1. Introduction
The Central Asian Orogenic Belt (CAOB) represents the largest accretionary orogen in the world (Figure 1(a)) and is delimited by the Siberian, North China and Tarim cratons that extend through the territories of the Urals, Kazakhstan, Tien Shan, northwestern China, Mongolia and Southern Siberia. The CAOB displays a prolonged tectonic history of development from the Neoproterozoic to the early Mesozoic that involved a series of accretionary events during the evolution of the Palaeo-Asian Ocean (Windley et al. Reference Windley, Alexeiev, Xiao, Kröner and Badarch2007; Yarmolyuk & Degtyarev, Reference Yarmolyuk and Degtyarev2019). The formation of a vast volume of continental crust within the CAOB took place largely during the Precambrian (mostly Palaeo- and Mesoproterozoic) and in part during the early Palaeozoic (Kröner et al. Reference Kröner, Kovach, Belousova, Hegner, Armstrong, Dolgopolova, Seltmann, Alexeiev, Hoffmann, Wong, Sun, Cai, Wang, Tong, Wilde, Degtyarev and Rytsk2014, Reference Kröner, Kovach, Alexeiev, Wang, Wong, Degtyarev and Kozakov2017a; Degtyarev et al. Reference Degtyarev, Yakubchuk, Tretyakov, Kotov and Kovach2017). Consequently, the CAOB contains large domains of low- to moderate-grade metamorphosed Precambrian crustal rocks (gneissic granites, orthogneisses and felsic metavolcanic rocks).

Figure 1. (a) Location of the Central Asian Orogenic Belt (CAOB) in North Eurasia (after Degtyarev et al. Reference Degtyarev, Luchitskaya, Tretyakov, Pilitsyna and Yakubchuk2021); (b) Precambrian terranes distributed within the western segment of the CAOB: AY – Aktau-Yili, CT – Chinese Central Tien Shan, E–N – Erementau-Niyaz, IS – Issyk-Kul, K – Kokchetav, ZT – Zheltau, Ch-K – Chu-Kendyktas, K-D – Karatau-Dzhebagly, K-T – Karatau-Talas, N–S – Naryn-Sarydzhaz, Ul – Ulutau (after Degtyarev et al. Reference Degtyarev, Yakubchuk, Tretyakov, Kotov and Kovach2017).
In the study area of the southwestern segment of the CAOB, Precambrian metamorphic complexes are commonly associated with high- and ultrahigh-pressure (HP–UHP) rocks, which are mainly eclogites and garnet-bearing gneisses (HP granulites). These high-grade rocks were derived from the reworking of Precambrian crust in subduction zones during the Cambrian–Early Ordovician, when different oceanic basins of the Palaeo-Asian Ocean were closed (e.g. Kröner et al. Reference Kröner, Alexeiev, Hegner, Rojas-Agramonte, Corsini, Chao, Wong, Windley, Liu and Tretyakov2012; Konopelko et al. Reference Konopelko, Kullerud, Apayarov, Sakiev, Baruleva, Ravna and Lepekhina2012; Konopelko & Klemd, Reference Konopelko and Klemd2016; Pilitsyna et al. Reference Pilitsyna, Tretyakov, Degtyarev, Salnikova, Kotov, Kovach, Wang, Batanova, Plotkina, Tolmacheva, Ermolaev and Lee2019). The HP–UHP formations known from the Issyk-Kul terrane (Makbal ultrahigh-pressure (UHP) complex; North Tien Shan), Chu-Kendyktas terrane (Aktyuz HP complex; North Tien Shan) and Zheltau terrane (Koyandy HP complex; South Kazakhstan) have been the main focus of many studies aimed at estimating the timing, parameters and settings of the metamorphism of Precambrian continental crust and comparing these data with the extant results of investigations of eclogites from HP–UHP metamorphic complexes.
Here, we report new data on the ages of the HP paragneisses of the Koyandy complex (Zheltau terrane; South Kazakhstan), elucidate the P–T evolution of these rocks and correlate our results with those reported previously from the adjacent Aktyuz complex (Chu-Kendyktas terrane; North Tien Shan). We also discuss the Precambrian tectonic affinities of the protoliths of the studied HP paragneisses in the Koyandy complex and similar formations of the adjacent terranes and their late Cambrian–Early Ordovician metamorphic evolution. In the last part of the paper, we evaluate the existing geodynamic models of the early Palaeozoic evolution of HP–UHP rock assemblages in the southwestern segment of the CAOB in light of our new data reported in this study.
2. Geological background
The crustal makeup of the CAOB includes blocks of Precambrian crust of variable sizes and fragments of island arc and ophiolite fragments preserved within early Palaeozoic accretionary prism complexes (Yarmolyuk & Degtyarev, Reference Yarmolyuk and Degtyarev2019). In the western part of the CAOB exposed in Kazakhstan, Tien Shan and Northwestern (NW) China, Precambrian terranes occur as narrow tectonic zones that are several hundred km long and 50 to 100 km wide (Figure 1(b)). These Precambrian terranes commonly contain a basement composed of strongly to moderately deformed and metamorphosed Meso- and Neoproterozoic granitoids and/or felsic volcanic rocks (gneissic granites and orthogneisses). These crystalline rocks are covered by weakly deformed sedimentary cover consisting mainly of Ediacaran–Cambrian quartzites and shales (Degtyarev et al. Reference Degtyarev, Yakubchuk, Tretyakov, Kotov and Kovach2017; Kanygina et al. Reference Kanygina, Tretyakov, Degtyarev, Kovach, Skuzovatov, Pang, Wang and Lee2021; Skoblenko et al. Reference Skoblenko, Degtyarev, Kanygina, Pang and Lee2022). Extant geochronological and Sm–Nd isotope data show that the majority of the granitoids in the basement were derived from partial melting of an early Precambrian continental crust; juvenile crustal material contribution to the magmatic evolution of these granitoids was minimal (e.g. Kröner et al. Reference Kröner, Alexeiev, Hegner, Rojas-Agramonte, Corsini, Chao, Wong, Windley, Liu and Tretyakov2012, Reference Kröner, Alexeiev, Rojas-Agramonte, Hegner, Wong, Xia, Belousova, Mikolaichuk, Seltmann, Liu and Kiselev2013; Degtyarev et al. Reference Degtyarev, Yakubchuk, Tretyakov, Kotov and Kovach2017; Kovach et al. Reference Kovach, Degtyarev, Tretyakov, Kotov, Tolmacheva, Wang, Chung, Lee and Jahn2017).
In the southwestern (SW) part of the CAOB, the Precambrian crust exposed in the Zheltau and Chu-Kendyktas terranes in South Kazakhstan and in the North Tien Shan and in the Issyk-Kul terrane in the North Tien Shan (Figure 1(b)) is composed of metamorphosed Palaeo-, Meso- and Neoproterozoic felsic rocks (Degtyarev et al. Reference Degtyarev, Tretyakov, Ryazantsev, Kotov, Salnikova, Aleksandrov and Anisimova2011, Reference Degtyarev, Yakubchuk, Tretyakov, Kotov and Kovach2017; Kröner et al. Reference Kröner, Windley, Badarch, Tomurtogoo, Hegner, Jahn, Gruschka, Khain, Demoux and Wingate2007, Reference Kröner, Alexeiev, Hegner, Rojas-Agramonte, Corsini, Chao, Wong, Windley, Liu and Tretyakov2012, Reference Kröner, Alexeiev, Rojas-Agramonte, Hegner, Wong, Xia, Belousova, Mikolaichuk, Seltmann, Liu and Kiselev2013; Pilitsyna et al. Reference Pilitsyna, Tretyakov, Degtyarev, Salnikova, Kotov, Kovach, Wang, Batanova, Plotkina, Tolmacheva, Ermolaev and Lee2019; Skoblenko et al. Reference Skoblenko, Degtyarev, Kanygina, Pang and Lee2022; Kushnareva et al. Reference Kushnareva, Khudoley, Alexeiev and Petrov2022). HP–UHP rocks corresponding to variously retrograded eclogites and garnet-bearing quartz–feldspar gneisses (± coesite) are also common occurrences in these terranes and are interpreted to have originated from the subduction of Precambrian continental crust in the early Palaeozoic (509–474 Ma) during the closure stages of the Palaeo-Asian Ocean (e.g. Alexeiev et al.. Reference Alexeiev, Ryazantsev, Kröner, Tretyakov, Xia and Liu2011; Kröner et al.. Reference Kröner, Alexeiev, Hegner, Rojas-Agramonte, Corsini, Chao, Wong, Windley, Liu and Tretyakov2012; Rojas-Agramonte et al. Reference Rojas-Agramonte, Herwartz, García-Casco, Kröner, Alexeiev, Klemd, Buhre and Barth2013; Klemd et al. Reference Klemd, Hegner, Bergmann, Pfander, Li and Hentschel2014; Konopelko & Klemd, Reference Konopelko and Klemd2016; Pilitsyna et al. Reference Pilitsyna, Tretyakov, Degtyarev, Cuthbert, Batanova and Kovalchuk2018a, Reference Pilitsyna, Tretyakov, Degtyarev, Salnikova, Kotov, Kovach, Wang, Batanova, Plotkina, Tolmacheva, Ermolaev and Lee2019). The HP–UHP rock assemblages were then exhumed from different structural depths, forming tectonic slices of various metamorphosed formations. Within the study area, these HP–UHP rocks are assigned to the Makbal (Issyk-Kul terrane), Aktyuz (Chu-Kendyktas terrane) and Koyandy complexes (Figure 1(b)). The formation of these complexes is thought to have been related to the evolution of the early Palaeozoic Dzhalair-Naiman and Kara-Archa ophiolite zones.
2.1. Makbal ultrahigh-pressure complex (Issyk-Kul terrane)
The UHP formations within the Issyk-Kul terrane in the North Tien Shan are represented by eclogites and amphibolites (formed after eclogites), as well as coesite-bearing garnet–chloritoid–talc schists and coesite-bearing garnet quartzites of the Makbal complex. The UHP rocks of the Makbal complex are juxtaposed against quartzites and mica schists of lower grades. The protoliths of the eclogites are considered either palaeo-oceanic crust or mafic dikes emplaced into continental crust prior to UHP metamorphism during the final stages of Rodinia breakup (Meyer et al. Reference Meyer, Klemd, Hegner and Konopelko2014; Rojas-Agramonte et al. Reference Rojas-Agramonte, Herwartz, García-Casco, Kröner, Alexeiev, Klemd, Buhre and Barth2013). The timing of the near-peak-stage metamorphism of the eclogites has been generally interpreted as ∼509–498 Ma based on U–Pb dating of zircon rims (Konopelko et al. Reference Konopelko, Kullerud, Apayarov, Sakiev, Baruleva, Ravna and Lepekhina2012), although a Lu–Hf garnet-whole rock isochron yields a younger age of ca. 470 Ma for this HP metamorphism (Rojas-Agramonte et al. Reference Rojas-Agramonte, Herwartz, García-Casco, Kröner, Alexeiev, Klemd, Buhre and Barth2013). Some of the crustal rocks of the Makbal complex have experienced UHP re-equilibration at >24 kbar (Tagiri & Bakirov, 1990; Meyer et al. Reference Meyer, Klemd, Hegner and Konopelko2014). Zircon rims from the garnet–chloritoid–talc schists have yielded a U–Pb mean age of ca. 502 Ma for the UHP event, whereas zircon core ages cover a wide range from 2,583 to 642 Ma. This wide age span may indicate the sedimentary origin of the protolith rocks (Konopelko et al. Reference Konopelko, Kullerud, Apayarov, Sakiev, Baruleva, Ravna and Lepekhina2012, Konopelko & Klemd, Reference Konopelko and Klemd2016). The negative εNd = −11 values (Meyer et al. Reference Meyer, Klemd, Hegner and Konopelko2014) of the garnet–chloritoid–talc schists suggest that ancient crustal material was an essential component of their protoliths. Protoliths of coesite-bearing garnet quartzites of the Makbal complex were similarly made of terrigenous strata, the accumulation of which occurred in a passive margin setting (Togonbaeva et al. Reference Togonbaeva, Takasu, Bakirov, Sakurai, Tagiri, Bakirov and Sakiev2009; Konopelko & Klemd, Reference Konopelko and Klemd2016). Neoarchean and Palaeoproterozoic crustal material (2.7–1.6 Ga) was the main source of the protoliths of quartzites (Degtyarev et al. Reference Degtyarev, Ryazantsev, Tretyakov, Tolmacheva, Yakubchuk, Kotov, Salnikova and Kovach2013; Konopelko & Klemd, Reference Konopelko and Klemd2016; Alexeiev et al. Reference Alexeiev, Khudoley and DuFrane2020).
2.2. Aktyuz high-pressure complex (Chu-Kendyktas terrane)
Late Tonian medium-grade gneissic granites and orthogneisses (∼840–780 Ma) and variously altered Cambrian paragneisses with low-grade mica schists make up much of the Aktyuz, Kemin and Kokdzhon complexes in the SE part of the Chu-Kendyktas terrane in the North Tien Shan (Figure 2) (Kröner et al. Reference Kröner, Alexeiev, Hegner, Rojas-Agramonte, Corsini, Chao, Wong, Windley, Liu and Tretyakov2012; Rojas-Agramonte et al. Reference Rojas-Agramonte, Kröner, Alexeiev, Jeffreys, Khudoley, Wong, Geng, Shu, Semiletkin, Mikolaichuk, Kiselev, Yang and Seltmann2014; Skoblenko et al. Reference Skoblenko, Kanygina, Tretyakov, Degtyarev, Nguyen, Pang, Sheshukov and Erofeeva2023). Magmas of the Neoproterozoic granitoids were derived from partial melting of early Precambrian crustal material with model Nd ages of 2.1–1.5 Ga (Kröner et al. Reference Kröner, Alexeiev, Hegner, Rojas-Agramonte, Corsini, Chao, Wong, Windley, Liu and Tretyakov2012). These Neoproterozoic granitoids constitute the basement of the Chu-Kendyktas terrane. The Aktyuz complex includes eclogite that is spatially associated with quartz–feldspar garnet–mica gneisses and their lower-grade derivatives. Lu–Hf garnet isochron dating of the amphibolised eclogite yields an age of ca. 474 Ma, which has been interpreted as the timing of their near-peak stage of HP metamorphism. The protolith of this eclogitic rock was rifting-derived mafic dikes that intruded into the continental crust sequence prior to the late Cambrian-Early Ordovician subduction stage (Rojas-Agramonte et al. Reference Rojas-Agramonte, Kröner, Alexeiev, Jeffreys, Khudoley, Wong, Geng, Shu, Semiletkin, Mikolaichuk, Kiselev, Yang and Seltmann2014; Klemd et al. Reference Klemd, Hegner, Bergmann, Pfander, Li and Hentschel2014). On the other hand, zircons separated from the associated HP garnet–mica gneisses have yielded two age clusters, corresponding to the timing of the HP re-equilibration at ca. 490 Ma and the protolith age of ca. 844 Ma known from the inherited zircon cores (Skoblenko et al. Reference Skoblenko, Kanygina, Tretyakov, Degtyarev, Nguyen, Pang, Sheshukov and Erofeeva2023). Detrital zircon populations from the paragneisses and schists of the Kokdzhon and Kemin complexes of the Chu-Kendyktas terrane show wide age ranges for the cores (at 600, 800 and 1000 Ma and less than 1.5 and 2.5 Ga) and varisized rims aged 495–471 Ma, compatible with the near-peak to retrograde metamorphic evolution of the metasedimentary rocks (Skoblenko et al. Reference Skoblenko, Kanygina, Tretyakov, Degtyarev, Nguyen, Pang, Sheshukov and Erofeeva2023).
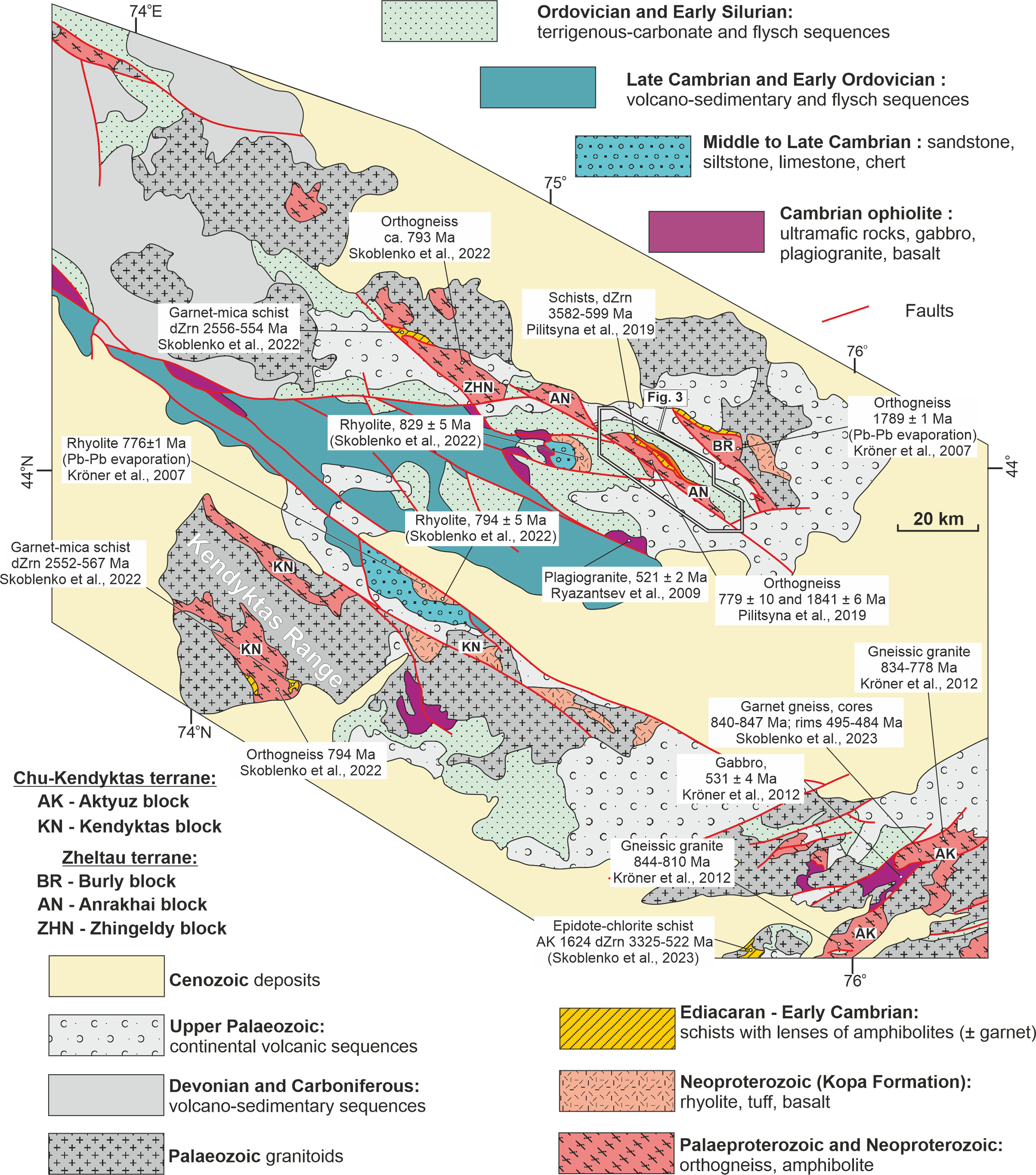
Figure 2. Geological map of the SE part of the Chu-Kendyktas (North Tien Shan) and Zheltau (South Kazakhstan) terranes. Age estimates for the principal rock types are after Kröner et al. (Reference Kröner, Windley, Badarch, Tomurtogoo, Hegner, Jahn, Gruschka, Khain, Demoux and Wingate2007, Reference Kröner, Alexeiev, Hegner, Rojas-Agramonte, Corsini, Chao, Wong, Windley, Liu and Tretyakov2012), Ryazantsev et al. (Reference Ryazantsev, Mikolaychuk, Tolmacheva, Degtyarev, Kotov, Nikitina, Mamonov and Zorin2009), Pilitsyna et al. (Reference Pilitsyna, Tretyakov, Degtyarev, Salnikova, Kotov, Kovach, Wang, Batanova, Plotkina, Tolmacheva, Ermolaev and Lee2019) and Skoblenko et al. (Reference Skoblenko, Degtyarev, Kanygina, Pang and Lee2022, Reference Skoblenko, Kanygina, Tretyakov, Degtyarev, Nguyen, Pang, Sheshukov and Erofeeva2023).
2.3. Koyandy high-pressure complex (Zheltau terrane)
Prevailing metamorphic formations in the basement of the Zheltau terrane in South Kazakhstan consist mainly of Palaeoproterozoic (ca. 1840 Ma) and Neoproterozoic (ca. 790 Ma) orthogneisses with amphibolites (± garnet) derived from early Precambrian crustal sources with estimated Nd model ages of 2.3–2.1 Ga (Pilitsyna et al. Reference Pilitsyna, Tretyakov, Degtyarev, Salnikova, Kotov, Kovach, Wang, Batanova, Plotkina, Tolmacheva, Ermolaev and Lee2019; Skoblenko et al. Reference Skoblenko, Degtyarev, Kanygina, Pang and Lee2022) (Figure 2). Ediacaran–Cambrian metasedimentary formations take up much less space in the Koyandy complex and are composed mainly of low-grade two-mica or chloritized schists, quartzites and marbles, associated with variously retrograded kyanite-bearing garnet–mica gneisses and schists (Figure 3). The latter commonly contains tectonic lenses and boudins of eclogites intercalated with garnet pyroxenites, garnet amphibolites formed after eclogites and rare serpentinized symplectite-bearing peridotites, which record HP near-peak stages of at least P = 16-18 kbar during their evolution (Pilitsyna et al. Reference Pilitsyna, Tretyakov, Degtyarev, Cuthbert, Batanova and Kovalchuk2018a, b). A U–Pb zircon age of ca. 490 Ma was obtained from the garnet pyroxenites of the Koyandy complex (Alexeiev et al. Reference Alexeiev, Ryazantsev, Kröner, Tretyakov, Xia and Liu2011), corresponding to the timing of their HP metamorphism. In turn, the protoliths of the eclogites and garnet pyroxenites are interpreted to have been derivatives of intraplate mafic melts that intruded into continental crust before the onset of subduction (Pilitsyna et al. Reference Pilitsyna, Tretyakov, Degtyarev, Cuthbert, Batanova and Kovalchuk2018a). Protoliths of metasedimentary garnet–mica schists exposed in the central part of the Anrakhai Block (Figure 3) are terrigenous rocks with main age peaks at ∼991 Ma and ∼1082 Ma and, to a lesser extent, 1.6–1.8 Ga and ∼2.5 Ga (Pilitsyna et al. Reference Pilitsyna, Tretyakov, Degtyarev, Salnikova, Kotov, Kovach, Wang, Batanova, Plotkina, Tolmacheva, Ermolaev and Lee2019). One of the detrital zircon grain rims obtained from these schists revealed an age of ∼486 Ma from its rims, indicating that the protoliths of these rocks might have experienced subduction zone processes.

Figure 3. Geological map of the Anrakhai Block (Zheltau terrane). Age estimates for the principal rock types of the Anrakhai and Koyandy metamorphic complexes are after Alexeiev et al. (Reference Alexeiev, Ryazantsev, Kröner, Tretyakov, Xia and Liu2011) and Pilitsyna et al. (Reference Pilitsyna, Tretyakov, Degtyarev, Salnikova, Kotov, Kovach, Wang, Batanova, Plotkina, Tolmacheva, Ermolaev and Lee2019).
In the SE part of the Anrakhai Block of the Zheltau terrane (Figure 3(a)), quartz–feldspar migmatized gneisses (Figure 3(b)) are spatially associated with metre-scale eclogitic boudins (Figure 3(c)). One of these migmatitic gneiss exposures contains distinctive bands of melanosomes composed of garnet–mica gneisses with kyanite and leucosome bands of weakly deformed granitoid gneisses (Figure 3(d)–(e)). Exposures of strongly foliated, coarse-grained garnet–kyanite schists (retrograded paragneisses) crop out in the vicinity of migmatitic gneiss with eclogites (Figure 4). These rocks have been interpreted to represent HP felsic granulites formed at a near-peak stage of metamorphism at pressures of 15–18 kbar and temperatures of 750–850°C (Pilitsyna et al. Reference Pilitsyna, Tretyakov, Degtyarev, Salnikova, Kotov, Kovach, Wang, Batanova, Plotkina, Tolmacheva, Ermolaev and Lee2019).

Figure 4. Scanned photograph of a polished sample of garnet–kyanite schist (retrograded paragneiss) AN1803 from the Koyandy complex (Zheltau terrane).
3. Methods
3.1. Whole-rock and mineral chemistry analyses
X-ray fluorescence (XRF) was used to determine the major elements using a Bruker AXS wavelength dispersive S4 PIONEER spectrometer with a 4-kW X-ray tube at the Geological Institute of the Russian Academy of Sciences (RAS). The Spectra-Plus software package was used for data processing. The automatic registration of peak overlaps and correction for matrix effects were performed during individual measurements of fundamental parameters for each sample. The intervals of analyzed concentrations obtained after re-calculation to oxides (mass fraction %) were as follows: Si = 0.01–1.0, Ti = 0.01–5.0, Al = 1.0–60.0, Fe (total) = 1.0–40.0, Mn = 0.01–1.0, Ca = 1.0–50, Mg = 0.1–40, Na = 0.1–10.0, K = 0.1–10.0 and P = 0.01–5.0. The contents of FeO were determined by wet chemistry via titration with potassium dichromate in sulphuric acid medium after acid decomposition of the sample in the absence of oxygen at the Geological Institute RAS. To obtain the contents of Fe(III) in the form of Fe2O3, the XRF data was corrected for the amount of iron (II) oxide obtained from the results of titration.
Whole-rock trace element analysis was performed at the Analytical Centre of the Institute of Microelectronics Technology, Moscow, and High-Purity Materials, RAS, using a PerkinElmer ELAN 6100 DRC inductively coupled plasma mass spectrometer (ICP-MS) and atomic emission spectrometry with an ICP-MS (ICAP-61, Thermo Jarrell Ash; X-7, Thermo Elemental, USA). The relative standard deviations for all analyzed elements were not more than 0.2 for element contents below the five-fold detection limit and below 0.1 for contents above this detection limit. The major elements and trace elements concentrations are presented in wt.% and ppm, respectively.
Mineral compositions and element maps were obtained using JEOL JXA-8230 and JXA-iHP200F EPMA at the Institut des Sciences de La Terre (ISTerre, University Grenoble Alpes, France), equipped with five wavelength dispersive spectrometers and an energy dispersive (EDX) spectrometer operating at 20 kV accelerating voltage, 20 nA probe current and 1 μm beam diameter. Mineral compositions of the sample AK1904 were obtained using a JSM-6510 scanning electron microscope with an EDX INCA Energy-350 (Oxford Instruments) and MIRA 3 (TESCAN, Czech Republic) at the Analytical Center of Geological Institute RAS.
Fe3+ was recalculated for garnet based on charge balance and stoichiometry. Mineral abbreviations are after Whitney & Evans (Reference Whitney and Evans2010); a.p.f.u. is atom per formula unit.
3.2. SHRIMP II U–Pb isotope analyses
A SHRIMP II ion probe was used for zircon dating at the Center for Isotopic Research at the Karpinsky Russian Geological Research Institute (Saint Petersburg, Russia) following conventional techniques (Williams, Reference Williams1998; Larionov et al. Reference Larionov, Andreichev, Gee, Gee and Pease2004). The zircons were mounted in epoxy along with the zircon standards 91500 (Wiedenbeck et al. Reference Wiedenbeck, Alle, Corfu, Griffin, Meier, Oberli, von Quadt, Roddick and Spiegel1995) and Temora (Black et al. Reference Black, Kamo, Allen, Aleinikoff, Davis, Korsch and Foudoulis2003), polished to approximately half of the grain thickness and coated with 99.999% pure gold. Optical microscopy and cathodoluminescence were performed on euhedral crystals selecting the regions devoid of visible cracks and inclusions to investigate the inner structure of the zircons. Data were processed with SQUID v1.12 and ISOPLOT/Ex 3.22 (Ludwig, Reference Ludwig2005a, b) using the decay constants of Steiger & Jäger (Reference Steiger and Jäger1976). Common Pb correction was applied based on the model by Stacey & Kramers (Reference Stacey and Kramers1975) using the measured 204Pb/206Pb ratio.
3.3. LA-ICP-MS U-Pb isotope analyses
U-Th-Pb zircon dating by LA-ICP-MS was carried out at the Geological Institute of RAS employing a sector field – inductively coupled plasma – mass spectrometer (SF-ICP-MS) Thermo-Scientific Element2 coupled to Electro Scientific NWR-213 laser ablation system (Sheshukov et al. Reference Sheshukov, Kuzmichev, Dubenskiy, Okina, Degtyarev, Kanygina, Kuznetsov, Romanjuk and Lyapunov2018). Helium gas was used as the laser ablation carrier with further admixture of argon. Precise filtration and gas mixing were used to reduce gas noise and increase the stability of the analytical signal. The zircon reference material GJ-1 (Jackson et al. Reference Jackson, Pearson, Griffin and Belousova2004; Elhlou et al. Reference Elhlou, Belousova, Griffin, Pearson and Riley2006) with accepted CA-ID-TIMS 206Pb/238U age of 601.9 ± 0.4 Ma (Horstwood et al. Reference Horstwood, Kosler, Gehrels, Jackson, McLean, Paton, Pearson, Sircombe, Sylvester, Vermeesch, Bowring, Condon and Schoene2016) was used as a primary standard for external calibration procedure, provided by the Australian Research Council (ARC) National Key Centre for Geochemical Evolution and Metallogeny of Continents, Macquarie University (Sydney, Australia). For accuracy control, there were used 91500 (Wiedenbeck et al. Reference Wiedenbeck, Alle, Corfu, Griffin, Meier, Oberli, von Quadt, Roddick and Spiegel1995, Reference Wiedenbeck, Hanchar, Peck, Sylvester, Valley, Whitehouse, Kronz, Morishita, Nasdala, Fiebig, Franchi, Girard, Greenwood, Hinton, Kita, Mason, Norman, Ogasawara, Piccoli, Rhede, Satoh, Schulz-Dobrick, Skår, Spicuzza, Terada, Tindle, Togashi, Vennemann, Xie and Zheng2004) and Plesovice (Sláma et al. Reference Sláma, Kosler, Condon, Crowley, Gerdes, Hanchar, Horstwood, Morris, Nasdala, Norberg, Schaltegger, Schoene, Tubrett and Whitehouse2008) zircon references with accepted CA-ID-TIMS 206Pb/238U ages 1063.5 ± 0.4 Ma and 337.2 ± 0.1 Ma, respectively (Horstwood et al. Reference Horstwood, Kosler, Gehrels, Jackson, McLean, Paton, Pearson, Sircombe, Sylvester, Vermeesch, Bowring, Condon and Schoene2016). Glitter 4.4 software (Van Achterbergh et al. Reference Van Achterbergh, Ryan, Jackson, Griffin and Sylvester2001) was used for data reduction and common lead correction was carried out using the T. Andersen procedure (Andersen, Reference Andersen2002) – ComPbCorr 3.18 software (Andersen, Reference Andersen and Sylvester2008). The Isoplot 4.15 software (Ludwig, Reference Ludwig2008) and Isoplot R (Vermeesch, Reference Vermeesch2018) were used for graphic illustration of the results.
3.4. Sm-Nd isotope data
The Sm-Nd isotope research was carried out in the Collective Use Centre of the Kola Science Centre RAS (Apatity, Russia). The isotope Nd composition and Sm and Nd contents were measured by a 7-channel solid-phase mass-spectrometer, Finnigan-MAT 262, equipped with a Retarding Potential Quadrupole in a static double-band mode, using Ta + Re filaments. A mean value of 143Nd/144Nd ratio in a JNdi-1 standard was 0.512311 ± 7 (N = 5) in the test period. An error in 147Sm/144Nd in ratios was 0.3% (2σ), which is the mean value of seven measurements in a BCR-2 standard (Raczek et al. Reference Raczek, Jochum and Hofmann2003). An error in the estimation of isotope Nd composition in an individual analysis was up to 0.004%. The blank intralaboratory contamination was 0.3 ng in Nd and 0.06 ng in Sm. The accuracy of the estimation of Sm and Nd contents was ±0.5%. Isotope ratios were normalized per 146Nd/144Nd = 0.7219, and then recalculated for 143Nd/144Nd in JNdi-1 = 0.512115 (Tanaka et al. Reference Tanaka, Togashi, Kamioka, Amakawa, Kagami, Hamamoto, Yuhara, Orihashi, Yoneda, Shimizu, Kunimaru, Takahashi, Yanagi, Nakano, Fujimaki, Shinjo, Asahara, Tanimizu and Dragusanu2000). Values of εNd(T) and T(DM) model ages were estimated using present-day values of CHUR as described in (Bouvier et al. Reference Bouvier, Vervoort and Patchett2008) (143Nd/144Nd = 0.512630, 147Sm/144Nd = 0.1960) and DM as described in (Goldstein & Jacobsen, Reference Goldstein and Jacobsen1988) (143Nd/144Nd = 0.513151, 147Sm/144Nd = 0.2136).
4. Results
4.1. Petrography, whole-rock and mineral chemistry of high-pressure metasedimentary rocks of the Koyandy complex
Metasedimentary rocks of the Koyandy complex in the Zheltau terrane consist of variously altered paragneisses and schists with different proportions of garnet, micas and feldspars relative to quartz. In the NW part of the Anrakhai Block (Figure 3(a)), mica-rich garnet schists form a narrow, 50–100 m-thick zone, whereas migmatized kyanite-bearing quartz–feldspar paragneisses and schists that are spatially associated with low-grade mica schists occur in the SE part of this block. The micaceous high-grade metasedimentary rocks contain pods and boudins of eclogites and garnet amphibolites formed after eclogites (Figure 3(c)), suggesting that HP rocks and metasedimentary rocks might have formed concurrently.
4.1.1. Migmatized garnet–mica paragneisses
These rocks contain intercalating bands of garnet–mica–feldspar–quartz±kyanite (melanosome) and granitoid leucosomes composed of feldspars, quartz and white mica (Figure 3(d)). The leucosomes also form injections and layers in the bands of dark garnet–mica gneisses (Figure 3(e)). Garnets in melanosome bands display euhedral or subhedral habits with partially preserved facets up to 0.5 mm in size (Figure 5(b) and (c); Figure 6(a)) and commonly contain inclusions of quartz, rutile and kyanite near their margins (Figure 5(a); Table S4). Garnets are characterized by pronounced chemical zoning with Mn-rich cores (Xmn = 0.25) and Ca-rich rims with Mg-rich rims (Xca and Xmg correspond to 0.16 compared to Xca = 0.09 and Xmg = 0.15 in the core) (Figure 6(a); Table S1). These zones show irregular diffusional boundaries (Figure 6(a)), which might indicate high-temperature modifications under increased P–T conditions. Feldspars of the melanosome matrix include plagioclase (oligoclase–andesine) and potassium feldspar (Table S2; Figure 5(e) and (f)), and together with quartz (up to 0.1 mm thick), they form ribbons in the direction of lineation in paragneisses (Figure 5(b)). Biotite and white mica grains are subparallel to lineation and are abundant in melanosomes in nearly equal proportions. Muscovite makes up elongated, 0.5–1.5-mm-long prismatic grains (Figure 5(b), (e), (f)), whereas biotite appears as prismatic or anhedral, <0.2-mm-long grains (Figure 5(a) and (c)). White mica corresponds to phengite (Siapfu = 3.32–3.37) or later phengite–muscovite (Siapfu = 3.01–3.15) (Table S3) and locally encloses garnet inclusions (Figure 5(c)). In turn, leucosome granitoids are nearly free of dark-coloured minerals and exhibit gneissic textures with oriented subhedral grains of potassium feldspar and 0.05–0.8-mm-long plagioclase (albite) grains spatially associated with quartz (Figure 5(d); Table S2). Phengite (Siapfu = 3.2) comprises varisized, unevenly distributed grains elongated in the same direction as feldspars (Figure 5(d); Table S3). Both garnet–mica paragneisses and leucosome granitoids contain numerous zircon grains, rutile and titanite.

Figure 5. Photomicrographs and element maps of the main mineral phases and microtextures of a migmatized garnet–mica paragneiss with kyanite (garnet–mica–feldspar–quartz–kyanite melanosome AN1801/1 (a)–(c); (e), (f) and granitoid leucosome AN1801/2 (d), garnet–kyanite schist (retrograded paragneiss) AN1803 (g)–(i): (a) garnet and kyanite bounded by micas (Al element map); (b) porphyroblasts of garnet with white micas within the quartz–biotite–feldspar matrix (crossed nicols); (c) relict garnet enclosed by a coarse grain of white mica in the quartz–biotite–feldspar matrix (plane light); (d) quartz–feldspar matrix with porphyroblasts of white mica, plagioclase and K-feldspar (crossed nicols); (e), (f) association of white mica, plagioclase and K-feldspar (K and Al element maps); (g) coarse porphyroblasts of kyanite and rutile in the quartz–feldspar matrix (crossed nicols); (h) coarse porphyroblasts of garnet in the quartz–feldspar matrix (plane light); zoned porphyroblast of garnet (Ca element map). Key for the abbreviations: Bt – biotite; WM – white mica; Grt – garnet; Kfs – K-feldspar; Pl – plagioclase; Qz – quartz; Rt – rutile; Ky – kyanite.

Figure 6. Element maps of garnets (Mn, Ca, Mg and Fe distributions) with pronounced zoning: Left: garnet–mica–feldspar–quartz–kyanite melanosome AN1801/1. Right: garnet–kyanite schist (retrograded paragneiss) Z12375 (Pilitsyna et al. Reference Pilitsyna, Tretyakov, Degtyarev, Salnikova, Kotov, Kovach, Wang, Batanova, Plotkina, Tolmacheva, Ermolaev and Lee2019; completely analogous to sample AN1803). Consistent with Table S1.
In terms of the whole-rock composition, the garnet–mica paragneiss of the melanosome (sample AN1801/1) is characterized by significant contents of MgO (2.07 wt.%), FeOtot (8.43 wt.%) and TiO2 (1.4 wt.%) with 64.39 wt.% SiO2 (Table 1), reflecting the existence of high modal contents of garnet and biotite in the rocks. This finding is also consistent with the notable concentrations of trace elements, such as Co, Sc and V (Table 1). In contrast, leucosome granitoids (samples AN1801/2, AN1802 and TS11100) have low concentrations of MgO (0.08–0.76 wt.%) and FeOtot (0.99–2.29 wt.%), along with high contents of ΣNa2O+K2O of 6.51–8.02 wt.% and SiO2 (73.85–78.19 wt.%), consistent with a predominance of feldspars and quartz among the main rock-forming minerals (Table 1). All the analyzed samples show differentiated REE spectra with enrichments in LREEs and depletions in HREEs and display negative Eu anomalies of ∼0.6 (Figure 7), compatible with a granitoid source of their protoliths. Notably, sample AN1801/2 of the granitoid leucosome (Figure 3(d) and 7) exhibits a strongly negative Eu anomaly with Eu/Eu* = 0.15, which might be indicative of a highly evolved melt and relatively earlier crystallization of plagioclase.
Table 1. Selected chemical analyses of the key rock types from the Koyandy complex (Zheltau terrane) and Aktyuz with Kemin complexes (adjacent Chu-Kendyktas terrane; Skoblenko et al. Reference Skoblenko, Kanygina, Tretyakov, Degtyarev, Nguyen, Pang, Sheshukov and Erofeeva2023) for comparison. Major elements are in wt.%, and trace elements are in ppm. FeO(tot) = 0.9Fe2O3 + FeO; n.d. is ‘not determined’; LOI is ‘loss on ignition’. Chemical compositions of the gneisses of the Aktyuz complex are taken from Skoblenko et al. (Reference Skoblenko, Kanygina, Tretyakov, Degtyarev, Nguyen, Pang, Sheshukov and Erofeeva2023); chemical compositions of samples AN1470 and AN1320 are from Pilitsyna et al. (Reference Pilitsyna, Tretyakov, Degtyarev, Salnikova, Kotov, Kovach, Wang, Batanova, Plotkina, Tolmacheva, Ermolaev and Lee2019)

4.1.2. Garnet–kyanite schists (retrograded paragneisses)
These are intensively foliated coarse-grained rocks, in which 3-5-mm-long garnet porphyroblasts and 0.5-0.8-mm-long kyanite occur in the matrix (Figure 4, 5(g), (h)). Both potassium feldspar and plagioclase (albite–oligoclase) grains are preferentially oriented; mesoperthite of Ab50Or50 has also been identified (Table S2). Quartz dominates among the light-coloured minerals and forms anhedral grains (0.05–0.5 mm in size), showing irregular edges and undulating extinction (Figure 5(g)). Micas constitute ∼15 vol.% of the rock and are largely represented by phengite–muscovite (Siapfu = 3.1; Table S3) with minor biotite (Figure 5(h); Table S3). White mica generally forms aggregates of fine-grained flakes in the matrix, but it also locally replaces kyanite (Figure 5(g)). Coarse porphyroblasts of Fe-Mg garnet demonstrate pronounced chemical zoning (Figure 6(b)), in which near-peak and postpeak stages of garnet growth are identified. We observed smaller garnets with mostly homogenized interiors and analyzed them (Table S1). These garnets appear to have been more intensively modified by high-temperature diffusion processes, which nearly obliterated their primary growth zoning (Carlson, Reference Carlson2006). The only exception is calcium distribution, displaying a clear decrease from the grain cores (Xca = 0.14) to the rims (Xca = 0.10) (Figure 5(i); Table S1), reflected by a slower Ca diffusion rate compared to Fe and Mg (Vielzeuf et al.. Reference Vielzeuf, Baronnet, Perchuk, Laporte and Baker2007). Garnet–kyanite schists often include coarse grains of rutile (up to 1 mm in length; Figure 5(g)), varisized titanite, monazite and apatite with exsolution lamellae of monazite, as well as voluminous zircons.
The rocks (e.g. sample AN1803) possess SiO2 contents of 74.79 wt.% and moderate-to-high contents of MgO and FeOtot of 1.81 and 5.12 wt.%, respectively (Table 1), and demonstrate an enrichment in LREEs relative to HREEs, with a negative Eu anomaly of 0.63 (Figure 7). This finding is consistent with the element distributions obtained for the migmatized garnet–mica paragneisses. Chemical compositions and chondrite-normalized patterns of REE distribution of the studied garnet–kyanite schists are comparable to those of the granitoids, suggesting a predominance of felsic rocks in the source of their protoliths.
4.1.3. Garnet–mica schists (retrograded paragneisses) with relics of kyanite
Garnet–mica schists are the most widespread among the micaceous metasedimentary lithologies of the Koyandy complex. As described in detail in Pilitsyna et al. (Reference Pilitsyna, Tretyakov, Degtyarev, Salnikova, Kotov, Kovach, Wang, Batanova, Plotkina, Tolmacheva, Ermolaev and Lee2019), these rocks contain abundant micas (biotite and muscovite), partly resorbed garnet and plagioclase with quartz (samples AN1470, AN2201/1; Table 1). This assemblage is considered to have grown during retrogression under amphibolite facies conditions. Kyanite is only locally preserved as inclusions in garnets.
Low-grade mica schists consist of various proportions of micas and chlorite along with plagioclase and quartz (without garnet) and correspond to epidote–amphibolite facies metamorphism at the peak. However, they are juxtaposed against the high-grade paragneisses, and collectively, they all represent derivatives of terrigenous protoliths formed after erosion of granitoids, as inferred from their chemical compositions and εNd(t) characteristics (Figure 7; Table 1) (Pilitsyna et al. Reference Pilitsyna, Tretyakov, Degtyarev, Salnikova, Kotov, Kovach, Wang, Batanova, Plotkina, Tolmacheva, Ermolaev and Lee2019).
4.2. Phase equilibria modelling of the high-pressure metasedimentary rocks of the Koyandy complex
We used the Perple_X software package (version 6.9.1; Connolly, Reference Connolly1990, Reference Connolly2005) in conjunction with the internally consistent thermodynamic database of Holland & Powell (Reference Holland and Powell2011) to construct P–T pseudosections for the migmatized garnet–mica paragneiss with kyanite AN1801 and garnet–kyanite schist (retrograded paragneiss) AN1803 (Koyandy complex, Zheltau terrane) (Figure 8 and 9; Table 1). A P–T pseudosection was also constructed for the garnet–mica gneiss AK1904 (Aktyuz complex, Chu-Kendyktas terrane) sample for comparison (Figure S1; Table 1).

Figure 8. P–T pseudosections with the estimated prograde (a) and postpeak to retrograde (b) stages of metamorphism for migmatized garnet–mica paragneiss with kyanite AN1801 in the NKCTiFMMnASH system, based upon the reintegrated bulk composition (given in mol.%). The details of recalculations and implemented solution models are given in Section 4.2. Coloured lines are compositional isopleths for Xmn, Xca and Xmg in garnet (Table S1) and Si (a.p.f.u.) in white mica (Table S3).

Figure 9. P–T pseudosections with the estimated prograde (a) and near-peak to retrograde (b) stages of metamorphism for garnet–kyanite schist (retrograded paragneiss) AN1803 in the NKCTiFMMnASH system based upon measured bulk compositions (given in mol.%). The details of recalculations and implemented solution models are given in Section 4.2. Coloured lines are compositional isopleths for Xfe, Xca and Xmg in garnet (Table S1).
Assuming that migmatization of the protolith of paragneisses occurred under suprasolidus conditions, which led to the formation of garnet-rich melanosome AN1801/1 and granitoid leucosome AN1801/2 (Figure 3), we used the reintegrated averaged whole-rock composition to constrain the approximate prograde, near-peak and retrograde stages of the metamorphic evolution of the rocks (Figure 8(a) and (b)). Garnet–kyanite gneiss sample AN1803 is a complete equivalent of sample Z12375, as considered in Pilitsyna et al. (Reference Pilitsyna, Tretyakov, Degtyarev, Salnikova, Kotov, Kovach, Wang, Batanova, Plotkina, Tolmacheva, Ermolaev and Lee2019). However, additional calculations were conducted in the present study to assess the retrograde stage evolution of the rocks by using the whole-rock compositions (Figure 9(a) and (b)).
Phase diagrams for the indicated metasedimentary rocks were constructed using the Na2O–K2O–CaO–TiO2–FeO––MgO–MnO–Al2O3–SiO2–H2O model system (NKCTiFMMnASH), which was calculated assuming the saturation of an H2O fluid (the CORK equation of state; Holland & Powell, Reference Holland and Powell1998). Assuming an anatexis of metapelites, H2O was specified as a system component consistent with loss on ignition values. The pseudosections were calculated with the solution models of Fsp(C1) for feldspars (Holland & Powell, Reference Holland and Powell2003), Gt(HP) for garnet (Holland & Powell, Reference Holland and Powell1998), Mica(CHA) for white mica (Coggon & Holland, Reference Coggon and Holland2002; Auzanneau et al. Reference Auzanneau, Schmidt, Vielzeuf and Connolly2010), Bio(HP) for biotite (White et al. Reference White, Powell and Holland2007) and melt(HP) for haplogranitic melt (Holland & Powell, Reference Holland and Powell2001; White et al. Reference White, Powell and Holland2001). Mineral assemblages of the paragneisses contain negligible contents of Fe3+; therefore, total iron was introduced in the calculations. Implemented whole-rock compositions are provided in mol.%, and garnet compositional isopleths are introduced into pseudosections for more accurate P–T path estimations. Garnet compositions are provided in Table S1.
4.2.1. Migmatized garnet–mica paragneiss
For sample AN1801, the wet solidus at pressures of 8–15 kbar corresponds to T = ∼ 680–690°C (Figure 8(a)), which indicates the onset of anatexis through dehydration melting of micas and water flux melting at T > 690°C. The best reproducibility of Xmn, Xca and Xmg compositional isopleths for garnet cores (Table S1) corresponds to pressures of 9–12 kbar and temperatures of 720–760°C that are interpreted as the parameters of suprasolidus garnet growth at prograde stages under increasing P–T and subsequent melting propagation conditions. The appearance of kyanite as inclusions in the marginal parts of garnet grains indicates a breakdown of white mica through the reaction of Ph + Pl + Qz = Grt + Ky + Kfs + melt (Indares & Dunning, Reference Indares and Dunning2001) at P > 18.5 kbar and T>870°C (Figure 8(b)). The following evolution of the rocks is related to retrograde growth of phengite–muscovite that is reflected by the intersection of Xmn and Xca compositional isopleths of garnet rims (Table S1) and Siapfu isopleths of 3.14–3.15 for phengite (Table S3) in a temperature range of 870 to 800°C at pressures of 18.5–12 kbar (Figure 8(b)). The subsequent extensive growth of biotite and muscovite associated with garnet resorption is consistent with complete melt crystallization at P < 12 kbar and T < 720°C. In doing so, Zr-in-rutile thermometry yields a range of temperatures corresponding to ∼650–690°C (Table S5), compatible with the near-suprasolidus conditions of the formation of rutile.
4.2.2. Garnet–kyanite schist (retrograded paragneiss)
Mineral assemblages and garnet zoning in sample AN1803 (equivalent to sample Z12375 analyzed in Pilitsyna et al. Reference Pilitsyna, Tretyakov, Degtyarev, Salnikova, Kotov, Kovach, Wang, Batanova, Plotkina, Tolmacheva, Ermolaev and Lee2019) reflect several stages in the evolution of the rocks at prograde, near-peak, postpeak and late retrograde stages. Coarse garnet grains (Figure 6(b)) record prograde P–T path parameters at 8–9.5 kbar and 710–720°C (stage I) observed from the intersection of Xfe, Xca and Xmg isopleths (Figure 9(a); Table S1). The isopleth intersection is in the vicinity of the solidus line, which might indicate the formation of garnet cores under suprasolidus conditions. Near-peak conditions of the evolution of the rocks are defined as P = 15–17 kbar and T = 760–810°C (stage II, obtained by Pilitsyna et al. Reference Pilitsyna, Tretyakov, Degtyarev, Salnikova, Kotov, Kovach, Wang, Batanova, Plotkina, Tolmacheva, Ermolaev and Lee2019), with the best reproducibility of XMn, XCa, XFe and XMg compositional isopleths corresponding to mantle-inner rim parts of garnet (Figure 9(a); Table S1). The transition to the near-peak stage is determined by the disappearance of biotite to produce magnesium-rich garnet rims associated with potassium feldspar and melt via the reaction of Bt + Ph + Pl + Qz = Grt + Kfs + melt (Indares & Dunning, Reference Indares and Dunning2001). In accordance with the phase equilibria modelling, the following temperature increase provokes phengite-dehydration melting at T > 800°C and P > 10 kbar with kyanite formation (Figure 9(b)). The results of Zr-in-rutile thermometry show consistent values of ∼750–870°C (Table S5). Finer grains of garnet (Figure 5(i)) from the same sample of garnet–kyanite paragneiss AN1803 demonstrate prominent zoning in Ca distribution with the maximum contents in the cores and a gradual decrease towards the rims, marking a decompression reaction of garnet with kyanite to produce anorthite-rich plagioclase (Ganguly & Saxena, Reference Ganguly and Saxena1984). The XCa, XFe and XMg compositional isopleths of the garnet core (Table S1) exhibit an accurate intersection in a small area of P = 12 kbar and T = 770°C (Figure 9(b), stage III), whereas the P–T parameters of formation of the garnet rims are constrained by the best reproducibility of XCa, XFe and XMg isopleths at P = 10.5–11 kbar and T = 770–775°C in the stability field of both micas (Figure 9(b), stage IV). Final melt crystallization at T = ∼720°C is compatible with an assemblage of the common medium-grade garnet–mica paragneiss/schist, consisting of garnet, muscovite, biotite, plagioclase and quartz.
Widespread garnet–mica schists with relics of kyanite of the Koyandy complex may, therefore, represent the most altered varieties of the paragneisses, transformed to medium- to low-grade schists through a series of metamorphic events during their retrograde evolution. In turn, subordinate garnet-free schists associated with the garnet–mica schists and paragneisses (Figure 3) evidently underwent low-grade alterations and avoided HP re-equilibration.
4.3. Geochronology and Sm–Nd isotope systematics
4.3.1. Migmatized garnet–mica paragneisses
The detrital zircon population from the granitoid leucosome of the garnet–mica migmatized paragneiss (sample AN1801/2; Figure 3(d)) exhibits euhedral and subhedral habits and well-developed oscillatory zoning and is characterized by the presence of inherited cores (Figure 10(a)). The grain sizes of these detrital zircons vary in the range of 100–200 μm, and the elongation coefficient varies from 1.5 to 2.5. The analyzed zircon cores (SHRIMP II) have Th/U ratios consistent with their magmatic origin and ranging between 0.39 and 0.69, whereas their rims demonstrate significantly lower Th/U ratios of 0.01–0.09, interpreted to have originated from HP metamorphic re-equilibration (Table 2). Furthermore, separated grains with similar Th/U ratios are also found among the studied zircons (Figure 10(a)).
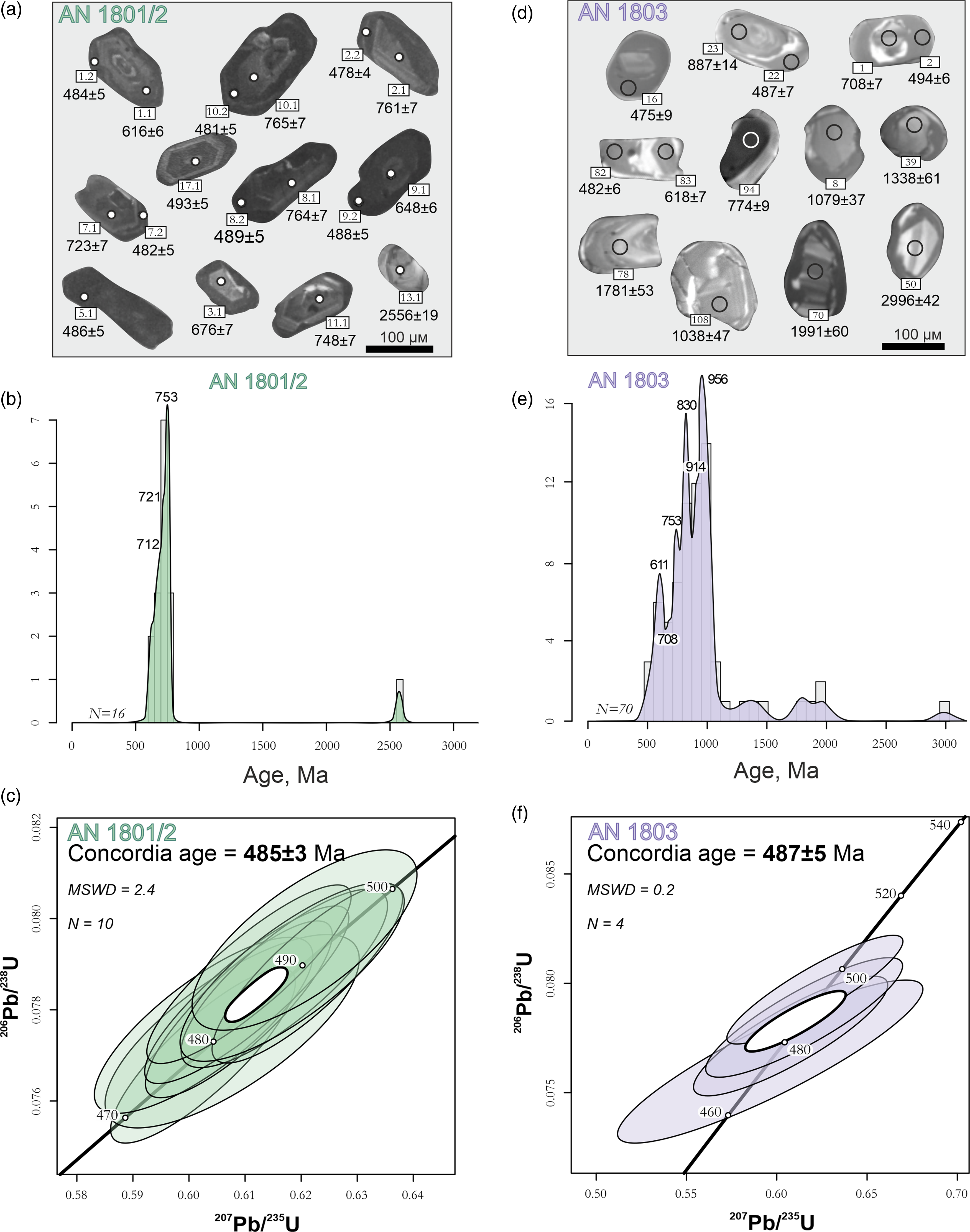
Figure 10. Cathodoluminescence photographs of zircon grains (a), (d), histograms of the relative probability for zircon core ages (b), (e) and concordia diagrams of younger zircon rim ages (c), (f) for paragneisses of the Koyandy complex (Zheltau terrane): (a)–(c): granitoid leucosome of garnet–mica paragneiss AN1801/2. The circles are SHRIMP II analytical spots; the numbers in the squares are in accordance with Tables 2 and 3. (d)–(f): Garnet–kyanite schist (retrograded paragneiss) AN1803. The circles are LA-ICP-MS analytical spots; the numbers in the squares are in accordance with Tables S6 and 3.
Table 2. Results of ion microprobe (SHRIMP II) U–Th–Pb analyses of zircons from the granitoid leucosome of garnet–mica migmatized paragneiss AN1801/2

Errors are 1-sigma; Pbc and Pb* indicate the common and radiogenic portions, respectively.
Error in standard calibration was 0.34% (not included in above errors but required when comparing data from different mounts).
(1) Common Pb corrected using measured 204Pb.
The observed zircon population yielded an age range of 616–766 Ma (SHRIMP II) for their cores, with population peaks at 712 Ma, 721 Ma and 753 Ma (Figure 10(b); Table 2, 3). The core of one zircon grain revealed a Neoarchean crystallization age of ∼2556±19 Ma, indicating the involvement of the early Precambrian basement as a source of clastic material in the protoliths of paragneisses (Table 2). The concordant 206Pb/238U age obtained from the rims of ten zircon grains and newly formed zircons corresponds to an age of 485 ± 3 Ma (MSWD = 2.4) (Figure 10(c); Table 2).
Table 3. Selected results of age peak (Gehrels, Reference Gehrels, Busby and Azor2012) calculations for detrital zircons from the granitoid leucosome of garnet–mica migmatized paragneiss AN1801/2 and garnet–kyanite schist (retrograded paragneiss) AN1803

The garnet–mica paragneisses are characterized by negative εNd(t) values of −4.9, consistent with our inference of the derivation of the protoliths of these rocks from a reworked, early Precambrian continental crustal source. The Nd model age tNd(DM) of this source is ca. 1.6 Ga (Table 4).
Table 4. Sm–Nd isotope data for the studied paragneisses within the Koyandy complex (Zheltau terrane)

4.3.2. Garnet–kyanite schists (retrograded paragneisses)
Zircon grains from the garnet–kyanite paragneiss AN1803 display subrounded habits and preserve oscillatory zoning and/or contain inherited cores. Their lengths vary between 50 and 130 μm with elongation coefficients of 1.0–2.5 (Figure 10(d)). The age population (LA-ICP-MS) of the analyzed zircon grains ranges between 593 and 1443 Ma, with the main peaks at 611 Ma, 708 Ma, 753 Ma, 830 Ma, 914 Ma and 956 Ma (Figure 10(b); Table 3, Table S6). The single zircon grains yield Meso- and Palaeoproterozoic ages of 1.8–2 Ga, indicating the presence of an ancient crustal source in the protoliths of these metasedimentary rocks (Table S6; Figure 10(e); Table 3). Some of these zircons have overgrown rims, and such rims in four of the zircons yield a concordant age of 487 ± 5 Ma (MSWD= 0.19) (Figure 10(f); Table S6). We interpret this age as the timing of the HP metamorphism experienced by the rocks.
A negative εNd(t) value of -11.01, obtained from the garnet–kyanite retrograded paragneisses, is compatible with a source of their terrigenous protoliths through partial melting of Palaeoproterozoic crust with an Nd model age of ∼2.2 Ga (Table 4).
5. Discussion
5.1. Precambrian tectonic affinity of the protoliths of the high-pressure paragneisses in the Koyandy complex
The observed garnet-bearing HP paragneisses of the Koyandy complex occurring in the SE part of the Anrakhai Block in the Zheltau terrane (Figure 3) have detrital zircon age distributions in the ranges of ca. 1443–593 Ma, with main peaks at 708–611 Ma, 830–753 Ma, 956–914 Ma (garnet–kyanite schist–retrograded paragneiss, sample AN1803) and ca. 766–616 Ma (granitoid leucosome of the garnet–mica migmatized paragneiss, sample AN1801/2) (Table 1–3; S6). The Sm–Nd isotope data and chemical compositions of the paragneisses with characteristic differentiated spectra of REE distributions and negative Eu anomalies of 0.63–0.64 (Figure 7; Table 4) indicate a predominance of felsic rocks in the source of the sedimentary protoliths of the paragneisses. These felsic rocks formed as a result of reworking of continental crust with an age range of ca. 2.2–1.6 Ga in accordance with their Nd model ages. The youngest single zircon grains from both types of paragneisses yielded ages of ca. 600–520 Ma, which constrain the timing of the lower limit of deposition for their protoliths as Ediacaran–lower Cambrian, whereas the upper limit is determined by the timing of HP metamorphism consistent with the obtained zircon rim ages of ca. 487–485 Ma (Figure 10(c) and (f)).
The garnet–mica schist with relics of kyanite (sample AN1470) and the low-grade muscovite-chlorite schist (sample AN1320) (Table 1) from the central part of the Anrakhai Block (Zheltau terrane) contain detrital zircon grains with peak age populations of ca. 834–667 Ma, 1051–868 Ma, 1220–1087 Ma, 1378–1296 Ma, 2539–2464 Ma and ca. 672–590 Ma, 790–695 Ma, 1332–905 Ma, 1491–1427 Ma and 2023–1991 Ma, respectively (Pilitsyna et al. Reference Pilitsyna, Tretyakov, Degtyarev, Salnikova, Kotov, Kovach, Wang, Batanova, Plotkina, Tolmacheva, Ermolaev and Lee2019), which are similar to the age peaks of the garnet–kyanite paragneiss (sample AN1803).
We compared the detrital zircon age peaks from the paragneisses and schists in the Koyandy complex by using ‘Overlap-Similarity’ software (Gehrels, Reference Gehrels2000). This comparative analysis has shown a high extent of overlapping zircon age distributions between these two major metamorphic units (Figure 11; Table 5). Moreover, an epidote-chlorite schist sample (АК1624) from the adjacent Kokdzhon complex of the Chu-Kendyktas terrane (Figure 1(b) and 2) exhibits detrital zircon age populations with main peaks at ca. 606 Ma, 796 Ma, 1026 Ma and 1608–1464 Ma and main spikes at 1480 and 1589 Ma (Skoblenko et al. Reference Skoblenko, Kanygina, Tretyakov, Degtyarev, Nguyen, Pang, Sheshukov and Erofeeva2023). Detrital zircon age assessments for samples AN1803, AN1470, AN1320 and AK1624 overlap to a degree of 0.629–0.747, and the extent of similarity is estimated to range from 0.672 to 0.758 (Table 5). These findings suggest that the protoliths of the analyzed rocks were possibly sourced from a single provenance area and that these protolith sedimentary rocks were deposited during the Ediacaran–Cambrian. On the other hand, the UHP metasedimentary rocks of the Makbal complex (Issyk-Kul terrane) are characterized by detrital zircon age distributions covering the ranges of ca. 2500–600 Ma, with the main spikes at ca. 1.95–1.75 Ga and 2.7–2.5 Ga (garnet–chloritoid–talc schists) and ca. 1.6 Ga, 1.95–1.75 Ga and 2.7–2.5 Ga (metaquartzites), which indicate much older ages of the clastic source(s) of their protoliths (Konopelko & Klemd, et al.2016). We infer that an early Precambrian continental crust was the main source for the protoliths of all the indicated metasedimentary formations of the Zheltau, Chu-Kendyktas and Issyk-Kul terranes in accordance with their Sm–Nd characteristics.

Figure 11. Normalized (a) and cumulative (b) probability plots (Gehrels, Reference Gehrels, Busby and Azor2012) with the age distributions obtained for detrital zircons from garnet–kyanite schist (retrograded paragneiss) AN1803 (this study), muscovite-chlorite schist АN1320 and garnet–mica schist АN1470 (Pilitsyna et al. Reference Pilitsyna, Tretyakov, Degtyarev, Salnikova, Kotov, Kovach, Wang, Batanova, Plotkina, Tolmacheva, Ermolaev and Lee2019) from the Koyandy complex (Zheltau terrane), and epidote–chlorite schist АК1624 of the Kokdzhon complex (Chu-Kendyktas terrane; Skoblenko et al. Reference Skoblenko, Kanygina, Tretyakov, Degtyarev, Nguyen, Pang, Sheshukov and Erofeeva2023). Consistent with Table 5.
Table 5. Comparison of the U–Th–Pb zircon data for the studied garnet–kyanite schist (retrograded paragneiss) AN1803 (this study), muscovite–chlorite schist АN1320 and garnet–mica schist АN1470 (Pilitsyna et al. Reference Pilitsyna, Tretyakov, Degtyarev, Salnikova, Kotov, Kovach, Wang, Batanova, Plotkina, Tolmacheva, Ermolaev and Lee2019) from the Koyandy complex (Zheltau terrane) and epidote–chlorite schist АК1624 of the Kokdzhon complex (Chu-Kendyktas terrane; Skoblenko et al. Reference Skoblenko, Kanygina, Tretyakov, Degtyarev, Nguyen, Pang, Sheshukov and Erofeeva2023). The degree of overlap determines whether two curves contain overlapping ages, and the degree of similarity displays whether overlapping ages have similar proportions. A value of 1.0 indicates a perfect match of all grains derived from the same source; 0.0 reflects no age match (Gehrels, Reference Gehrels2000)

The zircon core ages of ca. 766–616 Ma and a single-grain age of ca. 2550 Ma obtained from the granitoid leucosome of the garnet–mica migmatized paragneiss (sample AN1801/2) in the Koyandy complex of the Zheltau terrane demonstrate distinct age peaks at 712 Ma, 721 Ma and 753 Ma. However, older zircon age estimates are virtually absent in comparison to the abovementioned garnet–kyanite paragneiss (sample AN1803). The age distribution in sample AN1801/2 implies a predominance of late Neoproterozoic (Tonian–Cryogenian) complexes in the source for the migmatized paragneisses and is uncommon for the associated metasedimentary paragneisses and schists of the Zheltau terrane, showing the main age spike at ca. 1 Ga (Figure 10(e), 11). At the same time, within the Kemin and Kokdzhon complexes of the adjacent Chu-Kendyktas terrane (Figure 2), meta-arkose (sample AK1909) and amphibole-bearing paragneiss (sample АК1913/2) contain detrital zircons with cores dated at ca. 855–574 Ma and ca. 831–518 Ma, respectively (Skoblenko et al. Reference Skoblenko, Kanygina, Tretyakov, Degtyarev, Nguyen, Pang, Sheshukov and Erofeeva2023). We correlated the detrital zircon age assessments of samples AN1801/2, AK1909 and AK1913/2 by using ‘Overlap–Similarity’ software (Gehrels, Reference Gehrels, Busby and Azor2012). The rocks share many similarities in terms of the statistics of their zircon age peaks (Figure 12) and show a degree of overlap in the age data, varying from 0.510 to 0.754, i.e. proposing a possible single provenance area for the protoliths of these rocks (Table 6).
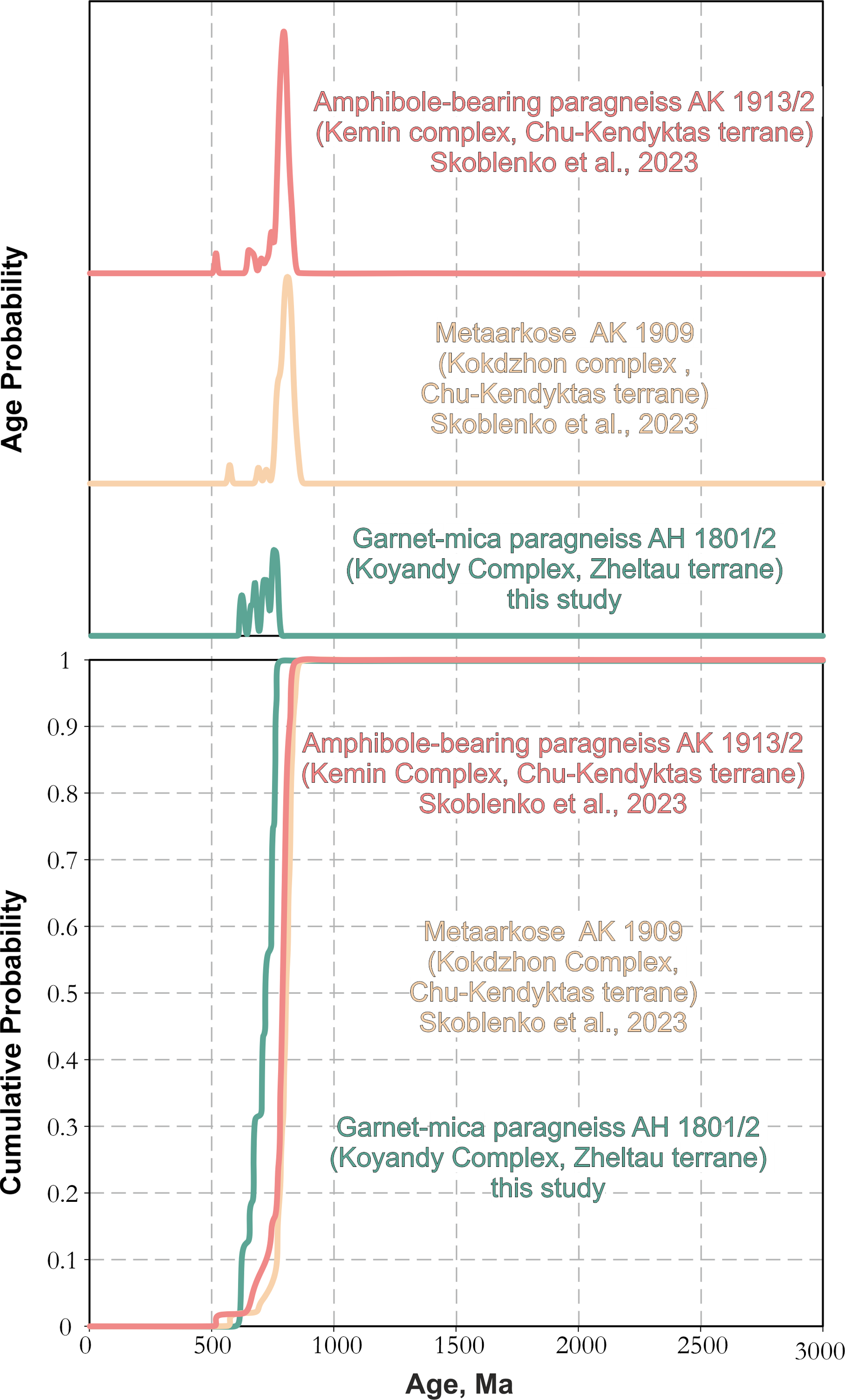
Figure 12. Normalized (a) and cumulative (b) probability plots (Gehrels, Reference Gehrels, Busby and Azor2012) with the age distributions obtained for detrital zircons from the migmatized garnet–mica paragneiss (leucosome) AH1801/2 of the Koyandy complex (Zheltau terrane; this study), meta-arkose АК1909 of the Kokdzhon complex and amphibole-bearing paragneiss АК1913/2 of the Kemin complex (Chu-Kendyktas terrane; Skoblenko et al. Reference Skoblenko, Kanygina, Tretyakov, Degtyarev, Nguyen, Pang, Sheshukov and Erofeeva2023). Consistent with Table 6.
Table 6. Comparison of the U–Th–Pb zircon data for the studied migmatized garnet–mica paragneiss (leucosome) AH1801/2 of the Koyandy complex (Zheltau terrane; this study), meta-arkose АК1909 of the Kokdzhon complex and amphibole-bearing paragneiss АК1913/2 of the Kemin complex (Chu-Kendyktas terrane; Skoblenko et al. Reference Skoblenko, Kanygina, Tretyakov, Degtyarev, Nguyen, Pang, Sheshukov and Erofeeva2023). The degree of overlap determines whether two curves contain overlapping ages, and the degree of similarity displays whether overlapping ages have similar proportions. A value of 1.0 indicates a perfect match of all grains derived from the same source; 0.0 reflects no age match (Gehrels, Reference Gehrels2000)

Hence, we posit that protoliths of the studied paragneisses and schists appear to have contained the same sedimentary cover as the Zheltau and Chu-Kendyktas terranes, whereas Palaeo-, Meso- and Neo-Proterozoic metamorphosed felsic volcanic rocks and granitoids constituted the basement of these terranes. The obtained zircon core age ranges and the Sm–Nd characteristics of the analyzed paragneisses and their derivatives are believed to reflect the long-term evolution of their protoliths prior to the onset of early Palaeozoic HP metamorphism. This metamorphic event and the associated deformation encompassed multiple stages of continental crustal growth, its subsequent metamorphic transformation, uplift and erosion of all crustal complexes and transport and deposition of all detrital material.
Manifestations of granitoid magmatism at ∼2.3 Ga and ∼1.8 Ga have been reported from the Kuilyu complex of the Middle Tian Shan (Naryn-Sarydzhaz) terrane (Kröner et al. Reference Kröner, Alexeiev, Kovach, Tretyakov, Mikolaichuk, Xie and Sobel2017b), as well as from the Zheltau terrane (Pilitsyna et al. Reference Pilitsyna, Tretyakov, Degtyarev, Salnikova, Kotov, Kovach, Wang, Batanova, Plotkina, Tolmacheva, Ermolaev and Lee2019) and the northern part of the Tarim Craton (He et al. Reference He, Zhang, Zong and Dong2013) (Figure 1(b)). Magmatic rock assemblages of ∼1.6 Ga are unknown within our study area; however, this age window is recorded by quartzites or quartzite-schist sequences of the Akbastau and Makbal Formations in the adjacent Chu-Kendyktas and Issyk-Kul terranes (Figure 1(b)) and marks the youngest magmatic episode providing the 1672–1625 Ma zircons (Kanygina et al. Reference Kanygina, Tretyakov, Degtyarev, Pang, Wang, Lee and Plotkina2019; Alexeiev et al. Reference Alexeiev, Khudoley and DuFrane2020). The age range of 1.5–1.3 Ga is traced within the Chinese Central Tien Shan terrane and the Yili Block at ca. 1450–1410 Ma and ca. 1329 Ma (Huang et al. Reference Huang, Yuan, Long, Zhang and Du2019; He et al. Reference He, Klemd, Zhang, Zong, Sun, Tian and Huang2015), the Issyk-Kul terrane at ca. 1.3 Ga (Kröner et al. Reference Kröner, Alexeiev, Rojas-Agramonte, Hegner, Wong, Xia, Belousova, Mikolaichuk, Seltmann, Liu and Kiselev2013) and the Ulutau terrane at ca. 1338 Ma (Tretyakov et al. Reference Tretyakov, Degtyarev, Kanygina and Fedorov2022) (Figure 1(b)). A pronounced age peak at ca. 1.1–0.9 Ga, observed from the studied paragneisses and schists, correlates well with the episodes of granitoid magmatism within the Issyk-Kul and Kokchetav terranes at ca. 1.1 Ga (Degtyarev et al. Reference Degtyarev, Tretyakov, Ryazantsev, Kotov, Salnikova, Aleksandrov and Anisimova2011; Tretyakov et al. Reference Tretyakov, Kotov, Degtyarev, Salnikova, Shatagin, Yakovleva and Anisimova2011a, b; Kröner et al. Reference Kröner, Alexeiev, Rojas-Agramonte, Hegner, Wong, Xia, Belousova, Mikolaichuk, Seltmann, Liu and Kiselev2013; Kushnareva et al. Reference Kushnareva, Khudoley, Alexeiev and Petrov2022) and the Aktau-Yili terrane at ca. 925 Ma (Tretyakov et al. Reference Tretyakov, Degtyarev, Shatagin, Kotov, Salnikova and Anisimova2015; Alexeiev et al. Reference Alexeiev, Degtyarev, Tretyakov and Kanygina2021) (Figure 1(b)). Finally, the late Tonian age range of 840–770 Ma is widespread within the Zheltau and adjacent Chu-Kendyktas terranes and documents the timing of voluminous granitoid magmatism in the North Tien Shan and South Kazakhstan (Kröner et al. Reference Kröner, Alexeiev, Hegner, Rojas-Agramonte, Corsini, Chao, Wong, Windley, Liu and Tretyakov2012; Pilitsyna et al. Reference Pilitsyna, Tretyakov, Degtyarev, Salnikova, Kotov, Kovach, Wang, Batanova, Plotkina, Tolmacheva, Ermolaev and Lee2019; Tretyakov et al. Reference Tretyakov, Pilitsyna, Degtyarev, Salnikova, Kovach, Lee, Batanova, Wang, Kanygina and Kovalchuk2019; Skoblenko et al. Reference Skoblenko, Degtyarev, Kanygina, Pang and Lee2022, Reference Skoblenko, Kanygina, Tretyakov, Degtyarev, Nguyen, Pang, Sheshukov and Erofeeva2023). The youngest zircon age peaks of ca. 700–600 Ma are assigned to a major part of the early Palaeozoic metasedimentary rocks of the Zheltau and Chu-Kendyktas terranes (Skoblenko et al. Reference Skoblenko, Degtyarev, Kanygina, Pang and Lee2022, Reference Skoblenko, Kanygina, Tretyakov, Degtyarev, Nguyen, Pang, Sheshukov and Erofeeva2023; this study); however, their source is unknown within the observed area because it might be either located beyond the study region or unexposed.
5.2. High-pressure metamorphism of continental crust within the Zheltau and adjacent terranes of the SW part of the Central Asian Orogenic Belt in the late Cambrian–Early Ordovician
Multiple lines of evidence for the occurrence of UHP metamorphism between 530 and 470 Ma have been reported for the Kokchetav, Issyk-Kul, Chu-Kendyktas and Zheltau terranes within the western segment of the CAOB (e.g. Shatsky et al. Reference Shatsky, Jagoutz, Sobolev, Kozmenko, Parkhomenko and Troesch1999; Togonbaeva et al. Reference Togonbaeva, Takasu, Bakirov, Sakurai, Tagiri, Bakirov and Sakiev2009; Ragozin et al. Reference Ragozin, Liou, Shatsky and Sobolev2009; Alexeiev et al. Reference Alexeiev, Ryazantsev, Kröner, Tretyakov, Xia and Liu2011; Rojas-Agramonte et al. Reference Rojas-Agramonte, Herwartz, García-Casco, Kröner, Alexeiev, Klemd, Buhre and Barth2013; Klemd et al. Reference Klemd, Hegner, Bergmann, Pfander, Li and Hentschel2014; Meyer et al. Reference Meyer, Klemd, Hegner and Konopelko2014; Konopelko et al. Reference Konopelko, Kullerud, Apayarov, Sakiev, Baruleva, Ravna and Lepekhina2012; Konopelko & Klemd, Reference Konopelko and Klemd2016; review of Skoblenko & Degtyarev, Reference Skoblenko and Degtyarev2021) (Figure 1(b)). Formation of the early Palaeozoic UHP rocks is believed to have been related to the subduction of different rock associations to depths of at least 60 km and possibly deeper to the stability field of coesite (Makbal complex, Issyk-Kul terrane; Zerendy Group; Kokchetav terrane) or even of diamond (Zerendy Group; Kokchetav terrane). The age estimates, which correspond to the near-peak stages of UHP metamorphism, were mostly obtained for eclogites by using U–Pb zircon and Sm–Nd with Lu–Hf garnet isochron dating. Although eclogites tend to show better preservation of HP mineral parageneses, deciphering the geochemical affiliation of eclogites and reconstructing the palaeotectonic position of their protoliths are challenging tasks. Accordingly, interpretations of oceanic ridge versus intraplate continental magmatism have been suggested by different authors for the eclogites of the Makbal (Meyer et al. Reference Meyer, Klemd, Hegner and Konopelko2014 vs. Rojas-Agramonte et al. Reference Rojas-Agramonte, Herwartz, García-Casco, Kröner, Alexeiev, Klemd, Buhre and Barth2013) and Aktyuz (Rojas-Agramonte et al. Reference Rojas-Agramonte, Herwartz, García-Casco, Kröner, Alexeiev, Klemd, Buhre and Barth2013 vs. Klemd et al. Reference Klemd, Hegner, Bergmann, Pfander, Li and Hentschel2014) complexes of the Issyk-Kul and Chu-Kendyktas terranes, respectively. Similarly, Precambrian pelitic sedimentary sequences (Konopelko & Klemd, Reference Konopelko and Klemd2016) versus hydrothermally altered oceanic crust (Meyer et al. Reference Meyer, Klemd, Hegner and Konopelko2014) are proposed as the protoliths for the UHP garnet–talc–chloritoid schists of the Makbal complex (Issyk-Kul terrane). Moreover, the origin of early Palaeozoic UHP metamorphism within the western CAOB is interpreted to have been closely related to the subduction of various fragments of continental crust under eclogite-facies conditions. This interpretation is supported by the findings of the involvement of Precambrian continental crust in subduction processes regarding the evolutionary history of the Zerendy Group of the Kokchetav terrane in Northern Kazakhstan (Claoue-Long et al. Reference Claoue-Long, Sobolev, Shatsky and Sobolev1991). Zircons recovered from diamondiferous garnet–biotite gneisses in the Zerendy Group have yielded U–Pb ages of UHP metamorphism at ca. 530 Ma, compatible with the ages obtained from the spatially associated eclogites (Shatsky et al. Reference Shatsky, Yagoutz, Kozmenko, Blinchik and Sobolev1993).
In the SW part of the CAOB in the North Tien Shan, coesite-bearing garnet quartzites of the Makbal complex (Issyk-Kul terrane) are known as strongly metamorphosed terrigenous crustal formations. However, zircon ages obtained from these rocks have not provided any constraints for the timing of UHP metamorphism; rather, the obtained data provided ages for their protoliths (Konopelko & Klemd et al. Reference Konopelko and Klemd2016). Two age clusters of ∼509–498 Ma (U–Pb zircon dating) and ∼470 Ma (Lu–Hf garnet isochron dating), which are thought to be consistent with the near-peak stage of metamorphism, were obtained from the amphibolised eclogites of the Makbal complex (Issyk-Kul terrane) (Konopelko et al. Reference Konopelko, Kullerud, Apayarov, Sakiev, Baruleva, Ravna and Lepekhina2012; Rojas-Agramonte et al. Reference Rojas-Agramonte, Herwartz, García-Casco, Kröner, Alexeiev, Klemd, Buhre and Barth2013). Diverse age assessments of the near-peak stage of UHP re-equilibration of ca. 502 Ma (U–Pb dating of zircon rims) and ca. 475 Ma (Sm–Nd garnet isochron dating) were also obtained for the associated garnet–talc–chloritoid schists (Konopelko et al. Reference Konopelko, Kullerud, Apayarov, Sakiev, Baruleva, Ravna and Lepekhina2012; Meyer et al. Reference Meyer, Klemd, Hegner and Konopelko2014). Protoliths of the UHP rocks of the Makbal complex were, hence, considered to have been subducted under the Chu-Kendyktas (North Tien Shan) terrane during the closure of the Kara-Archa ocean basin (Konopelko et al. Reference Konopelko, Kullerud, Apayarov, Sakiev, Baruleva, Ravna and Lepekhina2012) and then subjected to metamorphic transformations during the time window of 509–470 Ma. These multiple deformed and metamorphosed rock associations were subsequently exhumed as tectonic slivers, consisting of metamorphosed continental crustal assemblages intercalated with derivatives of ancient oceanic lithosphere, recorded by the ages of inherited zircon cores and Sm–Nd with Lu–Hf isotope data.
Metamorphic complexes of the Zheltau (South Kazakhstan) and Chu-Kendyktas terranes (North Tien Shan) bear a strong resemblance in terms of the development of their Precambrian meta-magmatic and metasedimentary formations, which show strong similarities in the ages and compositions of their protolith source (Paragraph 5.1; Skoblenko et al. Reference Skoblenko, Degtyarev, Kanygina, Pang and Lee2022). The HP rocks attributed to the Koyandy (Zheltau terrane) and Aktyuz complexes (Chu-Kendyktas terrane) are located at a distance of ∼100 km relative to each other (Figure 2) and are separated by an early Palaeozoic (531–520 Ma based on plutonic units) Dzhalair-Naiman ophiolite zone (Ryazantsev et al. Reference Ryazantsev, Mikolaychuk, Tolmacheva, Degtyarev, Kotov, Nikitina, Mamonov and Zorin2009; Kröner et al. Reference Kröner, Alexeiev, Hegner, Rojas-Agramonte, Corsini, Chao, Wong, Windley, Liu and Tretyakov2012). The metamorphic history of the high-grade rocks of both complexes is, therefore, expected to have involved the same tectonic events associated with the evolution of the Dzhalair-Naiman ocean basin.
Variably amphibolised eclogites and garnet pyroxenites (only the Koyandy complex) that are spatially associated with HP garnet-bearing gneisses and their retrograded derivatives within the Koyandy and Aktyuz complexes are juxtaposed against moderate- to low-grade gneissic granites, schists and locally occurring marbles. None of these rocks shows any mineralogical or textural evidence for HP re-equilibration. Zircons from garnet pyroxenites of the Koyandy complex yield a mean U–Pb age of HP metamorphism of ca. 490 Ma (Alexeiev et al. Reference Alexeiev, Ryazantsev, Kröner, Tretyakov, Xia and Liu2011) at P–T conditions of 15–19 kbar and 750–850°C (Pilitsyna et al. Reference Pilitsyna, Tretyakov, Degtyarev, Cuthbert, Batanova and Kovalchuk2018a). A Lu–Hf garnet isochron age (∼474 Ma) has been interpreted as the near-peak stage, HP metamorphism of the eclogites in the Aktyuz complex at P = 21 kbar and T = 670°C (Rojas-Agramonte et al. Reference Rojas-Agramonte, Herwartz, García-Casco, Kröner, Alexeiev, Klemd, Buhre and Barth2013). In turn, zircons from the HP garnet gneisses in the Aktyuz complex, which contains eclogite bodies of variable sizes, display two age clusters of ca. 844 Ma (zircon cores) and ca. 490 Ma (zircon rims and separated grains). These ages mark two main stages of the evolution of the host rocks in the late Neoproterozoic (emplacement of their protoliths) and in the latest Cambrian (HP metamorphism of the gneisses’ protoliths) and the following stages of rock exhumation at 490–471 Ma (Skoblenko et al. Reference Skoblenko, Kanygina, Tretyakov, Degtyarev, Nguyen, Pang, Sheshukov and Erofeeva2023).
An affinity of the garnet-bearing gneisses to the HP rocks formed at P = ∼13–15 kbar and T = 635–745°C has been suggested previously by Orozbaev et al. (Reference Orozbaev, Takasu, Bakirov and Sakiev2010). This comparison is in agreement with the preliminary results of pseudosection modelling in conjunction with the garnet isopleth geothermobarometers of P = 10 kbar and T = 700°C at the prograde stage of garnet nucleation and P = 14 kbar and T = 760°C at the near-peak stage of the formation and melting propagation of garnet rims (Figure S1; Table S1). Garnet–mica and garnet–kyanite paragneisses of the Koyandy complex formed at the prograde metamorphic stage at P = 9–12 kbar and T = 720–760°C, whereas the near-peak to retrograde stage of development occurred at P = 15–18 kbar and T = 750–870°C (Pilitsyna et al. Reference Pilitsyna, Tretyakov, Degtyarev, Salnikova, Kotov, Kovach, Wang, Batanova, Plotkina, Tolmacheva, Ermolaev and Lee2019; this study (Figure 8 and 9)). Detrital zircons obtained from the paragneisses and schists show different age distributions in the cores (Paragraph 5.1), whereas the rims appear to have grown during HP re-equilibration at ∼487–485 Ma. This age range is compatible with the age estimate of ca. 490 Ma known from the eclogite-bearing garnet gneisses of the Aktyuz complex (Figure 13).

Figure 13. Concordia diagram and normalized probability plot (Gehrels, Reference Gehrels, Busby and Azor2012) for zircon rim ages corresponding to the timing of the high-pressure (HP) metamorphism re-equilibration from the garnet–kyanite schist (retrograded paragneiss) AN1803 (Koyandy complex, Zheltau terrane; this study), granitoid leucosome of garnet–mica paragneiss AN1801/2 (Koyandy complex, Zheltau terrane; this study) and garnet–mica gneiss AK1904 (Aktyuz complex, Chu-Kendyktas terrane; Skoblenko et al. Reference Skoblenko, Kanygina, Tretyakov, Degtyarev, Nguyen, Pang, Sheshukov and Erofeeva2023).
Thus, we propose a clockwise, ‘subduction-type’ P–T evolution trajectory for all the early Palaeozoic UHP metamorphic rocks of the Makbal, Aktyuz and Koyandy complexes in the SW part of the CAOB (Figure 1(b)). The zircon age estimates obtained from the HP quartz–feldspar gneisses and their retrograded varieties indicate the involvement of crustal rocks in a Precambrian basement (Aktyuz complex; magmatic zircon cores of Tonian age) and the Ediacaran–Cambrian sedimentary cover of this basement (Makbal, Kemin and Koyandy complexes; detrital zircons) in subduction zone processes during the closure of the Palaeo-Asian Ocean in the latest Cambrian through Early Ordovician.
5.3. Assessment of the existing models of the Cambrian–Early Ordovician evolution of metamorphic complexes in the Zheltau and adjacent terranes in the SW part of the Central Asian Orogenic Belt
Based on the results of our study and the extant data in the literature, we propose a tectonic model explaining the geological evolution of the Precambrian metamorphic complexes in the Zheltau and Chu-Kendyktas terranes within the SW segment of the CAOB (Skoblenko et al. Reference Skoblenko, Degtyarev, Kanygina, Pang and Lee2022; this study). We recognize that the Precambrian evolutionary trend of the Issyk-Kul terrane differs from that of the Zheltau and Chu-Kendyktas terranes (Degtyarev et al. Reference Degtyarev, Yakubchuk, Tretyakov, Kotov and Kovach2017; Kanygina et al. Reference Kanygina, Tretyakov, Degtyarev, Kovach, Skuzovatov, Pang, Wang and Lee2021). The Ediacaran–Cambrian continental rifting and seafloor spreading processes resulted in the opening of the Palaeo-Asian Ocean, encompassing multiple seaways that separated various continental blocks. The remnants of one of these seaways occur in the Cambrian Dzhalair–Naiman zone (Figure 2) and consist of variously serpentinized mafic–ultramafic rocks of residual and layered complexes, gabbroids and plagiogranites, as well as bimodal basalt–rhyolite series (Ryazantsev et al. Reference Ryazantsev, Mikolaychuk, Tolmacheva, Degtyarev, Kotov, Nikitina, Mamonov and Zorin2009). Metagabbroids in the Dzhalair–Naiman zone are ∼531 Ma in age and are part of the Kopurelisay complex within the Aktyuz continental block (Chu-Kendyktas terrane). Serpentinites and gabbroids in the Anrakhai block within the Zheltau terrane (Figure 2 and 3) may potentially be part of the Dzhalair–Naiman zone, and together with the Kopurelisay complex, they may represent a suprasubduction-zone (SSZ) ophiolite. This SSZ oceanic crust evolved into the development of an enigmatic island arc (Sulusay island arc associated with OIB volcanism) at ∼510–500 Ma (Ryazantsev et al. Reference Ryazantsev, Mikolaychuk, Tolmacheva, Degtyarev, Kotov, Nikitina, Mamonov and Zorin2009; Degtyarev, pers. comm.), reminiscent of island arc-type ophiolites (Dilek & Furnes, Reference Dilek and Furnes2011, Reference Dilek and Furnes2014). Metamorphic formations containing HP mineral assemblages (i.e. Koyandy and Aktyuz complexes) within the studied terranes of South Kazakhstan and the North Tien Shan generally occur with tectonically juxtaposed fragments of ophiolites (Figure 2 and 3).
The presence of eclogites in both the Koyandy and Aktyuz complexes of the Zheltau and Chu-Kendyktas terranes is a distinguishing geological feature (Paragraph 5.2). However, the available different ages of HP metamorphism (∼490 and ∼474 Ma) and variable modal and chemical compositions of the metamorphic and ophiolitic rock units have allowed different interpretations for the Cambrian–Early Ordovician tectonic evolution of those eclogites. Alexeiev et al. (Reference Alexeiev, Ryazantsev, Kröner, Tretyakov, Xia and Liu2011) and Kröner et al. (Reference Kröner, Alexeiev, Hegner, Rojas-Agramonte, Corsini, Chao, Wong, Windley, Liu and Tretyakov2012) suggested, for example, that NE subduction of the oceanic lithosphere of the Dzhalair–Naiman basin under the Zheltau terrane might have involved subduction erosion, i.e. rock material scraped off from the bottom of the Zheltau terrane in the upper plate and downwards transport of this eroded material along the subduction interface at approximately 490–485 Ma. During this subduction process, the eroded crustal fragments underwent metamorphic transformations up to eclogite-facies conditions, recorded by the U–Pb zircon age of garnet pyroxenites intercalated with eclogites of ca. 490 Ma (Koyandy complex). Continued subduction of the oceanic lithosphere led to the terminal closure of the Dzhalair–Naiman basin and the collision of the Zheltau and Chu-Kendyktas terranes at approximately 485–474 Ma. Partial subduction of the passive margin of the Chu-Kendyktas terrane caused HP metamorphism of its siliciclastic and carbonate rock assemblages in the latest Cambrian, followed by their exhumation (e.g. as considered in models of Dilek et al. (Reference Dilek, Festa, Ogawa and Pini2012); Barbero et al. (Reference Barbero, Dilek, Festa and Saccani2023)). The second stage subduction of oceanic lithosphere is marked by a Lu–Hf garnet isochron age (ca. 474 Ma) of the eclogites in the Aktyuz complex, which were similarly exhumed along with the adjacent crustal formations after slab break-off.
On the other hand, Pilitsyna et al. (Reference Pilitsyna, Tretyakov, Degtyarev, Alifirova, Batanova, Cuthbert, Kovalchuk and Ermolaev2018b) proposed that two different oceanic basins must have existed in the vicinity of the Zheltau and Chu-Kendyktas terranes during the Cambrian; the terranes were separated by the Dzhalair-Naiman ocean basin that existed between the Zheltau and Chu-Kendyktas terranes, while another basin existed to the north of the Zheltau terrane. Diachronous processes of subduction of the ocean floor within these two basins during 500–474 Ma led to the partial subduction of the passive margin of the Zheltau terrane under the Aktau-Yili (?) terrane (Figure 1(b)) to form eclogites and garnet pyroxenites (aged ca. 490 Ma), which are currently spatially associated with the HP felsic gneisses and symplectite-bearing peridotites and with the partial subduction of the continental margin of the Chu-Kendyktas terrane under the Zheltau terrane after the closure of the Dzhalair-Naiman basin. This event resulted in the formation of eclogites and quartz–felspar rock assemblages at approximately 474 Ma. Exhumation of all the HP complexes occurred during the Early Ordovician.
We evaluated the contradicting aspects of these two models based on our new data obtained for the Zheltau and Chu-Kendyktas terranes. Age clusters of ∼844 Ma and ∼490 Ma obtained from the zircons recovered from the high-grade garnet gneisses of the Aktyuz complex (Skoblenko et al. Reference Skoblenko, Kanygina, Tretyakov, Degtyarev, Nguyen, Pang, Sheshukov and Erofeeva2023), which are spatially associated with the 474 Ma eclogites, provide strong geological and geochronological evidence for the partial subduction of the continental crust of the Chu-Kendyktas terrane during the latest Cambrian. This association makes the origin and affinity of the eclogites in the Aktyuz complex ambiguous. Moreover, even assuming a diachronous prograde-to-peak metamorphism of the continental crust (gneisses) and oceanic (?) (eclogite) complexes of the Chu-Kendyktas terrane and their mutual exhumation at 490–471 Ma reflected by zircon rim ages, it appears to be hardly feasible for the gneisses to have retained garnet zoning during ∼20–15 Ma of residence until the protoliths of eclogites achieved HP conditions. In fact, both eclogites and enclosing garnet gneisses of the Aktyuz complex exhibit well-developed textural evidence of ‘prograde-type’ zoning with a decrease in Mn contents from cores to rims (Figure S2; Table S1). In turn, the spatial closeness of the Zheltau and Chu-Kendyktas terranes, nearly equal compositions of the ophiolitic complexes developed in the NE part of the Anrakhai block (Figure 3) and within the Kopurelisay complex (Figure 2), along with the obtained age of HP metamorphism of garnet gneisses of the Aktyuz complex compatible to that of the eclogites with garnet pyroxenites of the Koyandy complex and garnet–mica paragneisses of ca. 490–487 Ma, lend support for a single ocean basin (i.e. Dzhalair-Naiman basin) evolution of the studied complexes, rather than two different basins. Notably, despite very similar characteristics of the high-grade crustal quartz–feldspar rocks of the Aktyuz and Koyandy complexes attributed to the same stage of metamorphism at ca. 490 Ma, eclogites of these complexes are different in terms of the P–T parameters of their formation and chemical compositions. Phengite eclogites of the Aktyuz complex are derivatives of the ‘cold’ geothermal gradient (P = 21 kbar; T = 670°C; Klemd et al. Reference Klemd, Hegner, Bergmann, Pfander, Li and Hentschel2014), whereas eclogites of the Koyandy complex were metamorphosed at the peak P–T conditions of the ‘hot’ gradient of 15–19 kbar and 750–850°C (Pilitsyna et al. Reference Pilitsyna, Tretyakov, Degtyarev, Cuthbert, Batanova and Kovalchuk2018a) (Figure S2). These findings may favour a distinct, oceanic tectonic setting for the formation of the Aktyuz eclogite protoliths. Protoliths of the eclogites in the Koyandy complex were already included in a sequence of continental crust prior to their HP metamorphism. Based on these observations and findings, we suggest that the Lu–Hf garnet isochron age of the near-peak stage of metamorphism for the Aktyuz eclogites should be reconsidered.
The age estimates obtained for the UHP formations of the Makbal complex in the Issyk-Kul terrane (Figure 1(b)) also appear to be ambiguous. Both age assessments of ∼509–498 Ma and ∼470 Ma have been interpreted as the timing of the near-peak stage of UHP metamorphism of amphibolised eclogitic rock associations (Konopelko et al. Reference Konopelko, Kullerud, Apayarov, Sakiev, Baruleva, Ravna and Lepekhina2012; Rojas-Agramonte et al. Reference Rojas-Agramonte, Herwartz, García-Casco, Kröner, Alexeiev, Klemd, Buhre and Barth2013). Similarly, major discrepancies exist in the timing of UHP metamorphism for garnet–talc–chloritoid schists, as reflected by the existing ages of 502 Ma and ca. 475 Ma (Konopelko et al. Reference Konopelko, Kullerud, Apayarov, Sakiev, Baruleva, Ravna and Lepekhina2012; Meyer et al. Reference Meyer, Klemd, Hegner and Konopelko2014). These age values indicate that we can constrain the timing of HP–UHP metamorphism episodes in the early Palaeozoic evolution of the CAOB.
Although there is a strong consensus on the occurrence of UHP metamorphism and overprinted retrogression of the Precambrian continental crust in the Issyk-Kul, Chu-Kendyktas and Zheltau terranes during the period of 509–474 Ma, the existing tectonic models are controversial and require a re-evaluation of the available age estimates and conduction of systematic, field-based geochronological and isotopic studies.
6. Conclusions
-
1. Migmatized kyanite-bearing garnet–mica paragneisses, garnet–kyanite paragneisses and their retrograded derivatives in the Koyandy complex within the Zheltau terrane in South Kazakhstan (SW part of the Central Asian Orogenic Belt) represent fragments of Precambrian continental crust that underwent HP re-equilibration in the latest Cambrian.
-
2. Prograde-stage metamorphism of the migmatized kyanite-bearing garnet–mica paragneisses occurred under P–T conditions of 9–12 kbar and 720–760°C, consistent with suprasolidus garnet growth under increased P–T and subsequent melt propagation conditions. The existence of kyanite in garnet rims constrains the lower P–T limit to 18.5 kbar and 870°C, whereas the retrograde growth of white mica is consistent with the P–T ranges of 18.5–12 kbar and 870–800°C. Detrital zircons from the granitoid leucosome of the garnet–mica paragneisses have yielded an age range between ∼616 Ma and 766 Ma (SHRIMP II) for the cores. Concordant 206Pb/238U ages obtained from the rims of ten zircons and two separated zircon grains, which grew during the HP metamorphic event, correspond to ca. 485 Ma.
-
3. Coarse garnet grains obtained from the garnet–kyanite paragneisses of the Koyandy complex record P–T parameters of 8–9.5 kbar and 710–720°C for the prograde stage of their metamorphism. The near-peak metamorphic conditions of these rocks are determined to be P = 15–17 kbar and T = 760–810°C. Extensive kyanite growth is consistent with phengite-dehydration melting at T > 800°C and P> 10 kbar, whereas the formation of finer garnet grains indicates retrograde evolution of their host rocks at P = 12–10.5 kbar and T = ∼770°C. Detrital zircon cores from the garnet–kyanite paragneisses (LA-ICP-MS) cover the age range of 593–1443 Ma, with main peaks at 611 Ma, 708 Ma, 753 Ma, 830 Ma, 914 Ma and 956 Ma. The rims of four zircon grains reveal a concordant age of ca. 487 Ma, which we interpret as the timing of the near-peak stage of HP metamorphism of their host rocks.
-
4. A comparison of the age peaks obtained from the paragneisses and schists of the Koyandy complex with those reported from the Kemin and Kokdzhon complexes in the adjacent Chu-Kendyktas terrane in the North Tien Shan shows strong similarities. This positive comparison allows us to suggest that sediments of the protoliths of the paragneisses and schists were possibly sourced from a single provenance area and that they were deposited during the Ediacaran–Cambrian. Among the high-grade rock formations of the Aktyuz complex in the Chu-Kendyktas terrane, eclogite-bearing garnet gneisses have yielded an age cluster of ca. 490 Ma for zircon rims. We interpret this age as the timing of HP re-equilibration. We, therefore, propose a mutual Precambrian–early Palaeozoic evolutionary trend for the high-grade metamorphic complexes in the Zheltau and Chu-Kendyktas terranes.
-
5. Clockwise ‘subduction-type’ P–T paths are suggested for the development of the early Palaeozoic HP metamorphic rocks in the SW part of the CAOB. The zircon age estimates obtained from the HP quartz–feldspar gneisses and their retrograded derivatives in the Zheltau and Chu-Kendyktas terranes indicate that Precambrian basement rocks (Aktyuz complex; magmatic zircon cores of Tonian age of ca. 844 Ma) and Ediacaran–Cambrian sedimentary cover rocks (Kokdzhon, Kemin and Koyandy complexes; detrital zircons) were involved in the latest Cambrian subduction zone processes during the closing stages of the Palaeo-Asian Ocean.
Supplementary material
The supplementary material for this article can be found at https://doi.org/10.1017/S0016756823000626
Acknowledgements
The authors are grateful to Prof. Dmitry Gladkochub and an anonymous reviewer for their constructive comments and useful suggestions. A.S. is sincerely thankful to Prof. Alexander V. Sobolev for the access to the IMAP and kind support, and Dr. Andrey A. Tretyakov for his help during the fieldwork in Kazakhstan.
Funding statement
The study was supported by the Russian Science Foundation (Grant № 21-77-00055). Purchase and maintenance of Electron Probe Microanalysator in ISTerre and participation of V. Batanova were supported by the grant from the European Research Council under the European Union’s Horizon H2020 research and innovation programme (Synergy Grant MEET, grant agreement no. 856555).
Competing interests
All authors have participated in (a) conception and design, or analysis and interpretation of the data; (b) drafting the article or revising it critically for important intellectual content; and (c) approval of the final version. This manuscript has not been submitted to, nor is it under review at, another journal or other publishing venue.
Authorship
The authors have no affiliation with any organization with a direct or indirect financial interest in the subject matter discussed in the manuscript. The following authors have affiliations with organizations with direct or indirect financial interest in the subject matter discussed in the manuscript: Anfisa V. Skoblenko (Pilitsyna): Geological Institute, Russian Academy of Sciences, Pyzhevsky lane, 7a, Moscow, Russia; Nadezhda A. Kanygina: Geological Institute, Russian Academy of Sciences, Pyzhevsky lane, 7a, Moscow, Russia; Alexander S. Dubenskiy: Geological Institute, Russian Academy of Sciences, Pyzhevsky lane, 7a, Moscow, Russia; Valentina G. Batanova: Univ. Grenoble Alpes, Univ. Savoie Mont Blanc, CNRS, IRD, IFSTTAR, ISTerre, 38000 Grenoble, France; Yildirim Dilek: Department of Geology & Environmental Earth Science, Miami University, Oxford, OH 45056, USA; Victor S. Sheshukov: Geological Institute, Russian Academy of Sciences, Pyzhevsky lane, 7a, Moscow, Russia; and Pavel A. Serov: Geological Institute of the Kola Science Centre, Russian Academy of Sciences, 184209 Apatity, Russia.