clay beds around the dune ponds showed 1000 times higher LPS amounts compared to adjacent sands.
• This campaign has confirmed the relevance of clays, brines and permafrost as interest targets of research on Mars for life detection and biomarker preservation as a proxy for recent and ancient life.
• Last but not least, as follow-up of the Spaceward Bound expedition series, the Ladakh Spaceward Bound expedition promoted collaboration between the US, Indian and international research groups; engaged local schools and communities in the exploration of the space and Mars; and sat up the stage for an astrobiology research roadmap for India.
Author ORCIDs
Siddharth Pandey, 0000-0002-4123-3949
Acknowledgements
The team would like to express its gratitude to Birbal Sahni Institute of Palaeosciences, Department of Science and Technology, Office of Chief Wildlife Warden of Ladakh, Government of India for helping arrange the requisite clearances and permits for the conducted work. Project mentoring and guidance provided by Spaceward Bound members at NASA Ames Research Center. Financial and logistics support provided by Tata Motors Ltd, Inspired Journeys Co, Pearl Travels Ltd and National Geographic Traveller India. Website and IT support provided by the Blue Marble Space Institute of Science. Audio-video documentation support provided by Astroproject India and The Highroad Co.