Introduction
Sustaining human outposts on Mars by providing life-support consumables from Earth is unrealistic mainly due to launch costs, travel times and failure risks. The development of Life Support Systems (LSS) is mandatory. On Earth, oxygenic photosynthesizers convert CO2 to organic matter which serves as food, and H2O to O2, processes that should play a key role in Biological Life Support Systems (BLSS) off the planet (Binot et al., Reference Binot, Tamponnet and Lasseur1994; Wheeler et al., Reference Wheeler, Mackowiak, Stutte, Yorio, Ruffe, Sager, Prince and Knott2008). An important aspect of LSS is the utilization of local materials, the so-called in-situ resource utilization (ISRU), although, to date, these technologies focused mainly on inorganic chemistry and building materials (Sridhar et al., Reference Sridhar, Finn and Kliss2000).
A link between local resources and BLSS components, otherwise unable to live ‘off the land’ might be provided by oxygenic phototrophs, such as cyanobacteria, thanks to the development of Bio-ISRU technologies (Verseux et al., Reference Verseux, Baqué, Lehto, de Vera, Rothschild and Billi2016; Brown, Reference Brown2018). Lithotrophic cyanobacteria have been proposed as ideal candidates for Bio-ISRU due to their capability of utilising rocks as a growth substrate (Olsson-Francis and Cockell, Reference Olsson-Francis and Cockell2010).
The concept of using cyanobacteria to feed bacteria has been approached by genetically modifying the cyanobacterium Anabaena sp. 7120 in order to secrete sucrose to grow Bacillus subtilis (Moss and Ho, Reference Moss and Ho2011; McCutcheon et al., Reference McCutcheon, Kent, Paulino-Lima, Pless, Ricco, Mazmanian, White, Tapio and Rothschild2015). This approach was further developed into the NASA PowerCell space experiment, on-board the DLR Eu:CROPIS satellite (Hauslage et al., Reference Hauslage, Strauch, Eßmann, Haag, Richter, Krüger, Stoltze, Becker, Nasir, Bornemann, Müller, Delovski, Berger, Rutczynska, Marsalek and Lebert2018), aimed to germinate Bacillus subtilis spores by using Anabaena lysate under simulated Moon and Martian gravity (McCutcheon et al., Reference McCutcheon, Kent, Paulino-Lima, Pless, Ricco, Mazmanian, White, Tapio and Rothschild2015).
It has been suggested that cyanobacteria could be used in BLSS using Martian local resources with relatively low hardware requirements and attenuation of surface conditions, such as low pressure and high flux of ionizing and UV radiation (Lehto et al., Reference Lehto, Lehto and Kanervo2006; Murukesan et al., Reference Murukesan, Leino, Mäenpää, Ståhle, Raksajit, Lehto, Allahverdiyeva-Rinne and Lehto2016). However, the presence in the Martian soil of a highly oxidizing agent such as perchlorate in the range of 0.4–0.6 wt % (Hecht et al., Reference Hecht, Kounaves, Quinn, West, Young, Ming, Catling, Clark, Boynton, Hoffman, DeFlores, Gospodinova, Kapit and Smith2009) provides an additional limiting factor for Bio-ISRU.
The exploitation of desert strains of Chroococcidiopsis to support human space exploration has been proposed taking advantage of their remarkable desiccation- and radiation-tolerance (Billi, Reference Billi2019). In particular, strain CCMEE 029 isolated from the Negev Desert has been extensively investigated and reported to survive years of desiccation (Billi, Reference Billi2009), as well as high doses of ionizing (Billi et al., Reference Billi, Friedmann, Hofer, Grilli-Caiola and Ocampo-Friedmann2000; Verseux et al., Reference Verseux, Baqué, Cifariello, Fagliarone, Raguse, Moeller and Billi2017) and UV radiation (Baqué et al., Reference Baqué, Viaggiu, Scalzi and Billi2013). In addition, strain CCMEE 029 was reported to cope with Mars-like conditions simulated in low Earth orbit, when exposed as dried cells mixed Martian regolith simulants (Billi et al., Reference Billi, Verseux, Fagliarone, Napoli, Baqué and de Vera2019a) or as dried biofilms (Billi et al., Reference Billi, Staibano, Verseux, Fagliarone, Mosca, Baqué, Rabbow and Rettberg2019b).
Although the strategies to withstand the above mentioned conditions have not been fully deciphered, for Chroococcidiopsis sp. CCMEE 029 the following mechanisms have been identified: (i) avoidance of protein oxidation during air-drying and ionizing radiation (Fagliarone et al., Reference Fagliarone, Mosca, Ubaldi, Verseux, Baqué, Wilmotte and Billi2017); (ii) accumulation in response to desiccation of sucrose and trehalose that stabilize dried sub-cellular components, including ribosomal RNA and mRNAs codifying DNA repair proteins (Mosca et al., Reference Mosca, Rothschild, Napoli, Ferré, Pietrosanto, Fagliarone, Baqué, Rabbow, Rettberg and Billi2019; Fagliarone et al., Reference Fagliarone, Napoli, Chiavarini, Baqué, de Vera and Billi2020); and (iii) over-expression of DNA repair genes upon rehydration of dried cells exposed to a Mars-like UV flux (Mosca et al., Reference Mosca, Rothschild, Napoli, Ferré, Pietrosanto, Fagliarone, Baqué, Rabbow, Rettberg and Billi2019).
In the present work, we sought to address the hypothesis that as a consequence of its efficient antioxidant system, Chroococcidiopsis sp. CCMEE 029 might be perchlorate resistant and therefore a good candidate for Bio-ISRU on Mars. To test this hypothesis, we selected strain CCMEE 029 and its derivate CCMEE 029 P-MRS obtained from dried cells mixed with Phyllosilicatic Martian Regolith Simulant (P-MRS) and exposed to Mars simulations during the BIOMEX (BIOlogy and Mars EXperiment) space experiment performed during the EXPOSE-R2 space mission (Billi et al., Reference Billi, Verseux, Fagliarone, Napoli, Baqué and de Vera2019a). The perchlorate tolerance threshold of the two strains was identified by monitoring the growth rate during a 55-day exposure to 5, 50 and 100 mM Na-, Mg- and Ca-perchlorate. Once their perchlorate tolerance threshold was identified, the two strains were grown for 40 days in the presence of Mars-relevant perchlorate concentration, for example, 2.4 mM perchlorate ions supplied as a 60 and 40% mixture of Mg- and Ca-perchlorate, respectively, as reported by the NASA's Phoenix Mars Lander (Hassler et al., Reference Hassler, Zeitlin, Wimmer-Schweingruber, Ehresmann, Rafkin, Eigenbrode, Brinza, Weigle, Böttcher, Böhm, Burmeister, Guo, Köhler, Martin, Reitz, Cucinotta, Kim, Grinspoon, Bullock, Posner, Gómez-Elvira, Vasavada and Grotzinger2014). Then the biomass yielded by strain CCMEE 029 grown in 2.4 mM perchlorate ions was used to produce the lysate used to feed an E. coli strain capable of metabolizing sucrose.
Material and methods
Organisms and culture conditions
Chroococcidiopsis sp. CCMEE 029 was isolated by Roseli Ocampo-Friedmann from cryptoendolithic growth in the Negev Desert (Israel). The strain is part of the Culture Collection of Microorganisms from Extreme Environments (CCMEE), established by E. Imre and Roseli Ocampo-Friedmann, that is currently maintained at the Department of Biology, University of Rome Tor Vergata. Chroococcidiopsis sp. CCMEE 029 P-MRS was derived from dried cells of CCMEE 029 mixed with P-MRS and exposed to Mars simulations in low Earth orbit inside the EXPOSE-R2 facility: CO2 atmosphere (780 Pa), 2.19 × 102 kJ m−2 of UV200−400 nm radiation and 0.5 Gy of ionizing radiation (Billi et al., Reference Billi, Verseux, Fagliarone, Napoli, Baqué and de Vera2019a). Cyanobacterial strains were grown in BG11 medium (Rippka et al., Reference Rippka, Deruelles, Waterbury, Herdman and Stanier1979) by using 50 ml vented flasks, inside an incubator at 25°C, without shaking, under a photon flux density of 40 μmol m−2 s−1 provided by cool-white fluorescent lamp (4100 K) under continuous illumination.
Escherichia coli strain W (ATCC 9637) was purchased from the American Type Culture Collection (Manassas, VA, USA) and grown in Luria-Bertani broth (Sambrook et al., Reference Sambrook, Fritsch and Maniatis1989) at 37°C with orbital shaking.
Monitoring of growth rates
Cyanobacterial growth was monitored by determining the optical density at 730 nm (OD730) of triplicates, each one consisting of 100 μl aliquot. Bacterial growth was determined by measuring the optical density at 600 nm (OD600) of triplicates, each one consisting of 100 μl aliquot. Two calibration curves, one for OD730 nm versus cyanobacterial concentration and one for OD600 nm versus bacterial concentration, were obtained by determining cell concentrations (cells ml−1) with a Burker's chamber.
Determination of cyanobacterial perchlorate tolerance
One-ml aliquots of the two cyanobacterial cultures in the early stationary phase (about 1 × 107 cells) were centrifuged and pellets inoculated into 50 ml vented flasks containing 10 ml of liquid BG-11 with 5, 50 and 100 mM Na-, Mg- and Ca-perchlorate. Controls were obtained by inoculating 1 ml aliquots in liquid BG-11. During the 55 days of growth, the culture volume was kept constant by adding BG-11 or BG-11 with 5, 50 and 100 mM Na-, Mg- and Ca-perchlorate. The experiment was performed in triplicate.
Cyanobacterial growth in Mars-relevant perchlorate
One-ml aliquots of the two cyanobacterial cultures in the early stationary phase (about 1 × 107 cells) were centrifuged, and the pellets inoculated into 50 ml vented flasks containing 10 ml of BG-11 with 2.4 mM ClO4− provided as a 40% Mg-perchlorate and 60% Ca-perchlorate mixture. Each experiment was performed in triplicate. During the 40 days of growth, the culture volume was maintained constant by adding BG-11 or BG-11 with 2.4 mM ClO4. The experiment was performed in triplicate.
Cyanobacterial lysis
Six-ml aliquots (about 6 × 108 cells ml−1) obtained from 40-day-old cultures in BG-11 and BG-11 containing 2.4 mM ClO4− (as reported above) were centrifuged at 7000 g, at 20°C for 15 min. Pellets were washed twice in dd-H2O, air-dried overnight under a laminar-flow hood, and weighted prior to and after drying. Air-dried cells (30 mg) were resuspended in 3 ml dd-H2O and split into three aliquots. Pellets from each 1 ml aliquot were lysed as follows: (i) mortaring for 2 min, (ii) resuspension in 500 μl dd-H2O and immersion in liquid N2 for 1 min, (iii) thawing at 37°C for 10 min; (iv) centrifugation at 6000 g, for 10 min at 20°C to collect the soluble supernatant used as lysate medium.
Escherichia coli growth with cyanobacterial lysate-based medium
One-ml aliquots of overnight E. coli were diluted to about 1 × 106 cells ml−1, washed with 1X PBS, and resuspended in 1 ml lysate-based medium. As a positive control, 1 × 106 cells ml−1 were washed with PBS and inoculated in 1 ml of M9 minimal medium (Sambrook et al., Reference Sambrook, Fritsch and Maniatis1989) supplemented with 0.5% glucose. As negative controls, about 1 × 106 cells ml−1 were washed with PBS and inoculated into 1 ml of sterile dd-H2O and 1X PBS. Each sample was incubated in 1.5 ml Eppendorf tubes at 38°C overnight, under continuous shaking of 180 rpm. The experiment was performed in triplicate.
Perchlorate measurements
Perchlorate concentrations were determined by EPA Method 331.0 Rev. 1.0 – Liquid Chromatography/Electrospray Ionization/Mass Spectrometry (2012). Briefly, samples were diluted 1:20 with ultrapure H2O and 5 μl injected for analysis on a Waters ACQUITY I-Class UPLC/Xevo G2-XS QTof system. The quantification of perchlorate anion was based on Tof MRM negative acquisition mode of the following masses, under −25 V collision energy and by using a calibration curve that covered 0.01–10 mM concentration interval.
Results
Cyanobacterial tolerance towards increasing perchlorate concentrations
The perchlorate tolerance of Chroococcidiopsis strains CCMEE 029 and CCMEE 029 P-MRS was investigated by exposure for 55 days in liquid BG-11 medium containing increasing concentration of Na-, Mg- and Ca-perchlorate up to 100 mM (Fig. 1). The presence of 5 mM of Na-, Mg- and Ca-perchlorate (5, 10 and 10 mM perchlorate ions, respectively) did not inhibit the growth of the two strains. While the presence of 50 mM perchlorate resulted in a reduced growth rate of both strains, that was more evident in Mg- and Ca-perchlorate (100 mM perchlorate ions), than in Na-perchlorate (50 mM perchlorate ions). The growth of both strains was reduced by the 100 mM concentration of Na-perchlorate, while no growth occurred in 100 mM Mg- and Ca-perchlorate (200 mM perchlorate ions) (Fig. 1).

Fig. 1. Cell densities of cultures of Chroococcidiopsis sp. CCMEE 029 and CCMEE 029 P-MRS grown in BG-11 containing 5, 50 and 100 mM Mg-, Ca- and Na-perchlorate. Control cultures were grown in BG-11. Data are shown as mean ± standard deviation in three independent trials.
Effects of increasing perchlorate concentrations on cyanobacterial morphology
The exposure to increasing concentration of Na-, Mg- and Ca-perchlorate did not show any severe morphological changes. For example, after 55 days of exposure in 100 mM Na-perchlorate, Chroococcidiopsis sp. CCMEE 029 (Fig. 2a) and CCMEE 029 P-MRS (Fig. 2b) occurred as single cells and as four-celled aggregates as typical of strain CCMEE 029 when grown in BG-11 medium (Fig. 2c).

Fig. 2. CLSM images showing the morphology of Chroococcidiopsis sp. CCMEE 029 (A) and CCMEE 029 P-MRS (B) grown for 55 days in BG-11 medium containing 100 mM NaClO4 and in control of strain CCMEE 029 (C) grown in BG-11 medium. In red autofluorescence of photosynthetic pigments. Bar scale = 10 μm.
Effects of Mars-relevant perchlorate concentration on cyanobacterial growth
The ability of Chroococcidiopsis strains CCMEE 029 and CCMEE 029 P-MRS to grow in the presence of Mars-relevant perchlorate concentrations was investigated by incubation in liquid BG-11 containing 2.4 mM ClO4− provided as a 40% Mg-perchlorate and 60% Ca-perchlorate mixture (Fig. 3). The combination of the two salts did not affect the growth rate. After 40-day incubation, strains CCMEE 029 and CMEE 029 P-MRS showed a slight increase in cell densities compared to control cells grown in BG-11 medium (Fig. 3).
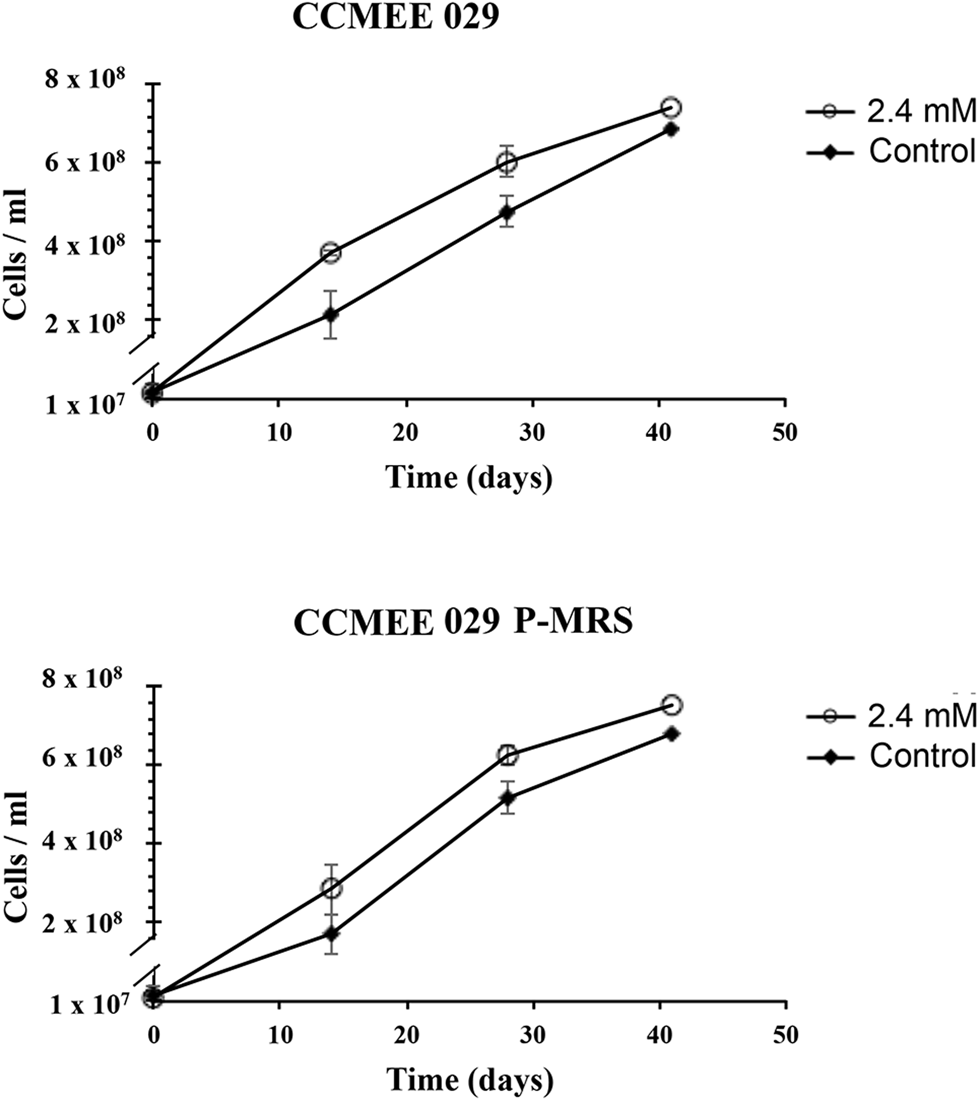
Fig. 3. Growth of Chroococcidiopsis sp. CCMEE 029 and CMEE 029 P-MRS in BG-11 and in BG-11 containing 2.4 mM perchlorate ions, provided as 40% Mg-perchlorate and 60% Ca-perchlorate mixture. Data are shown as mean ± standard deviation in three independent trials.
Perchlorate content during cyanobacterial growth
The concentration of perchlorate ions in Chroococcidiopsis strains CCMEE 029 and CCMEE 029 P-MRS was measured at the beginning and after 40-day incubation in BG-11 medium with 2.4 mM perchlorate ions. In the overall, no significant variation occurred between the perchlorate concentrations in the BG-11 medium recovered after the cyanobacterial growth and that in control BG-11 medium with 2.4 mM perchlorate ions.
Escherichia coli growth with cyanobacterial lysate-based medium
A cyanobacterial lysate-based medium was derived from the soluble fraction of lysed cells of Chroococcidiopsis sp. CCMEE 029 grown for 40 days in BG-11 with 2.4 mM perchlorate ions (Fig. 4). When about 1 × 106 E. coli cells were inoculated in 1 ml of the lysate-based medium obtained from the lysis of about 30 mg of air-dried cyanobacteria, the cell density resulted increased to about 2 × 108 cells ml−1 after overnight incubation. No significant difference occurred in E. coli cell density when using the lysate obtained from strain CCMEE 029 grown in BG-11 in the absence of 2.4 mM perchlorate ions. Escherichia coli reached an average density of 4 × 108 cells ml−1 after incubation in M9 minimal medium (containing salts, nitrogen and 0.5% glucose) and about 1 × 109 cells ml−1 in LB medium. No growth occurred when E. coli was inoculated in dd-H2O or PBS (Fig. 4).
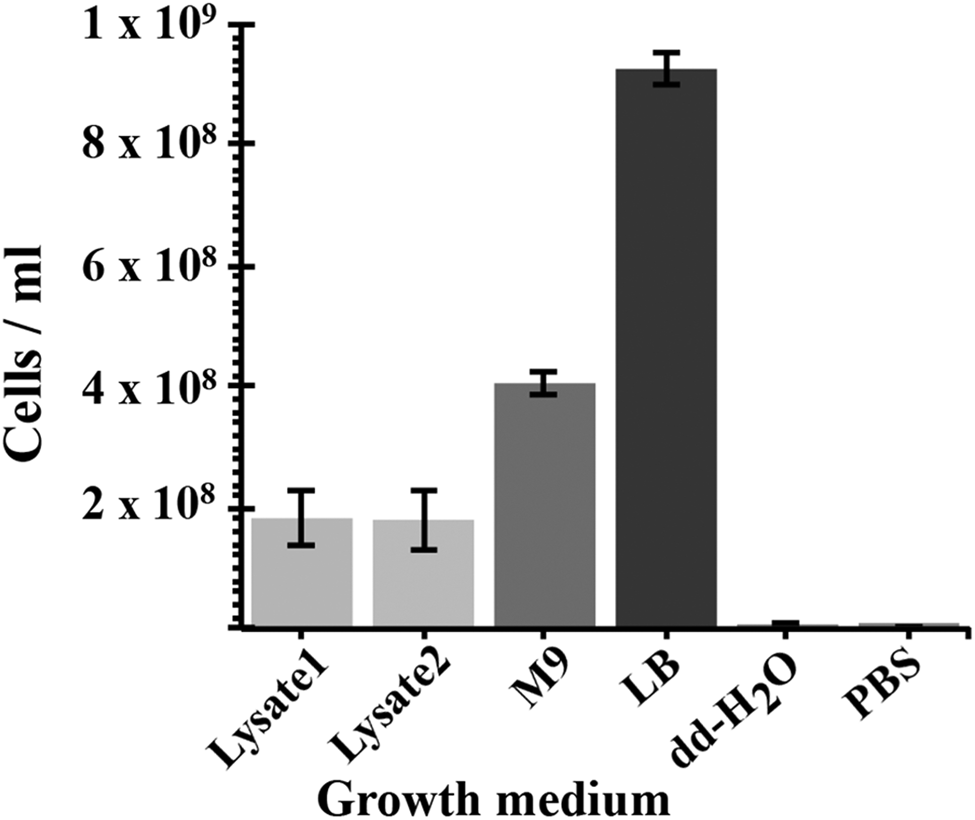
Fig. 4. Growth of E. coli supported by a cyanobacterium-based medium. Lysate 1: lysate of Chroococcidiopsis sp. CCMEE 29 grown in BG-11 containing 2.4 mM perchlorate ions; Lysate 2: lysate of Chroococcidiopsis sp. CCMEE 29 grown in BG-11. Positive controls: M9 minimal medium supplemented with 0.5% glucose and LB medium. Negative controls: dd-dH2O and PBS.
Discussion
The aim of this work was to investigate whether the desert cyanobacterium Chroococcidiopsis could be used in Bio-ISRU technologies to support the growth of BLSS components, namely bacteria, that need fixed carbon and that might be perchlorate sensitive (Rothschild, Reference Rothschild2016; Verseux et al., Reference Verseux, Baqué, Lehto, de Vera, Rothschild and Billi2016).
The incubation of Chroococcidiopsis sp. CCMEE 029 and CCMEE 029 P-MRS in increasing perchlorate concentrations identified a perchlorate threshold value at 100 mM perchlorate ions. No remarkable significant difference in the perchlorate tolerance occurred between CCMEE 029 and its space-derivate CCMEE 029 P-MRS, suggesting that the exposure to Mars simulations in low Earth orbit followed by rehydration on the ground after retrieval did not select any enhanced oxidative-stress resistance. Indeed on-going comparative genomic analysis between the genome sequence of strain CCMEE 029 and its space-derivate CCMEE 029 P-MRS will verify the eventual presence of differences between the two strains.
The identified perchlorate tolerance provided a first prerequisite for the use of Chroococcidiopsis in Bio-ISRU technologies. In contrast, at the Phoenix landing site, a concentration of about 2.4 mM perchlorate ions (0.5 wt %) was measured, whereas the Curiosity rover reported perchlorate concentrations up to 1% at the Gale Crater (Glavin et al., Reference Glavin, Archer, Brunner, Buch, Cabane, Coll, Conrad, Coscia, Dworkin, Eigenbrode, Freissinet, Mahaffy, Martin, McKay, Miller, Ming, Navarro-González, Steele, Summons, Sutter, Szopa and Teinturier2013).
Long-term (55 days) exposure of the two Chroococcidiopsis strains to increasing perchlorate concentrations revealed that growth rate was: (i) not affected in 5 mM Na-, Mg- and Ca-perchlorate (5, 10 and 100 mM perchlorate ions); (ii) slightly reduced in 50 mM Na-perchlorate; (iii) further impaired in 100 mM Na-perchlorate; (iv) reduced in 50 mM Mg- and Ca-perchlorate (100 mM perchlorate ions); and (v) completely inhibited in 100 mM Mg- and Ca-perchlorate (200 mM perchlorate ions). No growth occurred in 300 mM Na-perchlorate (not shown).
The identified 100 mM perchlorate ion threshold was comparable to that of a halophilic bacterium isolated from Big Soda Lake, growing in 2% Na-perchlorate, that is, 160 mM perchlorate ions (Matsubara et al., Reference Matsubara, Fujishima, Saltikov, Nakamura and Rothschild2017). However, Chroococcidiopsis perchlorate tolerance was lower than that of several halophiles. For example, halophilic Archaea of the family Halobacteriaceae and Halomonas elongata showed a weak growth in 600 mM Na-perchlorate (Oren et al., Reference Oren, Elevi Bardavid and Mana2014), the growth of the archaeon Halorubrum lacusprofundi was still measurable in 800 mM perchlorate ions (Laye and DasSarma, Reference Laye and DasSarma2018), while Hydrogenothermus marinus did not replicate in 400 mM Na-perchlorate (Beblo-Vranesevic et al., Reference Beblo-Vranesevic, Huber and Rettberg2017). So far the highest perchlorate tolerance was reported for the yeast Debaryomyces hansenii growing in 2.4 M Na-perchlorate (Heinz et al., Reference Heinz, Krahn and Schulze-Makuch2020), a value twice that of the bacterium Planococcus halocryophilus (Heinz et al., Reference Heinz, Waajen, Airo, Alibrandi, Schirmack and Schulze-Makuch2019).
Short-term (15 min) exposure in Na-perchlorate highlighted D10 values of 2.7, 1.3, 5 and >5 M, for Deinococcus radiodurans, E. coli, B. subtilis spores and H. marinus, respectively (Beblo-Vranesevic et al., Reference Beblo-Vranesevic, Huber and Rettberg2017). In the present work, Chroococcidiopsis strains were not exposed to short-term incubation in higher perchlorate concentrations because the main goal was to identify the tolerance threshold in long-term cultivation.
No significant morphological changes were observed in Chroococcidiopsis exposed up to 200 mM perchlorate ions. On the contrary, halophilic archaea occurred as swollen cells in perchlorate ion concentrations >200 mM (Oren et al., Reference Oren, Elevi Bardavid and Mana2014), H. marinus turned into long cell chains in Na-perchlorate concentrations >100 mM (Beblo-Vranesevic et al., Reference Beblo-Vranesevic, Huber and Rettberg2017), while P. halocryophilus formed large cell clusters in 1.1 M Na-perchlorate (Heinz et al., Reference Heinz, Waajen, Airo, Alibrandi, Schirmack and Schulze-Makuch2019).
The two Chroococcidiopsis strains better tolerated Na- and Mg-perchlorate than Ca-perchlorate. Indeed Ca-perchlorate is a chaotropic agent, causing macromolecules destabilization and growth inhibition stronger than Mg-perchlorate and Na-perchlorate, the latter being a weak chaotropic agent (Cray et al., Reference Cray, Russell, Timson, Singhal and Hallsworth2013; Nagler and Moeller, Reference Nagler and Moeller2015). Moreover, since Chroococcidiopsis sp. CCMEE 029 has been reported to bleach in 680 mM NaCl (Hershkovitz et al., Reference Hershkovitz, Oren and Cohen1991), the observed slightly reduced growth in 100 mM Na-perchlorate might be ascribed to the oxidizing action of perchlorate.
Since 2.4 mM perchlorate ions were reported to occur on Mars as a 60 and 40% mixture of Mg- and Ca-perchlorate, respectively (Hassler et al., Reference Hassler, Zeitlin, Wimmer-Schweingruber, Ehresmann, Rafkin, Eigenbrode, Brinza, Weigle, Böttcher, Böhm, Burmeister, Guo, Köhler, Martin, Reitz, Cucinotta, Kim, Grinspoon, Bullock, Posner, Gómez-Elvira, Vasavada and Grotzinger2014), the two Chroococcidiopsis strains were grown in Mars-relevant perchlorate ions as reported for the Phoenix landing site. The growth of the two Chroococcidiopsis strains was not impaired by 40-day incubations in 2.4 mM perchlorate ions, rather it increased slightly compared to control. This might be due to a lack of a synergistic negative effect of Mg- and Ca-perchlorate as reported for bacteria isolated from Big Soda Lake (Matsubara et al., Reference Matsubara, Fujishima, Saltikov, Nakamura and Rothschild2017), but also to a positive effect of the increased cation concentration. Indeed Anabaena sp. PCC 7120 was reported to grow in 1 mM CaCl2 (Singh et al., Reference Singh, Verma, Niveshika Tiwari and Mishra2016). Here Chroococcidiopsis cells were grown in concentrations of 0.24 mM CaCl2 and 0.30 mM MgSO4 supplied by the BG-11 medium (Rippka et al., Reference Rippka, Deruelles, Waterbury, Herdman and Stanier1979) while 0.5 mM Mg2+ and 0.75 mM Ca2+ were present in 2.4 mM perchlorate ions supplied as a 60 and 40% mixture of Mg- and Ca-perchlorate, respectively.
After 40-day growth in 2.4 mM perchlorate ions, no variation in the perchlorate content was observed; therefore, the perchlorate concentration in the lysate was not determined. Indeed cyanobacteria are non-perchlorate-reducing prokaryotes, although the presence of chlorite dismutase-like proteins has been reported in a few nitrogen-fixing species (Nerenberg, Reference Nerenberg2013; Schaffner et al., Reference Schaffner, Hofbauer, Krutzler, Pirker, Furtmüller and Obinger2015). Hence, the perchlorate tolerance of the two Chroococcidiopsis strains was likely a consequence of their efficient antioxidant system (Fagliarone et al., Reference Fagliarone, Mosca, Ubaldi, Verseux, Baqué, Wilmotte and Billi2017).
The observed Chroococcidiopsis capability to tolerate Mars-relevant perchlorate ions was further exploited to obtain a lysate-based medium to feed an E. coli strain, capable of utilizing sucrose as a carbon source (Lee and Chang, Reference Lee and Chang1993). Therefore, after 40-day growth in 2.4 mM perchlorate, Chroococcidiopsis cells were air-dried to induce a fivefold increase in their sucrose content from, up to 5 mg/g dry weight (not shown), as previously reported (Fagliarone et al., Reference Fagliarone, Napoli, Chiavarini, Baqué, de Vera and Billi2020). The lysate-based medium obtained from 10 mg of dried cyanobacteria supported an increase of the E. coli cell density from 1 × 106 to 2 × 108 cells after overnight incubation. A comparable increase of E. coli cell density was obtained using the lysate obtained from Chroococcidiopsis grown in standard BG-11 medium. This suggested that washing the cyanobacterial cells before lysis removed perchlorate and avoided a negative effect on E. coli growth. However, since a doubled cell density (about 4 × 108 cells) was supported by overnight growth in M9 minimal medium, an increased E. coli cell density is expected to be supported by lysing an increased amount of dried cyanobacteria.
However, results provided a first proof-of-concept of feeding a bacterium with a lysate-based medium obtained from Chroococcidiopsis grown in Mars-relevant perchlorate concentration. The use of this cyanobacterium as a pioneer for Bio-ISRU on Mars is further supported by its capability of lithotrophic growth on Mars regolith analogues, namely anorthosite and basalt (supplemented with NaNO2), resulting in elemental release and biomass production (Olsson-Francis and Cockell, Reference Olsson-Francis and Cockell2010).
Moreover, the feeding of E. coli with a cyanobacterial lysate is relevant since this bacterium is a typical chassis for genetic manipulation and metabolic engineering of bacteria was proposed to provide consumables to human outposts on Mars (Rothschild, Reference Rothschild2016). Recently, the use of cyanobacterial lysate to support B. subtilis engineered to synthetize aromatic polymers for space application has been reported (Averesch and Rothschild, Reference Averesch and Rothschild2019) and tested in NASA's PowerCell payload aboard the DLR EuCROPIS Satellite (McCutcheon et al., Reference McCutcheon, Kent, Paulino-Lima, Pless, Ricco, Mazmanian, White, Tapio and Rothschild2015).
In conclusion, Chroococcidiopsis might enable Bio-ISRU on Mars by combining its capability of converting CO2 to organic compounds with its perchlorate resistance, and its use in Bio-ISRU might be further reinforced by synthetic biology (Montague et al., Reference Montague, McArthur, Cockell, Held, Marshall, Sherman, Wang, Nicholson, Tarjan and Cumbers2012; Snyder et al., Reference Snyder, Walsh, Carr and Rothschild2019). Indeed, the development of Chroococcidiopsis CCMEE 029 as a chassis for synthetic biology will endow it with the ability to synthetize compounds not naturally produced and this approach will take advantage of the availability of a genetic system for its genetic manipulation (Billi et al., Reference Billi, Friedmann, Helm and Potts2001) and maintenance of plasmid DNA after prolonged air-dried storage (Billi, Reference Billi2012).
Acknowledgements
This research was supported by the Italian Space Agency (ReBUS – In-situ Resource Bio-Utilization per il Supporto alla Vita nello Spazio, grant n° 2019-4-U.0 WP: Cyanobacteria for ISRU, to D.B). We acknowledge Dr Elena Romano, Centre of Advanced Microscopy ‘Patrizia B. Albertano’, University of Rome Tor Vergata, for her skilful assistance in using the confocal laser scanning microscope.