1. Introduction
The Mistaken Point Ecological Reserve (MPER), on the southern shore of the Avalon Peninsula, Newfoundland, Canada (Fig. 1), is the site of some of the oldest known complex body fossils (e.g. Narbonne & Gehling, Reference Narbonne and Gehling2003). Owing to the global importance of the fossils, the MPER was declared a UNESCO World Heritage Site in 2016. The fossils of the Mistaken Point biotas lived in deep-water marine palaeoenvironments of Avalonia and form part of the Avalon Assemblage (Waggoner, Reference Waggoner2003), which is reported from Newfoundland, Canada, and in the United Kingdom (Brasier & Antcliffe, Reference Brasier and Antcliffe2004; Narbonne, Reference Narbonne2005), with similar biotas being reported from Siberia and western Canada (Grazdhankin et al. Reference Grazhdankin, Balthasar, Nagovitsin and Kochnev2008; Narbonne et al. Reference Narbonne, Laflamme, Trusler, Dalrymple and Greentree2014).
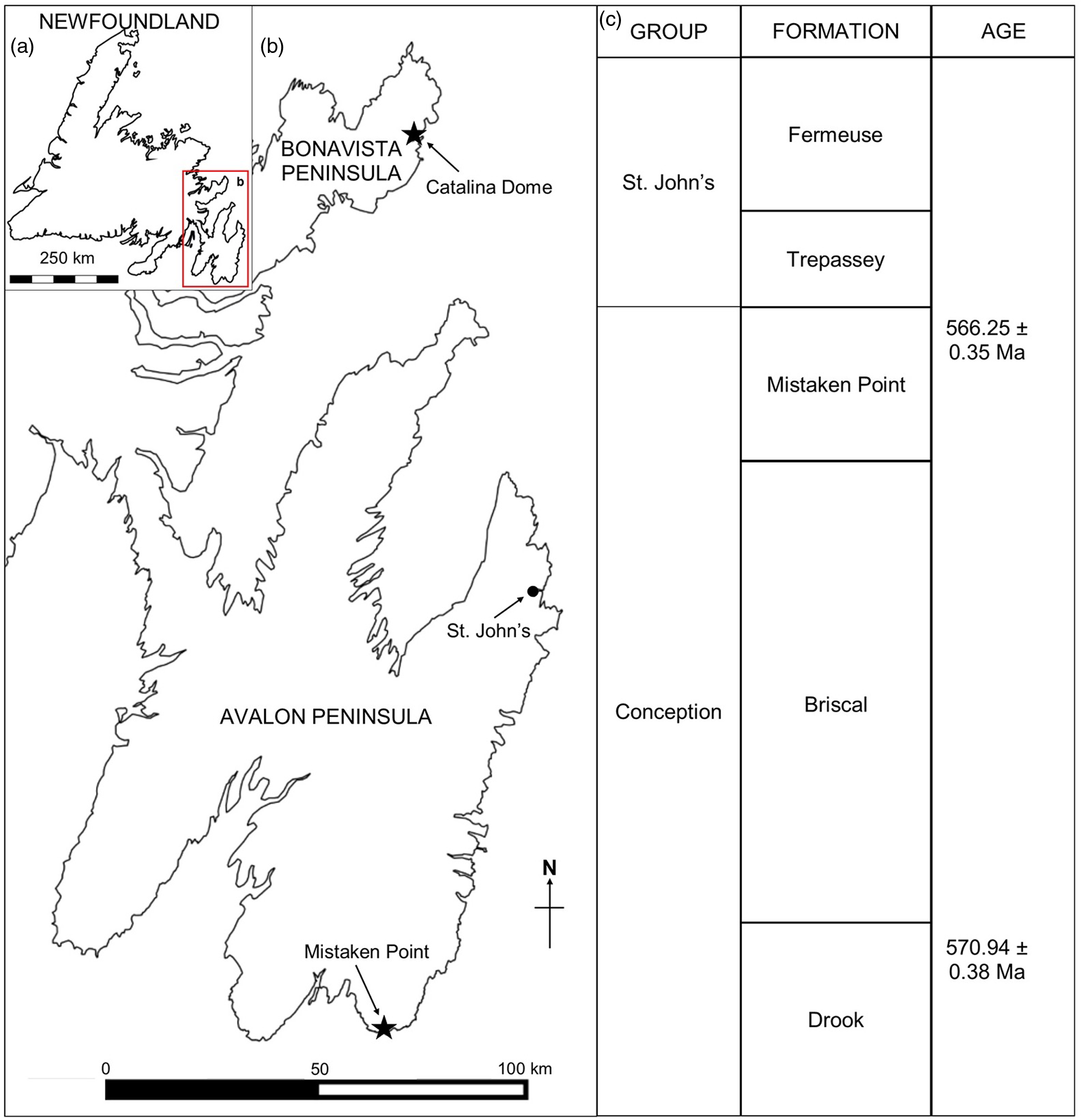
Fig. 1. (a) Map of Newfoundland, Canada. (b) Ediacaran localities at Mistaken Point (Avalon Peninsula) and Little Catalina (Bonavista Peninsula). (c) Ediacaran stratigraphy in Eastern Newfoundland (following O’Brien & King, Reference O’Brien and King2005 and Matthews et al. Reference Matthews, Liu, Yang, McIlroy, Levell and Condon2021).
Several Ediacaran fossiliferous localities have been discovered on the southeastern portion of the Avalon Peninsula in Newfoundland (see Liu et al. Reference Liu, Kenchington and Mitchell2015). The oldest known fossils in the region occur close to Pigeon Cove in the MPER (Narbonne & Gehling, Reference Narbonne and Gehling2003; Liu et al. Reference Liu, McIlroy, Antcliffe and Brasier2011, Reference Liu, McIlroy, Matthews and Brasier2012) that have recently been re-dated at 576.17 ± 0.66 Ma, based on geochronological study of the tuffite immediately overlying the fossils (Matthews et al. Reference Matthews, Liu, Yang, McIlroy, Levell and Condon2021; updated from Pu et al. Reference Pu, Bowring, Ramezani, Myrow, Raub, Landing, Mills, Hodgin and Macdonald2016). The best-known fossiliferous locality in the area is at Mistaken Point itself, where two of the most extensive bedding planes, the D and E surfaces, are exposed (Anderson & Misra, Reference Anderson and Misra1968; Misra, Reference Misra1969). The tuff immediately overlying the Fractofusus-rich E Surface has also been re-dated to 565.00 ± 0.64 Ma (Matthews et al. Reference Matthews, Liu, Yang, McIlroy, Levell and Condon2021).
The Ediacaran rangeomorph Fractofusus was illustrated several years before its full taxonomic description, being referred to as ‘spindles’ (e.g. Misra, Reference Misra1969; Anderson & Conway Morris, Reference Anderson and Conway Morris1982; Jenkins, Reference Jenkins, Lipps and Signor1992); Seilacher (Reference Seilacher1999, fig. 3) illustrated a specimen of Fractofusus considerably before its description, referring to it as ‘Vendofusus’. Fractofusus is represented in Newfoundland by two species: F. misrai and F. andersoni (Gehling & Narbonne, Reference Gehling and Narbonne2007). Fractofusus misrai (Fig. 2a) is the most common fossil on the D and E surfaces at Mistaken Point (Clapham et al. Reference Clapham, Narbonne and Gehling2003), accounting for 1070 (76.3 %) and 1140 (38.4 %) specimens on the two surfaces, respectively (Mitchell et al. Reference Mitchell, Kenchington, Liu, Matthews and Butterfield2015). Fractofusus andersoni is the most abundant member of the genus in the Catalina Dome, Bonavista Peninsula (Hofmann et al. Reference Hofmann, O’Brien and King2008; Figs 1, 2b), but F. andersoni also occurs in the MPER (Gehling & Narbonne, Reference Gehling and Narbonne2007). A single Fractofusus specimen has been reported by Narbonne et al. (Reference Narbonne, Laflamme, Trusler, Dalrymple and Greentree2014) from Ediacaran deposits in NW Canada, but preservational details are insufficient to allow for a definitive identification. Fractofusus might thus be endemic to Newfoundland, not even being reported in the contemporaneous and palaeogeographically adjacent Avalonian sections in the UK (e.g. Wilby et al. Reference Wilby, Carney and Howe2011; Kenchington et al. Reference Kenchington, Harris, Vixseboxse, Pickup and Wilby2018 b).

Fig. 2. (a) Fractofusus misrai. Scale bar = 20 mm. (b) Fractofusus andersoni. Scale bar = 10 mm. (c) Curved F. misrai. Scale bar = 30 mm. (d) Kinked F. misrai. Scale bar = 10 mm. (a), (c) and (d) from E Surface, Mistaken Point; (b) from Hofmann 14, Port Union, Bonavista Peninsula (field photographs).
Fractofusus has usually been described as ‘spindle-like’ or ‘spindle-shaped’ in appearance (e.g. Misra, Reference Misra1969; Hofmann, Reference Hofmann1987; Landing et al. Reference Landing, Narbonne, Myrow, Benus and Anderson1988), owing to its similarity to the fusiform wooden shuttle/spindle of the early mechanical looms. While idiomorphic specimens of F. misrai are fusiform, there are a range of morpho/taphotypes, whereas F. andersoni is uniformly ovoid in outline (Gehling & Narbonne, Reference Gehling and Narbonne2007). In this manuscript we focus on atypical F. misrai that deviate from the fusiform morphotype, including curved and kinked forms (Fig. 2c, d) and otherwise disturbed/tousled specimens, to explore aspects of its branch morphology, biostratinomy and palaeobiology.
2. Geological setting
Outcrops of the Neoproterozoic Conception and St John’s groups are found throughout southeastern Newfoundland, most notably at Mistaken Point and in the Catalina Dome (see Fig. 1a, b). The sedimentologic and stratigraphic history of the Mistaken Point region has been described in detail (King & Williams, Reference King and Williams1979; Benus, Reference Benus, Landing, Narbonne and Myrow1988; Wood et al. Reference Wood, Dalrymple, Narbonne, Gehling and Clapham2003; Ichaso et al. Reference Ichaso, Dalrymple and Narbonne2007) (Fig. 1c). Ediacaran fossiliferous surfaces at both Mistaken Point and the Bonavista Peninsula lay in deep-water marine palaeoenvironments preserved as obrution deposits with the smothering event being tuffite deposition (Benus, Reference Benus, Landing, Narbonne and Myrow1988; Seilacher, Reference Seilacher1992; Wood et al. Reference Wood, Dalrymple, Narbonne, Gehling and Clapham2003; O’Brien & King, Reference O’Brien and King2005; Hofmann et al. Reference Hofmann, O’Brien and King2008; Matthews et al. Reference Matthews, Liu, Yang, McIlroy, Levell and Condon2021). On the Avalon Peninsula F. misrai is reported from the Briscal and Mistaken Point formations (Matthews et al. Reference Matthews, Liu, Yang, McIlroy, Levell and Condon2021), whereas on the Bonavista Peninsula, Fractofusus has been reported from the Mistaken Point and Trepassey formations (O’Brien & King, Reference O’Brien and King2004, Reference O’Brien and King2005; Hofmann et al. Reference Hofmann, O’Brien and King2008).
3. Material
Fractofusus misrai is well known from several horizons in the MPER, most notably the D and E surfaces at Mistaken Point. Two specimens of F. misrai have also been reported from the Bonavista Peninsula (Hofmann et al. Reference Hofmann, O’Brien and King2008), casts of which were studied in The Rooms in St John’s, Newfoundland. We consider that the first of these (Hofmann et al. Reference Hofmann, O’Brien and King2008, fig. 20.1; supplemental fig. 1c, available at http://journals.cambridge.org/geo) is F. andersoni, and the second (Hofmann et al. Reference Hofmann, O’Brien and King2008, fig. 20.4) is too poorly preserved to identify with certainty.
This study focuses on F. misrai from the E Surface at Mistaken Point. Since it is illegal to collect fossils within the MPER, all specimens were observed and photographed in situ in the field. Silicone casts were produced where possible, from which replicas were produced for laboratory study and photography. Casts of Fractofusus from 2005 from the D and E surfaces of Mistaken Point (collection of Prof. Martin Brasier) were examined at the Oxford University Museum of Natural History.
Morphometric and orientation data were collected from 151 specimens of F. misrai from the Mistaken Point E Surface, based on field photographs and specimen casts of complete or nearly complete specimens. Metrics measured included number of first-order branches in both branch rows of straight specimens (online Supplementary Material Table S1); maximum length and width of straight and kinked/curved specimens (online Supplementary Material Tables S2, S3); number of first-order branches in both branch rows of kinked/curved specimens (online Supplementary Material Table S4); and location and angle of kink or curve in kinked/curved specimens (online Supplementary Material Table S5). Sampling was based on specimen completeness and preservation quality: data are only presented for specimens for which accurate data could be collected. Variations in first-order branch size and shape associated with the position of the kink or curve were examined in suitable specimens. Data were collected only from curved/kinked specimens that could be accurately measured; specimens were not included in these analyses if distal tip/branch morphology could not accurately be assessed or if primary branches could not be accurately counted. In the case of specimens showing unusual morphological features, morphometric data could not always be collected. Data were also collected from a sub-sample of well-preserved straight specimens from which accurate size/branch number data could be collected, for use as a comparator group.
4. Results
The detailed morphology of Fractofusus misrai is described in the Systematic Palaeontology section (see Section 5.i below).
4.a. Fidelity of Fractofusus preservation
Fractofusus specimens range from high-fidelity preservation – in which three orders of branches are typically preserved (Gehling & Narbonne, Reference Gehling and Narbonne2007; Brasier & Antcliffe, Reference Brasier and Antcliffe2009) – to effectively effaced smooth fusiform fossils that could be considered to be on the taphonomic pathway to becoming ivesheadiomorphs (Liu et al. Reference Liu, McIlroy, Antcliffe and Brasier2011; Antcliffe et al. Reference Antcliffe, Hancy and Brasier2015). The importance of the inferred grades of preservation is that they show a continuum from well-preserved forms that represent the lower surface of Fractofusus smothered in life by the overlying tuffite to poorly preserved outlines that may represent ivesheadiomorphs constituting necromass on the pre-tuffite seafloor (Liu et al. Reference Liu, McIlroy, Antcliffe and Brasier2011; Antcliffe et al. Reference Antcliffe, Hancy and Brasier2015). In most specimens, branching close to the longitudinal axis is well preserved and relatively high relief; however, at the distal tips of the frond and its lateral margins the first-order branches may show low-relief preservation (e.g. Fig. 3a, c).

Fig. 3. (a) Disruption of glide plane symmetry across the longitudinal midline of a Fractofusus misrai growing in close proximity to an ivesheadiomorph (cast photograph). Scale bar = 20 mm. (b) Folded-over specimen (cast photograph). Scale bar = 10 mm. (c) Magnification of F. misrai specimen shown in (a), highlighting the decrease in preservation detail from the medial region towards the distal tip of the specimen (field photograph). Scale bar = 5 mm. (d) Difference in length–width proportions on either side of the kink in a strongly kinked specimen (cast photograph). Scale bar = 20 mm.
4.b. Characteristics of the commissure and symmetry of Fractofusus
A near-straight midline is most often produced in Fractofusus when first-order branches either side of the central axis are opposite and of approximately the same size (Fig. 2a), whereas a zig-zag midline is produced when opposing first-order branches are arranged with glide plane symmetry (Brasier et al. Reference Brasier, Antcliffe and Liu2012; Fig. 3d). The asymmetrical arrangement of first-order branches in straight specimens usually results from the presence of one or more anomalously sized branches (Fig. 3a) or by having different numbers of first-order branches in each row (contra Anderson & Conway Morris, Reference Anderson and Conway Morris1982). Of the 39 straight specimens for which first-order branches could accurately be counted, only 13 possessed the same number of first-order branches in both rows (e.g. Fig. 3a; online Supplementary Material Table S1). The amplitude and wavelength of the zig-zagged commissure typically decreases towards the distal tips of F. misrai (Fig. 2c). Specimens with kinks are more likely to be longer (mean: 11.4 cm; range: 3.2–28.6 cm) and wider (mean: 3.7 cm; range: 1.6–9.8 cm) than straight specimens (length: mean: 9.8 cm; range: 5.4–20.3 cm; width: mean: 2.8 cm; range: 1.2–5.4 cm); a Welch two-samples t-test shows this difference in length and width to be significant, t(101) = 3.7534, p < 0.05 (Fig. 4; online Supplementary Material Tables S2, S3).

Fig. 4. Width versus length/width ratio of Fractofusus misrai specimens (blue = kinked specimens; green = straight specimens). Kinked specimens: R2 = 0.09; p-value = 0.0038. Straight specimens: R2 = 0.36; p-value = 0.075.
The number of first-order branches per row among the assemblage of F. misrai from Mistaken Point ranges from 8 to greater than 30 (the exact maximum number is unknown owing to distal tips not being preserved in specimens with the highest branch numbers). First-order branch size/shape is sometimes highly variable within a specimen (e.g. Fig. 5c), and may be related to changes in the proportional size of the second-order rangeomorph branches making up each first-order branch. The architecture of the second-order branches is consistent between adjacent first-order branches regardless of their shape. Inconsistencies in first-order branch shape likely reflect a combination of true biological variability, the vagaries of both antemortem current activity and postmortem biostratinomy/taphonomy.

Fig. 5. (a) Straight edge across several first-order branches (highlighted by arrow) plus growth of new branches in gap (details of new growth coloured orange in inset) (cast photograph). Scale bar = 20 mm (inset, 10 mm). (b) Curved and scalloped outer margin on first-order branch with clearest first-order branch highlighted with arrow (in print) and in orange (online) (cast photograph). Scale bar = 10 mm. (c) Branches of multiple lengths in one row (field photograph). Scale bar = 10 mm. (d) Variability in first-order branch size/shape (cast photograph). Scale bar = 20 mm.
Most specimens of F. misrai have the first-order branches closely adpressed against the adjacent branches at their lateral margins (their presumed life orientation). Some specimens, however, possess branches that are in disarray in relation to each other laterally, showing a range of orientations of the rangeomorph branches, and uneven distal margins (e.g. Fig. 6c). The orientations of the first-order branches in these specimens appear to have been lifted from the sediment surface and resettled on the seafloor in a disordered or ‘tousled’ manner (sensu Brasier et al. Reference Brasier, Antcliffe and Liu2012). This process of tousling provides unique insights into the morphology of parts of branches that are otherwise not in contact with the seafloor.

Fig. 6. (a) Pair of overlapping F. misrai (cast photograph). Scale bar = 20 mm. (b) Magnification of disc-like structure highlighted in Figure 6a (cast photograph). Scale bar = 10 mm. (c) Disturbed first-order branch alignment in tousled specimen (cast photograph). Scale bar = 35 mm. (d) Third-order branch tips, with undetermined circular structure (arrow; field photograph). Scale bar = 15 mm. (e) Third-order branch tips in Figure 7d with clarified branching details (field photograph). Scale bar = 15 mm.
4.c. Kinked and curved Fractofusus
While most F. misrai have an elongate fusiform outline, some specimens have a central axis that is curved, sinusoidal (Fig. 2c) or kinked. Curved or kinked Fractofusus may be of any size including individuals as small as 4.5 cm in length; therefore such forms probably result from intrinsic growth rather than external forces such as seafloor currents, which should preferentially impact large specimens owing to their greater aspect ratio.
Several specimens of F. misrai with curves or kinks have uneven numbers of first-order branches per row with the extra first-order branch being on the convex side of the curve/kink (Fig. 2d; online Supplementary Material Table S4). It is noted however that straight Fractofusus can possess uneven numbers of first-order branches across the midline, in which case some first-order branches are notably wider than others (Fig. 5d). Among a sample of 75 curved or kinked specimens for which the number of first-order branches could be determined in both rows, 49 specimens had unequal numbers of first-order branches in opposing rows. Of those 49 specimens, 44 had one extra branch, 5 had two extra branches and 3 specimens had three or more additional first-order branches in the convex branch row. This is taken as strong evidence that curvature/kinking is related to or causes additional branch nodes along the axis.
4.d. Damaged and regenerated Fractofusus
Rare F. misrai specimens show evidence of damage in the form of an interruption in the outline of the fossil, more often on the convex side of a localized kink, which may be related to mechanical tearing of the organism. In most specimens this damage occurs along the contact between adjacent first-order branches (Fig. 7b, c), implying that the first-order branches were normally isolated from each other and thus functioned as discrete mechanical (and possibly metabolic) entities in contrast to the highly constrained margins of the Charnida (e.g. Beothukis, Charnia and Trepassia; McIlroy et al. Reference McIlroy, Hawco, McKean, Nicholls, Pasinetti and Taylor2020). The gap region in one kinked specimen reveals small clusters of radiating branching elements (<1 cm in length) that might represent regenerative growth (Fig. 5a; specimen 193); such damage repair is rarely observed and is most likely only seen in the case of damage produced shortly before smothering/preservation.

Fig. 7. (a) Overlap of first-order branches on concave side of kink. Scale bar = 10 mm. (b) Pair of gaps in margin between first-order branches (highlighted by arrows). Scale bar = 20 mm. (c) Single gap in margin between first-order branches (highlighted by arrow). Scale bar = 20 mm. (d) Disruption in outer margin (highlighted by arrow). Scale bar = 20 mm (cast photographs).
4.e. Folded Fractofusus
In the original description of F. misrai, Gehling & Narbonne (Reference Gehling and Narbonne2007) suggested that some specimens were partially folded over with ‘no distinguishing ventral-dorsal characteristics’ visible, the upper and lower surfaces being allegedly identical (p. 375). An unexpected feature of purportedly folded F. misrai is a lack of evidence for the original position of the frond. If an epifaunal organism was folded over – particularly one that is quasi-infaunal (Dufour & McIlroy, Reference Dufour, McIlroy, Brasier, McIlroy and McLoughlin2017 a) – a ghost of the original position of the folded portion of the frond should intuitively be visible on the adjacent bedding plane (cf. McIlroy et al. Reference McIlroy, Brasier and Lang2009) if it were to be disrupted by turbidity currents during the smothering event (cf. Seilacher, Reference Seilacher1999). Such ghost outlines are not visible adjacent to any ‘folded’ Fractofusus specimens (Fig. 3b, d, online Supplementary Material Fig. S1a) requiring an alternative taphonomic model for ‘folded’ forms. Though we do note that ghost outlines of individual first-order branches of Fractofusus are known from ‘tousled’ specimens (sensu Brasier et al. Reference Brasier, Antcliffe and Liu2012; Fig. 6c). The location of the fulcrum in folded (and kinked) specimens of F. misrai is variable (Figs 2d, 3b, 5a, 7a, d) but is not observed close to the distal ends of the F. misrai frond (online Supplementary Material Table S5).
4.f. Fractofusus modes of reproduction
Several F. misrai observed during this study possess small (≤1 cm), discrete smooth circular to ovoid structures associated with some well-preserved first-order branches (Fig. 6a, b, d, e). We note that the circular structures are most often situated near the midline on approximately the fifth or sixth first-order unit from the distal end of the body. One of the circular structures close to the medial axis of a large specimen of F. misrai has a concentric ring within it (Fig. 6a, b). These atypical structures may represent evidence of reproductive structures; however, no clear evidence of propagules or physical connections between potential ‘parent–offspring’ pairs has been observed.
5. Discussion
Based on the observations presented in Section 4, we have developed a new reconstruction of Fractofusus misrai which considers its morphology, palaeobiology, palaeoecology and taphonomy. This model encompasses many of the unusual characteristics observed in this taxon, such as kinked or curved forms, variability in preservation quality between/within specimens, and individuals that appear to be folded over themselves.
5.a. Ecology of Fractofusus misrai
The assemblage of Fractofusus on the D and E surfaces has been determined by several authors to be randomly orientated (Seilacher, Reference Seilacher1992; Gehling & Narbonne, Reference Gehling and Narbonne2007; Antcliffe et al. Reference Antcliffe, Hancy and Brasier2015; Mitchell et al. Reference Mitchell, Kenchington, Liu, Matthews and Butterfield2015), which contrasts with the possibly rheotropically orientated Charniodiscus procerus of ‘Seilacher’s Corner’ (McIlroy et al., Reference McIlroy, Pérez-Pinedo, Pasinetti, McKean, Taylor and Hiscott2022; Fig. 8). The lack of current orientation is the basis for inferring a reclining mode of life for Fractofusus (Seilacher, Reference Seilacher1992; Gehling & Narbonne, Reference Gehling and Narbonne2007). More specifically, Fractofusus can be inferred to have had a quasi-infaunal sediment displacing mode of life, in which they both smothered and grew slightly down into the matground dominated seafloor below the ambient bedding plane, as can be seen in wave eroded specimens without an underlying microbial matground (Dufour & McIlroy, Reference Dufour, McIlroy, Brasier, McIlroy and McLoughlin2017 a). Fractofusus is one of the few fossil taxa that commonly overlies other fossils, particularly ivesheadiomorphs (Liu et al. Reference Liu, McIlroy, Antcliffe and Brasier2011; Dufour & McIlroy, Reference Dufour, McIlroy, Brasier, McIlroy and McLoughlin2017 a). The association with inferred necromass is consistent with the suggestion that Fractofusus was tolerant of sulfidic porewaters and may have had sulfur oxidizing ectosymbionts (Dufour & McIlroy, Reference Dufour, McIlroy, Brasier, McIlroy and McLoughlin2017 a,b; McIlroy et al. Reference McIlroy, Dufour, Taylor and Nicholls2021). Claims that chemosymbiosis is not a valid mode of life for the Ediacaran biota based on macrofossil spatial distribution data (Flude & Narbonne, Reference Flude and Narbonne2008) appear to be based on the consideration that chemosynthetic microbial communities are commonly related to localized seafloor seeps (cf. Georgieva et al. Reference Georgieva, Little, Bailey, Ball and Glover2018). However, organisms with chemosymbionts are relatively common components of seafloor communities in which the symbionts exploit the biogeochemistry of sulfidic porewaters (e.g. Dubilier et al. Reference Dubilier, Bergin and Lott2008; Zanzerl & Dufour, Reference Zanzerl and Dufour2017). The concept of Ediacaran organisms utilizing symbiotic relationships for feeding was previously discussed by Seilacher (Reference Seilacher1999), who suggested that Ediacaran ‘mat encrusters’ were permanently attached to the microbial mat on the seafloor and fed through either filtration or photosymbiosis. The argument that the earliest pre-sponges living on soft substrates might have had basipinacocytes (Dufour & McIlroy, Reference Dufour and McIlroy2017 b) was used by Cavalier-Smith (Reference Cavalier-Smith2018) to suggest that Fractofusus might have been a pre-sponge, with the ventral surface providing a barrier to sulfide build-up (see also Dufour & McIlroy, Reference Dufour, McIlroy, Brasier, McIlroy and McLoughlin2017 a), rather than an organism with a grade of organization close to the Placozoa (Dufour & McIlroy, Reference Dufour, McIlroy, Brasier, McIlroy and McLoughlin2017 a). We follow the latter authors in considering that the fractal-like lower surface is much more likely to have been for the culturing of chemosymbionts than simply as a permeability barrier.

Fig. 8. Aerial view of the NW portion of Mistaken Point’s E Surface, including specimens of Fractofusus misrai (F), Beothukis mistakensis (B), Bradgatia linfordensis (Bl), Charniodiscus procerus (C), Charniodiscus spinosus (Cs), Thectardis avalonensis (T) and ivesheadiomorphs (I); ‘Seilacher’s Corner’ (and its current-orientated Charniodiscus procerus) is situated in the lower right corner (scale bar = 10 cm).
Chemosynthetic phagotrophy is a likely mode of life for several non-motile reclining Ediacaran organisms such as Fractofusus, Beothukis, Gigarimaneta and possibly Charnia (Dufour & McIlroy, Reference Dufour, McIlroy, Brasier, McIlroy and McLoughlin2017 a; Taylor et al. Reference Taylor, Matthews, Nicholls and McIlroy2021; McIlroy et al. Reference McIlroy, Hawco, McKean, Nicholls, Pasinetti and Taylor2020, Reference McIlroy, Dufour, Taylor and Nicholls2021). Dufour & McIlroy (Reference Dufour, McIlroy, Brasier, McIlroy and McLoughlin2017 a) considered several possible alternative models for feeding among epifaunal Ediacaran organisms, including dissolved organic matter, osmotrophy and filter feeding, and demonstrated how each of these models would have been insufficient to provide these epifaunal organisms with adequate nutrition and/or protection from environmental sulfides. We utilize this chemosynthetic phagotrophy model as it is currently the most viable model for epifaunal organisms in the Ediacaran.
The high-fidelity preservation of Fractofusus may have been enhanced by factors other than its epifaunal lifestyle. It has been suggested that Fractofusus may have used ciliary pumping to move oxygenated water between the lower surface of the organism and the underlying sediment. Such an adaptation is necessary to reduce hydrogen sulfide accumulation that would otherwise be generated by anaerobic, sulfate-reducing bacteria and would otherwise cause cell death if not oxidized beyond the epithelium (Dufour & McIlroy, Reference Dufour, McIlroy, Brasier, McIlroy and McLoughlin2017 a,b). Bioirrigation would have encouraged the growth of chemolithoautotrophic bacteria below the organism, potentially providing an important source of nutrition through chemosymbiosis and/or phagotrophy (Dufour & McIlroy, Reference Dufour, McIlroy, Brasier, McIlroy and McLoughlin2017 a,b). A by-product of this simple ecosystem engineering may have been a physical and/or chemical binding of the sediment immediately below these chemolithoautotrophic bacteria, resulting in improved preservation of the lower surface of Fractofusus. It has been suggested that the presence of sulfate-reducing bacteria is required for Conception-style preservation (Narbonne, Reference Narbonne2005), in a manner comparable to the ‘death mask’ model for soft-bodied preservation (Gehling, Reference Gehling1999; Mapstone & McIlroy, Reference Mapstone and McIlroy2006) and supported by the presence of pyrite on fossiliferous surfaces (Liu, Reference Liu2016).
5.b. Morphological irregularities in Fractofusus misrai
5.b.1. Kinked/curved Fractofusus misrai
The occurrence of numerous kinked or curved specimens of F. misrai indicated that the tissues of the axis to which all first-order branches were presumably anchored must have possessed some degree of flexibility. The conventional interpretation of kinked or curved specimens of F. misrai is that they were moved by antemortem currents (Seilacher, Reference Seilacher1992), but these irregularities in the axis of Fractofusus may also be due to changes in growth direction in response to environmental gradients (e.g. chemotropism, thigmotropism or rheotropism). It is considered that, during ontogeny, Fractofusus had the potential to both add first-order branches and increase the size of those same units by inflation (Brasier et al. Reference Brasier, Antcliffe and Liu2012). It is likely to have been relatively simple for Fractofusus to grow in response to a variety of seafloor stimuli by adding additional units to a row of first-order branches to effect curvature towards the opposite side, or similarly by preferential inflation of first-order branches on one side of the organism.
In some strongly kinked specimens of F. misrai, there is a notable difference in length:width and outline of the first-order units on either side of the kink (Fig. 3d; online Supplementary Material Fig. S1a), which is exacerbated by tectonic deformation in some cases (Seilacher, Reference Seilacher1999; Wood et al. Reference Wood, Dalrymple, Narbonne, Gehling and Clapham2003). Retrodeformation of kinked specimens, however, results in a less pronounced, but nonetheless distinct, asymmetry between the two portions of the organisms on either side of the kink regardless of orientation relative to the shortening direction. This indicates that some of this asymmetry was due to uneven growth of the organism on either side of the kink (online Supplementary Material Figs S1a, b, S2). This asymmetry may also be due to the portion of the organism on one side of the kink having been lifted from the seafloor by a current before settling in a slightly different orientation than the unmoved portion of the body (Gehling & Narbonne, Reference Gehling and Narbonne2007). Low-energy contourite currents and higher-energy turbidity currents are both accepted to have affected the epibenthos of the MPER E Surface (Ichaso et al. Reference Ichaso, Dalrymple and Narbonne2007; Matthews et al. Reference Matthews, Liu, Yang, McIlroy, Levell and Condon2021). These shifted body regions would have required an undetermined amount of time to smother the underlying microbial mat sufficiently to create a negative relief impression.
In some kinked specimens the first-order branches closest to the inside of the kink are narrower than those found in straighter parts of the same organism, implying that the inner branches were more laterally constrained than along the straighter portions (Fig. 2d). Other specimens have overlapping branches on the inside margin of the kink, suggesting that they had originally grown normally on the seafloor but were shifted into an overlapping position, perhaps by the action of currents (Figs 5a, 7a) that were strong enough to lift and reposition a portion of the body but not the complete organism. Overlapping first-order branches may have continued to grow in this orientation once re-positioned, though how they would have functioned without direct contact with the sediment is problematic. The high surface area of the lower surface could conceivably have been repurposed for oxygen collection or osmotrophy (Laflamme et al. Reference Laflamme, Xiao and Kowalewski2009), particularly if Fractofusus were a modular colonial organism.
Several kinked specimens of F. misrai show breaks in the otherwise intact outline of the organism, often at the interface between adjacent first-order branches (Fig. 7b, c, d) indicating that they were independent of each other laterally and thus functioned as discrete mechanical (and possibly metabolic) entities. This is in contrast to the highly constrained margins of the Charnida Beothukis, Charnia and Trepassia (McIlroy et al. Reference McIlroy, Hawco, McKean, Nicholls, Pasinetti and Taylor2020). In most cases these gaps occur between adjacent branches that are complete but displaced from their conventional tightly packed arrangement. Rare specimens of F. misrai possess one or more breaks to the outline, usually associated with kinks; these may represent damage to the organism. Since the first-order branches on either side of such gaps show no evidence of compensatory growth to fill in the empty space it is likely that the second-order branches were sufficiently rigid in morphology that they could not spread out owing to gravity or by active growth to fill mechanically created gaps. Some specimens (e.g. Fig. 7b) show gaps between first-order branches at multiple locations, suggesting that the growth and/or orientation of Fractofusus might have been disturbed multiple times during the growth of an individual. One specimen with a gap in the place of a single first-order branch (Fig. 7c, highlighted by arrow) has a faint irregular outline in the approximate position where the distal margin of the missing first-order branch would have been. It is considered that this outline represents the impression of the edge of the missing branch. The specimen must have been buried before the microbial matground could grow over the newly exposed sediment to preserve evidence of its outline in this manner. In other specimens, an irregular margin occurs at the distal edge of incomplete single or multiple first-order branches, suggesting that these branches had been ‘torn’ by some external force (Fig. 7d).
Rare specimens of Fractofusus terminate abruptly, lacking one tapering distal end as if the organism had been torn completely across the midline. The absence of a ghost of the removed portion of the frond might be due to matground regeneration removing evidence of an impression below the lost portion (Fig. 7c). There is no evidence of regeneration from the broken end of the frond, though it is not clear how close to the time of burial the damage occurred. This kind of damage to portions of the reclining Fractofusus strongly suggests that it was sufficiently well anchored to the seafloor to able to resist shear forces that were able to tear the organism.
5.b.2. ‘Folded’ Fractofusus misrai
The possibility of some rare Fractofusus being folded over on themselves has been previously considered (Seilacher, Reference Seilacher1992; Gehling & Narbonne, Reference Gehling and Narbonne2007). A specimen described by O’Brien & King (Reference O’Brien and King2004, pl. 4c; online Supplementary Material Fig. S1c) and later identified by Gehling & Narbonne (Reference Gehling and Narbonne2007) as F. misrai actually has pinnate rather than plumose second-order branching, suggesting that it is the only figured example of folding in F. andersoni.
If folding is accepted, then the relocated part of the Fractofusus frond should be upside down and should lie (in part) on top of the non-folded portion. This has been used to infer that the upper and lower surfaces of rangeomorph fronds in the Avalon Biota were identical (Seilacher, Reference Seilacher1992; Gehling & Narbonne, Reference Gehling and Narbonne2007; Dufour & McIlroy, Reference Dufour, McIlroy, Brasier, McIlroy and McLoughlin2017 a; McIlroy et al. Reference McIlroy, Hawco, McKean, Nicholls, Pasinetti and Taylor2020). To determine which side of a folded frond is in situ, we consider that the deepest impressed side is in situ and the folded side is the poorly preserved portion of the impression. This is likely to be the case because of the quasi-infaunal mode of life of Fractofusus and the shorter period available for matground smothering beneath the newly relocated portion, which is considered necessary for the normal epirelief preservation of Fractofusus. Additionally, the lifted and folded part of Fractofusus would lie atop the in situ portion and could not be preserved in negative epirelief close to the point of folding.
We add to this discussion that the inferred folded specimens may also be a strongly developed kink in which the axis acted as a fulcrum around which a portion of the frond was rotationally relocated rather than flipped. In this alternative model, the lower surface is preserved on both limbs of the damaged specimen, and the relocated portion only smothers the sediment surface where it does not lie atop the stationary part of the organism. We consider that an epifaunal organism made up of two rows of independent first-order branches, joined together only at the midline, would be unlikely to partially flip over without complex twisting and tousling, making kinking a more parsimonious explanation. The implication of this is that we might not know what the top of a Fractofusus looks like.
The location of the fold/fulcrum in kinked or folded specimens of Fractofusus is variable (Figs 2d, 3b, d, 5a, 7a, d), but has not been observed immediately adjacent to the distal tips. In some kinked specimens the longer portion of the organism appears to have been lifted over a smaller section of the body: the shorter body section is preserved in the underlying sediment, concealing the branching detail of the overlapping portion of the overlying section which was clearly not in contact with the seafloor (Fig. 3b). Development of a kink or fold requires that a portion of the organism be lifted from and redeposited on the seafloor sediments and may have been due to hydrodynamic forces associated with deep-water currents. The portion of these kinked Fractofusus specimens that remained in contact with the seafloor must thus have been securely attached to the seafloor sediment. It is conceivable that some poorly preserved Fractofusus specimens may represent the impressions of individuals uprooted and removed by such currents that had been partly effaced by water activity before preservation.
5.c. Modes of reproduction
It has previously been proposed that Ediacaran organisms may have reproduced sexually via the continuous dispersal of small planktonic juveniles (Darroch et al. Reference Darroch, Laflamme and Clapham2013) and asexually via stolons (Mitchell et al. Reference Mitchell, Kenchington, Liu, Matthews and Butterfield2015). Potential evidence has been presented for the latter in F. andersoni (Liu & Dunn, Reference Liu and Dunn2020); there is yet no direct evidence available for the invoked propagules, so this reproductive option cannot be proven or discarded based on current evidence. In our study we have observed several small (≤1 cm), circular structures closely associated with the midline of the organism on approximately the fifth or sixth first-order unit which may represent the lower surface of reproductive bodies (Fig. 6a, b, d, e). These circular impressions may be the lower surface of a structure – akin to cnidarian frustules or podocysts – from which Fractofusus propagules developed and were released, or perhaps the points from which runner-like structures emerged (cf. Mitchell et al. Reference Mitchell, Kenchington, Liu, Matthews and Butterfield2015).
Of the thousands of F. andersoni from the MUN Surface of the Bonavista Peninsula and Brasier Surface in the MPER, only a few have filament-like structures aligned with the midline (Liu & Dunn, Reference Liu and Dunn2020). No specimens of F. misrai with such end-terminal filaments have yet been observed on the E Surface in the MPER. While it is conceivable that some of these filaments may represent reproductive stolons (sensu Mitchell et al. Reference Mitchell, Kenchington, Liu, Matthews and Butterfield2015), the MUN and Brasier surfaces are notable for their superabundance of filaments, which makes it difficult to be sure of biological versus accidental incidence. The morphology that we propose for F. misrai (and F. andersoni) invokes a complex three-dimensional structure (Fig. 9) which might have enabled loose microbial filaments to become tangled in the branches of Fractofusus, much in the same way as they get caught around the stems and holdfasts of other rangeomorph taxa (Liu & Dunn, Reference Liu and Dunn2020). The discovery of additional specimens of Fractofusus associated with filaments, as well as a more complete current analysis, will be required to better understand the nature of this relationship.
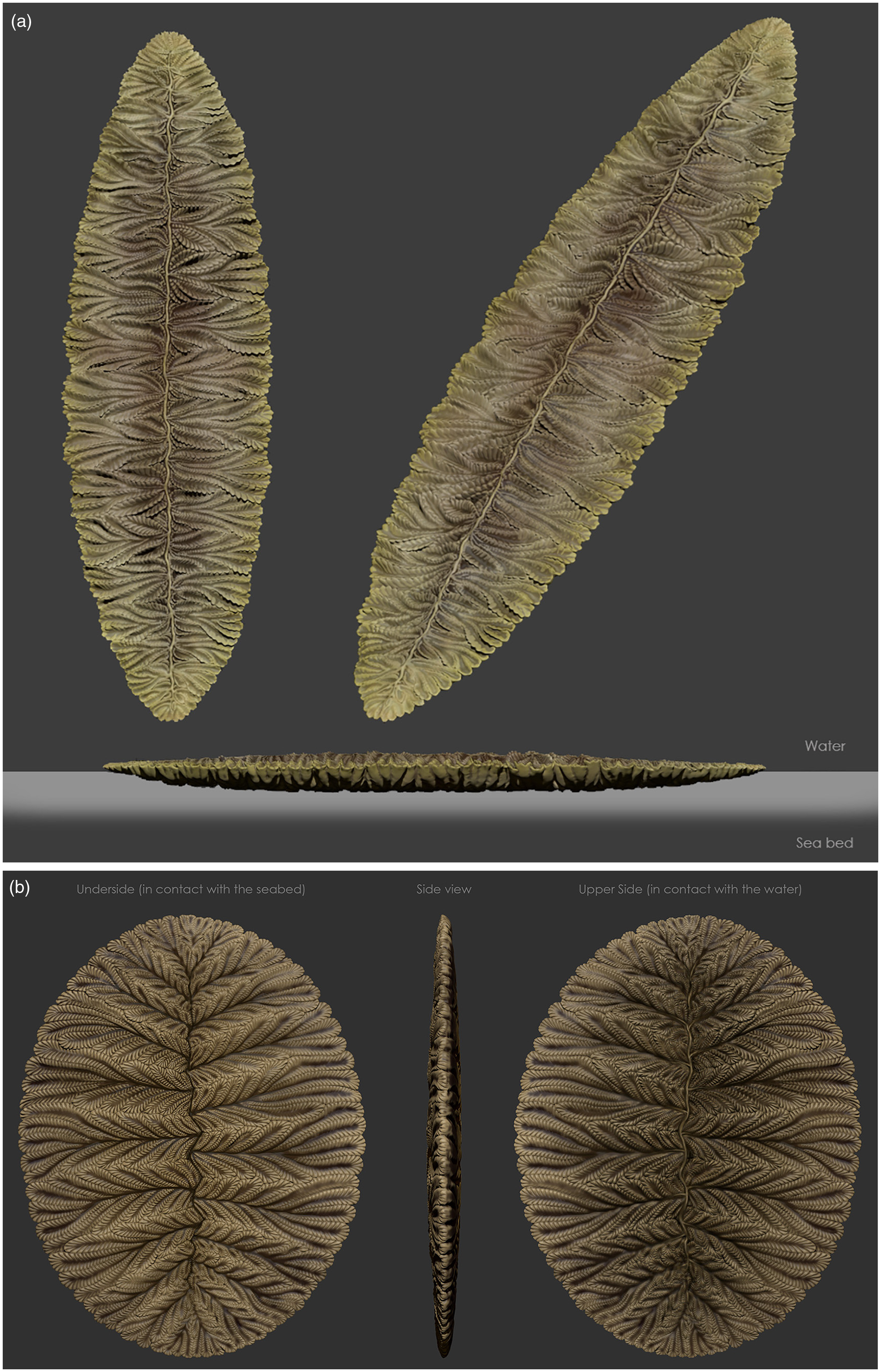
Fig. 9. (a) Reconstruction of F. misrai. (b) Reconstruction of F. andersoni.
5.d. The epifaunal matground mode of life and taphonomy
There is little contention that much of the preservation of fossiliferous deposits in the deep marine facies of Avalonia is due to a combination of microbial matgrounds and pyrite growth (Liu et al. Reference Liu, McIlroy, Antcliffe and Brasier2011, Reference Liu, Kenchington and Mitchell2015; Liu, Reference Liu2016) and casting of the fossils by tuffites (Seilacher, Reference Seilacher1992; Narbonne, Reference Narbonne2005; Matthews et al. Reference Matthews, Liu, Yang, McIlroy, Levell and Condon2021). That the Ediacaran macrobiota grew in or on the extensive contemporary microbial matgrounds is similarly uncontentious (i.e. matground encrusters and mat stickers sensu Seilacher, Reference Seilacher1999). Non-motile epifaunal matground dwelling organisms such as characterize much of the Avalon Assemblage (Waggoner, Reference Waggoner2003) are considered to have smothered the contemporary matground as they grew along the sediment–water interface (McIlroy et al. Reference McIlroy, Brasier and Lang2009, Reference McIlroy, Hawco, McKean, Nicholls, Pasinetti and Taylor2020, Reference McIlroy, Dufour, Taylor and Nicholls2021; Dufour & McIlroy, Reference Dufour, McIlroy, Brasier, McIlroy and McLoughlin2017 a,b). This matground-associated mode of life is responsible for high-fidelity preservation of the lower surface of reclining organisms such as Fractofusus (Gehling & Narbonne, Reference Gehling and Narbonne2007) and Beothukis (McIlroy et al. Reference McIlroy, Hawco, McKean, Nicholls, Pasinetti and Taylor2020, Reference McIlroy, Dufour, Taylor and Nicholls2021). The exact nature of the matgrounds themselves in terms of their microbial consortia, their physical integrity and textural properties during life is effectively unknown. Most modern matgrounds include relatively large amounts of microbial biomass and dissolved organic matter (DOM) production (Prieto-Barajas et al. Reference Prieto-Barajas, Valencia-Cantero and Santoyo2018), but also, particularly in fine-grained sediments, restrict oxygen diffusion into the porewater system by occlusion of pore throats by biomass, resulting in porewater dysoxia or even anoxia close to the sediment–water interface (Lawrence et al. Reference Lawrence, Wolfaardt and Korber1994). The fine-grained sediment below the Ediacaran matgrounds of Avalonia was most commonly pelagite or hemipelagite, probably with relatively high amounts of porewater (Brasier et al. Reference Brasier, Liu, Menon, Matthews, McIlroy and Wacey2013).
We consider that it is the direct interaction of reclining organisms with the sediment that causes the high fidelity of preservation. The deepest relief – and at times the best quality of preservation – is close to the axis of Fractofusus: the first-order rangeomorph units closest to one or both distal ends (e.g. Figs 2a, d, 3a, c, 5d, 6a, 10b) and/or the distal ends of the rangeomorph branches along the lateral margins of the body (e.g. Figs 2c, d, 3a, 10b) sometimes produce shallower, less detailed impressions in the underlying sediment. This taphonomic difference from the centre to the margins and the tips of immature Fractofusus is evidence of direct matground smothering by the organism and its sediment displacing mode of life. The growth of Fractofusus occurred at both distal tips and along its lateral margins, likely by both insertion and inflation, causing the branches to expand progressively outwards over the surrounding matground, smothering the microbiota and progressively creating negative surficial relief (cf. McIlroy et al. Reference McIlroy, Brasier and Lang2009). With smothering of the surficial matground, the Fractofusus branch tips could grow further downwards into the sediment. We therefore consider that Fractofusus was intimately associated with the matground, growing below the local plane of the sediment–water interface by matground smothering, in a manner that likely also provided some protection from seafloor currents.

Fig. 10. (a) Incomplete F. misrai showing unfurled subsidiary second-order branch (blue) overlapped by furled subsidiary second-order branch of adjacent first-order branch (yellow); arrows indicate non-bifurcating second-order branches (field photograph). Scale bar = 10 mm. (b) Adjacent specimens, smaller individual (upper right) curved to avoid overgrowth with larger specimen (cast photograph). Scale bar = 20 mm. (c) Adjacent specimens, smaller individual (upper right) curved to avoid overgrowth with larger specimen (cast photograph). Scale bar = 30 mm.
5.e. Taphonomic effects of current action on Fractofusus misrai
The frond tips and distal margins of the first-order branches of some F. misrai specimens show considerable variability, with distal margins of first-order branches ranging from scalloped to straight in outline. This indicates that the distal regions of these branches may have been unable to impress as deeply into the sediment as the axial region of the organism. The distal branch tips appear to have been thinner and more flexible than the proximal regions, where the rangeomorph units are larger. This may also be due in part to the distal branch tips being more loosely packed compared to the midline, where branches were more tightly constrained. The distal margins of first-order branches are also more likely to have been susceptible to lifting by currents (cf. the taphonomic model of Charnia lateral margins in Laflamme et al. Reference Laflamme, Narbonne, Anderson, Vickers-Rich and Komarower2007) since they are likely to have risen above the sediment–water interface when exposed to currents on the up-current margin. If the current was sediment laden, this may well have allowed sediment to come between the lower surface of Fractofusus and the associated seafloor impression, thus reducing the quality of preservation. In some specimens, the lateral margin on the up-current side of the Fractofusus frond does indeed consist of a straight, sharp line that truncates the otherwise scalloped distal margins of several first-order units (e.g. Fig. 5a; cf. Laflamme et al. Reference Laflamme, Narbonne, Anderson, Vickers-Rich and Komarower2007), and is therefore likely to be a taphonomic effect.
Some specimens of F. misrai show inconsistencies in first-order branch length within a row of first-order rangeomorph units. This is most notable with curved specimens, in which some parts of the organism would have been more susceptible to lift due to current drag resulting from portions of the frond edge being unequally exposed to the sediment-laden currents (Fig. 5c). Other specimens possess distinctly scalloped outer margins to each first-order branch (e.g. Fig. 5b), which we interpret to be the result of the growth of a Fractofusus without being disturbed by currents. Scalloped margins are here considered to be more readily preserved on the down-current side of the organism. This kind of scalloped margin is rare on the E Surface, suggesting the presence of a background current, perhaps the geostrophic currents inferred by earlier studies (Wood et al. Reference Wood, Dalrymple, Narbonne, Gehling and Clapham2003). Erosional scouring of the matground in the lee of Fractofusus has not been documented, implying that the adjacent matground was not significantly affected by eddying in the lee of Fractofusus, perhaps due to microbial binding of the sediment surface increasing its shear strength.
5.f. Morphological reconstruction of Fractofusus misrai
The earliest reconstructions of the Avalon Assemblage considered that Fractofusus lay with the lower surface on the seafloor with separate feeding and/or respiratory structures that projected into the water column (Jenkins, Reference Jenkins, Lipps and Signor1992). Most subsequent published reconstructions of Fractofusus have been rather biconvex with a plane of symmetry parallel to the seafloor (Gehling & Narbonne, Reference Gehling and Narbonne2007; Mitchell et al. Reference Mitchell, Kenchington, Liu, Matthews and Butterfield2015), notwithstanding the three- and four-vaned reconstructions posited, and rejected, by Gehling & Narbonne (Reference Gehling and Narbonne2007). Mitchell & Kenchington (Reference Mitchell and Kenchington2018) estimated Fractofusus height as being equal to one quarter of its width, suggesting that Fractofusus reached almost 5 cm height. This seems to be at odds with the conventional model suggesting that the upper and lower surfaces of Fractofusus were identical. No taphonomic/biostratinomic arguments for that inference have been provided, suggesting that the impact of Fractofusus on the benthic boundary layer is perhaps inflated. Several authors have considered that the first-order rangeomorph units of Fractofusus were independent of each other, prone to modification by currents (e.g. Brasier & Antcliffe, Reference Brasier and Antcliffe2009; Brasier et al. Reference Brasier, Antcliffe and Liu2012) or capable of active autonomous movement (Jenkins, Reference Jenkins, Lipps and Signor1992).
The model that individual rangeomorph units of Fractofusus were prone to current modification (Brasier & Antcliffe, Reference Brasier and Antcliffe2009) is based on the observations that (a) the first- and second-order branches could become disordered (‘tousled’ or ‘dishevelled’) when they resettled onto the seafloor (e.g. Fig. 6c) and (b) the second-order units of adjacent first-order branches could overlap each other (Fig. 10a). The lateral independence of individual first-order branches, along with the inference that they could be disrupted en masse during folding (Seilacher, Reference Seilacher1992, Reference Seilacher1999; Gehling & Narbonne, Reference Gehling and Narbonne2007), gives credence to the idea that first-order units may have been connected at their bases by a medial structure that was situated above the seafloor surface (Fig. 9).
We consider that the upper and lower surfaces of F. misrai were not identical, but that the convex lower surfaces of F. misrai that produce the characteristic negative epirelief impressions curled upwards towards the overlying seawater in the portions that are furled (sensu Brasier et al. Reference Brasier, Antcliffe and Liu2012) and were displayed – and thus more seafloor parallel – at their tips. Extrapolation of this model leads to the reconstruction of the upper surface of F. misrai (and by extension F. andersoni; Fig. 9b) as being more concave in morphology, having feathered edges that extended into the water column (Figs 6c–e, 9). The concavity of this upper surface is illustrated conservatively here; it might have been considerably more pronounced than we have presented it Figure 9, but it is difficult to estimate the extent of this feathering in the absence of Fractofusus preserved in three dimensions.
The second-order branches of F. misrai show considerable variation in size and shape between first-order units (Fig. 6d, e). The two central second-order branches diverge from each other very close to the specimen midline and are most likely attached to each other at the point of attachment to the midline. One of the two medial second-order branches bifurcate distally, lending a pronounced asymmetry to the first-order branches. Bifurcating branches commonly show at least two bifurcations. It remains possible that all second-order branches do bifurcate, but in some branches the division occurs in the portion of the branch that was not in contact with the seafloor; instead it would have been visible from above on the upper surface of the frond that is not preserved (Fig. 10a).
Adjacent to the medial first-order rangeomorph unit are ‘subsidiary branches’ that do not bifurcate. These subsidiary branches, which are present adjacent to the midline – and preserved in the broadly triangular gaps between adjacent first-order rangeomorph units (Fig. 6d, e) – are typically uniserial rangeomorph units. From this we infer that this second series of second-order rangeomorph units were curled to be sub-perpendicular to the seafloor and were adpressed against the subsidiary branch of the immediately adjacent first-order unit (Figs 6d, e, 9).
The medial first-order and subsidiary branches emerge from the axis of F. misrai, but there is no clear evidence of the attachment of these branches to their point of origin. Many specimens (e.g. Figs 5d, 6d) demonstrate fine branching details immediately adjacent to the organism midline, with no evidence of branch attachment visible. This suggests that the branches extend from a medial structure that lay somewhere above the organism–sediment interface. We consider that this structure would have been rod-like (Fig. 9). It has been suggested that some Ediacaran organisms with fronds may have developed without a central stem structure (i.e. Dunn et al. Reference Dunn, Wilby, Kenchington, Grazhdankin, Donoghue and Liu2019), exhibiting a mode of growth in which new branches emerged laterally from existing branches instead of a central stem. This model is feasible but is difficult to apply to organisms such as Fractofusus that grow in two directions simultaneously. If growth in Fractofusus commenced from a single medial central point and developed in opposite directions, with new branches emerging from previously existing branches, a symmetrical midline would be expected in the middle region of the organism where these initial branches developed. This is not the case for F. misrai, which possesses irregular first-order branch symmetry across the midline in the middle region of the organism.
Some kinked specimens of F. misrai show disruption to the first- and second-order branches, usually along the outer margin of the frond, suggesting that the irregularity in shape was caused by an external force such as a unidirectional turbidity current. In other cases, the outer margin of specimens with kinked central axes are smooth, suggesting either that the frond was little damaged by the current that dislocated the central axis, or that regenerative growth occurred on the outer margin to occlude gaps between fronds created by physical disturbance. The nature of regenerative growth and damage repair can be an important means of determining phylogenetic affinities (e.g. McIlroy et al. Reference McIlroy, Green and Brasier2001) but is seldom reported among elements of the Ediacaran biotas (Wilby et al. Reference Wilby, Kenchington and Wilby2015; Kenchington et al. Reference Kenchington, Dunn and Wilby2018 a).
The type description of Fractofusus posits that the upper and lower surfaces of Fractofusus were identical, but also that the plumose branching within each first-order branch would have resulted in ‘lateral crowding and rotation of branching elements out of the plane of the vane’ in order to account for the variability in expression of rangeomorph units as impressed onto the seafloor (Gehling & Narbonne, Reference Gehling and Narbonne2007). Their assertion that both the upper and lower sides of Fractofusus were identical, based on rare specimens with a portion of the body apparently folded backwards over the remainder of the organism (Seilacher, Reference Seilacher1992; Gehling & Narbonne, Reference Gehling and Narbonne2007; see Fig. 3b), is directly in conflict with this hypothesis. However, if instead of flipping upside down, the folded specimens are simply an end-member variant of the comparatively common kinked specimens, then it would be expected that the lower surface is always preserved. We additionally consider that if, as all the evidence we outline herein suggests, the first-order units were independent from one another and only connected by a medial rod that lay above the seafloor, then rotation around a point seems plausible. Such rotation might be expected to put strain on this presumably flexible medial rod, which if it were to break, might account for the rare incomplete specimens (Fig. 7c). It is noteworthy that in no instances do we see the ghost of the missing portion in the matground preserved, indicating that sufficient time passed between repositioning and preservation for matground regeneration to occur (cf. McIlroy et al. Reference McIlroy, Brasier and Lang2009).
5.g. The spatial distribution of Fractofusus misrai
Although F. misrai is found in large numbers on the Mistaken Point E Surface, less than 1 % of specimens come into contact with one other, and where contact occurs only a small portion of both specimens overlap, typically with side-to-side contact or slight marginal overlap. In almost all cases the larger specimen is fully preserved with the smaller Fractofusus wrapped around it or contorted against it (Fig. 10c), although there may have been some interdigitating of first-order branches between some of the overlapping branches (Fig. 10b). Overlap in which one specimen crosses the midline of the second is very rare (<0.1 %) and may represent living F. misrai overgrowing a decomposing specimen (e.g. Fig. 6a). It is thus considered that the upper surface of F. misrai may have been sufficiently high above the sediment surface to prevent them from being overgrown by other specimens (cf. Gehling & Narbonne, Reference Gehling and Narbonne2007) or there may have been some defence mechanism associated with the midline. Small F. misrai growing towards and in close proximity to a larger F. misrai typically develop a curve to the midline such that they lie alongside or in contact with the other organism along a portion of the margin, which is a possible example of phobotropism (Fig. 10b, c).
5.h. Developmental biology
There has been little consensus regarding the developmental biology of Fractofusus. It has previously been suggested that the number of first-order branches (‘modules’) present is species specific and is determined early in the development of an individual, with ensuing growth occurring primarily through inflation (Gehling & Narbonne, Reference Gehling and Narbonne2007). An alternative view is that Fractofusus grew through a combination of inflation of existing first-order branches coupled with the addition of new first-order branches at the distal ends of the organism (Anderson & Conway Morris, Reference Anderson and Conway Morris1982). The high variability in numbers of first-order branches reported between different individuals of the same size in the current study, ranging from 8 to at least 30, suggests that new first-order branches must have developed at the distal tips of the biterminal body during the lifespan of Fractofusus.
Comparison of the number of first-order branches per row and maximum specimen length (n = 39 straight, 81 curved/kinked F. misrai) showed no clear relationship between maximum specimen length and first-order branch number among either group, with correlation coefficients of −0.044 and 0.21 reported for straight and curved/kinked specimens, respectively (Fig. 11). The ontogeny and development of Fractofusus is thus in need of careful assessment, but it would seem that until a Fractofusus has developed around eight pairs of first-order rangeomorph units it either has a lifestyle that renders it unpreservable (e.g. being entirely planktonic or perhaps motile employing cilia for locomotion rather than irrigation (cf. Dufour & McIlroy, Reference Dufour, McIlroy, Brasier, McIlroy and McLoughlin2017 a)), or perhaps possessed an undifferentiated surficial structure. Additionally, the lack of relationship between length and number of modules may suggest a strong palaeoenvironmental control on morphology (i.e. ecophenotypism; cf. Gehling & Narbonne, Reference Gehling and Narbonne2007; Liu et al. Reference Liu, Kenchington and Mitchell2015). Such differences within the assemblages may hint at a complex community structure and the presence of several cohorts of individuals that experienced different environmental stresses during growth phases. This issue is far from resolved: Darroch et al. (Reference Darroch, Laflamme and Clapham2013) did not identify size cohorts within the Fractofusus population in the Mistaken Point community, while Mitchell et al. (Reference Mitchell, Kenchington, Liu, Matthews and Butterfield2015) identified three Fractofusus size classes.

Fig. 11. Number of primary branches per row/maximum length of Fractofusus misrai specimens (blue = kinked specimens; green = straight specimens). Kinked specimens: R2 = 0.046; p-value = 0.054. Straight specimens: R2 = 0.0019; p-value = 0.19.
5.i. Systematic palaeontology
Class RANGEOMORPHA Pflüg, Reference Pflüg1972
Genus Fractofusus Gehling & Narbonne, Reference Gehling and Narbonne2007
Type species. Fractofusus misrai Gehling & Narbonne, Reference Gehling and Narbonne2007, figs 2–19.
Reference Seilacher1999 ‘Vendofusus’, Seilacher, fig. 3.
non Reference O’Brien and King2004 ‘spindle-shaped frond’, O’Brien & King, fig. 4c.
Reference Gehling and Narbonne2007 Fractofusus misrai, Gehling & Narbonne, figs 4b, 5–11.
non Reference Hofmann, O’Brien and King2008 Fractofusus misrai, Hofmann et al., fig. 20.1.
?Reference Hofmann, O’Brien and King2008 Fractofusus misrai, Hofmann et al., fig. 20.4.
Reference Brasier and Antcliffe2009 Fractofusus misrai, Brasier & Antcliffe, fig. 9.
Reference Brasier, Antcliffe and Liu2012 Fractofusus misrai, Brasier et al., figs 7C, D, 8E.
Reference Dornbos, Clapham, Frasier, Laflamme, Solan, Aspden and Paterson2012 Fractofusus misrai, Dornbos et al., fig. 5.2f.
Reference Gradstein, Ogg, Schmitz and Ogg2012 Fractofusus misrai, Gradstein et al., fig. 18.3G.
Reference Darroch, Laflamme and Clapham2013 Fractofusus misrai, Darroch et al., fig. 2A.
Reference Seilacher and Gishlick2014 Fractofusus misrai, Seilacher & Gishlick, pl. 9.2.
Reference Antcliffe, Hancy and Brasier2015 Fractofusus, Antcliffe et al., figs 3, 10.
Reference Mitchell, Kenchington, Liu, Matthews and Butterfield2015 Fractofusus misrai, Mitchell et al., fig. 1b.
Reference Dufour, McIlroy, Brasier, McIlroy and McLoughlin2017 a Fractofusus misrai, Dufour & McIlroy, fig. 1c.
Reference Dufour, McIlroy, Brasier, McIlroy and McLoughlin2017 a Fractofusus, Dufour & McIlroy, fig. 3.
Reference Dufour and McIlroy2017 b Fractofusus misrai, Dufour & McIlroy, fig. 2d.
Reference Darroch, Laflamme and Wagoner2018 Fractofusus, Darroch et al., fig. 1a.
Emended specific diagnosis. Fusiform-shaped body widest at midpoint and narrowing towards distal ends, with two longitudinal branch rows meeting along straight to zig-zagged medial axis. Rows composed of a series of first-order rangeomorph branches arranged perpendicular to and attached at the midline but free laterally. First-order branches symmetrical or exhibiting glide plane symmetry across midline. First-order branches with multi-bifurcating medial second-order rangeomorph branch and unbranching lateral second-order branches, all showing marginal invaginations; second-order branches free laterally. Upper body surface concave with first-order branches growing downward from midline but upward at lateral edges, producing scalloped outer margin.
Description. All known specimens of Fractofusus misrai represent the preserved impressions of organisms on the Ediacaran seafloor, and so are imprints of the lower surface of the organism only. Fractofusus misrai varies in shape from acicular through fusiform to elliptic, with fusiform being the most common outline. The frond is composed of two rows of first-order rangeomorph branches, arranged on either side of the medial axis. Numerous specimens have a curved or kinked medial axis, sometimes with more than one curve or kink (no specimens with both kink(s) and curve(s) have been noted). The frond is normally widest at the midpoint, gradually tapering towards the termini, which are often poorly preserved.
The two rows consist of a series of first-order rangeomorph branches that are orientated perpendicularly from the midline. First-order branches range in shape from almost square to elongate and rectangular in shape; there is typically no separation between adjacent branches (Fig. 2a). The first-order branches are made up of second-order rangeomorph branches (Figs 5d, 6d, e, 10a). A single second-order branch emerges at the medial axis of each first-order branch, presumably originating at the midline but at a level above the organism–sediment interface and so is not preserved (Fig. 5d). The medial second-order branch widens and bifurcates close to the midline. One element of the second-order branch bifurcates, usually at least twice; the other element of the medial branch widens distally but does not bifurcate (Figs 6d, 10a). The medial second-order branch thus terminates in five or six subsidiary branches at the margin of the organism. Non-bifurcating subsidiary second-order branches are usually uniserial and grow immediately to either side of the base of the medial second-order branch, often at a high angle to the centre-line of the associated first-order rangeomorph unit (Figs 5d, 6d, e, 9). There may be one or two subsidiary branches on either side of the medial second-order branch. Where there are two subsidiary branches to one side of the medial second-order branch, the innermost subsidiary branch is notably larger than the outermost (Fig. 6d, e).
The second-order branches possessed what have been previously referred to as third-order (‘tertiary’) branches along both lateral edges; however, there is no evidence of true branching between these subunits; therefore we interpret them as invaginations along the lateral margins of the second-order branches rather than true branching structures. These third-order ‘branches’ usually develop symmetrically along the length of the second-order branches, although glide plane symmetry can be present. The few known folded specimens appear to have the more tubular morphology of the second-order branches, and little to no evidence for third-order branching (e.g. Gehling & Narbonne, Reference Gehling and Narbonne2007, fig. 8c). Second-order rangeomorph branches are often furled but may be unfurled, particularly at the distal branch tips; because of this furling, the lateral invaginations are often visible on only one lateral margin of second-order branches (Figs 6d, e, 10a) but may be visible on both branch edges (Fig. 10a). This high variability in branch furling suggests that the second-order branches possessed some degree of flexibility and would have been able to rotate or twist slightly, resulting in different parts of second-order branch(es) being exposed to the sediment underlying the organism (Fig. 10a). The upper and lower surface of these second-order branches possessed the same surficial invagination patterns; however, the upper and lower surfaces of F. misrai would have been different owing to their position in relation to the underlying sediment. The second-order branches initially grew downwards into the sediment near the midline with the branches turning upwards into the water column near the distal branch margins, resulting in a concave upper surface (with a raised medial region) and a convex lower surface.
Remarks. Fractofusus misrai is the only rangeomorph in the Avalon Assemblage that commonly overlaps other taxa. Fractofusus misrai is commonly found to overlie ivesheadiomorph pseudofossils (Liu et al. Reference Liu, McIlroy, Antcliffe and Brasier2011; Fig. 12), either completely or in part; Fractofusus was shown by Mitchell & Butterfield (Reference Mitchell and Butterfield2018) to be positively correlated with ivesheadiomorphs and lobate discs (as well as Plumeropriscum and holdfast discs) on the E Surface. Some Fractofusus are very poorly preserved and are thus likely to have been dead and partially decayed specimens at the time of fossilization (see Liu et al. Reference Liu, McIlroy, Antcliffe and Brasier2011; Antcliffe et al. Reference Antcliffe, Hancy and Brasier2015; Fig. 12). Other ivesheadiomorph-associated Fractofusus specimens preserve fine branching details, suggesting they were growing on pre-existing ivesheadiomorphs (Fig. 3a). Previous studies have already reported Fractofusus specimens overlying ivesheadiomorphs at Mistaken Point (Gehling & Narbonne, Reference Gehling and Narbonne2007; Liu et al. Reference Liu, McIlroy, Antcliffe and Brasier2011). These Fractofusus specimens may have been utilizing the nutrients made available by the decay of whatever organism(s) was decomposing on the seafloor (Dufour & McIlroy, Reference Dufour, McIlroy, Brasier, McIlroy and McLoughlin2017 a), though as immotile organisms they could not be considered to be scavengers.

Fig. 12. (a) Fractofusus misrai as part of ivesheadiomorphs. Scale bar = 20 mm. (b) Ivesheadiomorph with multiple Fractofusus misrai arranged in radiating and curving arrays. Scale bar = 40 mm. (c) Diagrammatic seafloor reconstruction showing the Fractofusus/ivesheadiomorph matground/necromass relationship: the matground on top of the necromass is inferred to have been prone to tearing during gas escape (after McIlroy et al. Reference McIlroy, Dufour, Taylor and Nicholls2021) (cast photographs).
Brasier & Antcliffe (Reference Brasier and Antcliffe2009) described a large ivesheadiomorph from Mistaken Point as Ivesheadia cf. lobata (now known to be a pseudofossil) that was made up of subunits closely resembling F. misrai but arranged in ‘ramifying, radiating and curving arrays (p. 626)’ (Fig. 12b). Re-examination of this specimen indicates that it is a large ivesheadiomorph with numerous specimens of F. misrai overgrowing it. While some of the ‘Fractofusus-like subunits’ appear to radiate out from an unidentified central point, others clearly grew with an orientation contrary to a radial arrangement. Also, some of the Fractofusus specimens extend beyond the outer edge of the ivesheadiomorph. Several of the Fractofusus specimens in question are preserved with notably less detail than others, suggesting that they may have actually been part of the original necromass making up this large ivesheadiomorph.
6. Conclusions
Fractofusus misrai exhibited a quasi-infaunal mode of life, growing downwards into matground-covered seafloor sediment with the branch tips rising above the seafloor. Adjacent specimens very rarely came into physical contact with each other, despite their abundance. First-order branches grew independently of each other, both laterally and across the midline. Second-order branches emerged from the centre of the body with the medial branches bifurcating multiple times as they extended to the outer margin; there is no evidence that the lateral subsidiary second-order branches bifurcated. The elongate fusiform body shape predominates, although ‘curved’ and ‘kinked’ specimens are well represented: atypical forms may have been caused by uneven growth in response to environmental factors and/or mechanical movement of the organisms by seafloor currents. The upper and lower surfaces of F. misrai were likely slightly different, representing the parts of rangeomorph elements that are not in contact with the sediment (following the model of Brasier et al. Reference Brasier, Antcliffe and Liu2012). The specimens previously recognized as ‘folded over’ are here interpreted as ‘kinked’, with the lower surface of the organism remaining in contact with the seafloor across both sides of the kink. Reduced quality of preservation near the margins and tips suggests that the edges of F. misrai did not adhere to the seafloor sediment and likely extended over the microbial matground surrounding the organism, eventually smothering and possibly exploiting it through time. Fractofusus misrai commonly overlay necromass on the Ediacaran seafloor, suggesting that they were tolerant of sulfidic porewaters and might have possessed sulfur oxidizing ectosymbionts. The combination of quasi-infaunal growth and chemosynthetic sedimentary symbionts may be responsible for the particularly good preservation seen in many F. misrai specimens.
Supplementary material
To view supplementary material for this article, please visit https://doi.org/10.1017/S0016756822000723
Acknowledgements
We wish to thank the people of the communities of Portugal Cove South, Trepassey and Port Union for their support over the course of this project. We also wish to thank Mark King and the staff at the Edge of Avalon Interpretation Centre in Portugal Cove South for their assistance and support over many years. Thanks are also extended to Nathalie DjanChekar (for access to the fossil collections at The Rooms in St John’s, Newfoundland and Labrador) and to the two anonymous reviewers whose comments helped to improve the manuscript. Daniel Pérez Pinedo is thanked for his assistance with statistical analyses. Research in the Mistaken Point Ecological Reserve and on the Bonavista Peninsula was conducted under permit from both the Department of Fisheries and Land Resources, and the Department of Tourism, Culture, Industry and Innovation. The Oxford University Museum of Natural History is gratefully acknowledged for granting a visiting fellowship to R. Taylor. This work has developed in part through discussions with F. S. Dunn, J. J. Matthews, D. Pérez Pinedo, C. McKean, G. Pasinetti, A. G. Liu, C. G. Kenchington, E. G. Mitchell and J. B. Hawco. Financial support for this project comes from a Natural Sciences and Engineering Research Council Discovery Grant and Discovery Accelerator Supplement funding to D. McIlroy.
It is illegal to collect fossils within the Mistaken Point Ecological Reserve due to the Wilderness and Ecological Reserves Act, and regulations made thereunder. Other sites possessing significant Ediacaran fossils within Newfoundland, including those on the Bonavista Peninsula, are protected by the Historic Resources Act and subsequent regulations. Access to Ediacaran fossiliferous surfaces in Newfoundland requires a research permit from the Government of Newfoundland and Labrador in accordance with Regulation 67/11 of the Historic Resources Act 2011.
Conflict of interest
None.