Introduction
Traditional Chinese Medicine (TCM) refers to the holistic approach to diagnosis, pathophysiology and therapy in the Chinese materia medica, based on over 2000 years of accumulated knowledge and practice (Ref. Reference Xu1). Major aspects of the practice include herbal medication, acupuncture and other physical therapy such as massage (Refs Reference Chan2, Reference KJ and H3, Reference Tang4). Outside of China, the practice is generally regarded as a complementary or ‘alternative’ form of medicine, although the international prevalence of the practice has been steadily increasing (Ref. Reference Chan5). Acceptance of TCM by the scientific community has been limited at best. Certain guiding principles of TCM such as the concept of ‘vital energy’ are esoteric and difficult to validate under modern scientific methods, and the TCM approach to both diagnosis and treatment also fundamentally differs from conventional Western methods (Refs Reference Jiang6, Reference Dong7). Nevertheless, there are select aspects of the practice that hold clear promise for modern evidence-based medicine. The field of Chinese herbals, in particular, has drawn increasing interest as a source of novel drugs and drug leads, which is unsurprising considering that many modern drugs are in fact derivatives of herbal medicines and natural products (Refs Reference Newman and Cragg8, Reference Cragg and Newman9, Reference Corson and Crews10, Reference Li and Vederas11). The awarding of part of the 2015 Nobel Prize in Physiology or Medicine for Tu Youyou's discovery and development of artemisinin, a potent antimalarial derived from the herbal Artemisia annua, is a clear example and reminder of the potential held by herbal medicine (Ref. Reference Tu12). Looking ahead, further understanding and appreciation of the current status and successes of TCM herbals will be important for continued developments in the field. In this paper, we seek to provide an overview of some of the major contributions that Chinese herbals have made to modern medicine, focusing on the background and process of discovery, molecular mechanisms, clinical evidence for established treatments as well as novel and promising treatments under development.
Artemisinin in the treatment of malaria
Malaria is caused by the Plasmodium genus of endoparasites and remains a global health concern with 212 million new cases and 429 000 deaths as recently as 2015 (Ref. 13). The artemisinin family of sesquiterpene lactone compounds, including artemisinin itself as well as synthetic derivatives such as dihydroartemisinin, artesunate and artemether (Fig. 1), currently serve as the standard treatment and represent the front line of antimalarial drugs with remarkable potency, specificity and safety (Refs 14, Reference van Agtmael, Eggelte and van Boxtel15, Reference Hoshen16). ACT (artemisinin-based combination therapies) remain the most effective and recommended treatment regimens for uncomplicated malaria, especially in cases caused by the prevalent Plasmodium falciparum strain of parasites (Refs Reference Looareesuwan17, Reference Bich18). The compound is derived from A. annua or ‘sweet wormwood’, which has been used in TCM (where it is known as ‘Qinghao’) for the relief of periodic fevers, a symptom of malaria (Ref. Reference Klayman19). The earliest documentation of such usage dates back to the East Jin Dynasty between 317 and 420 A.D., in Ge Hong's A Handbook of Prescriptions for Emergencies (Refs Reference Tu12, Reference Tu20).
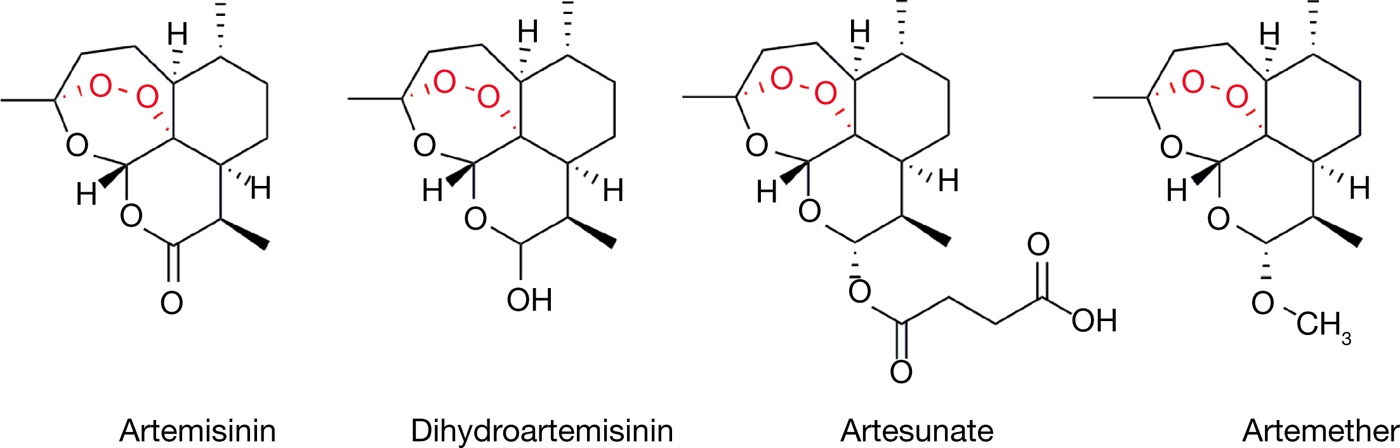
Figure 1. The structures of artemisinin and its clinically used derivatives.
The story of artemisinin is likely the most well-known example of Chinese medicine's contributions to modern medicine, given its outstanding real-world results and the recognition of the 2015 Nobel Prize. Under the Chinese nationwide antimalarial research initiative ‘Project 523’, the antimalarial agency headed by Tu Youyou in her institute performed extensive screening without success in hundreds of Chinese medicine-derived compounds, including A. annua (Refs Reference Tu12, Reference Tu20). With reference to the ancient literature which described preparation conditions for the herb, Tu then attempted a low-temperature approach in extracting the active components of A. annua and in 1971 identified a particular non-toxic extract which displayed close to 100% efficacy in mouse malaria models. The extract was quickly brought to clinical trial where recovery and full parasite clearance was reported in over 90% of cases. The active compound of A. annua was then successfully purified by the same group in 1972 and named artemisinin, or Qinghaosu in Chinese Mandarin. Finally, the full stereochemical structure of artemisinin was elucidated and published in 1977 (Ref. 21). Further work by Tu and others in the modification and derivitisation of artemisinin set the foundation for further understanding of critical functional groups as well as the development of artemisinin derivatives such as dihydroartemisinin, artesunate and artemether, which now serve as indispensable antimalarials worldwide (Refs 22, Reference Wang23).
Since artemisinin began to draw global attention in the early 1980s, numerous clinical studies have been performed in endemic regions to assess its safety and potency either in combination with other antimalarials or as a monotherapy. In particular, studies from China, Africa and Southeast Asia over the past three decades including recent meta-analytical data have convincingly demonstrated the outstanding clinical properties of artemisinin. Clinical results were marked by rapid parasite clearance and improvement of symptoms, especially for uncomplicated P. falciparum malaria in combination with longer-acting antimalarials such as mefloquine, lumefantrine, benflumetol and piperaquine (Refs Reference Looareesuwan17, Reference Bich18, Reference Li24, Reference Looareesuwan25, Reference von Seidlein26, Reference von Seidlein27, Reference Doherty28, 29). Results in severe malaria have likewise been generally positive for artemisinin derivatives compared with other treatments such as quinine, albeit with reduced efficacy compared with uncomplicated cases (Refs Reference Dondorp30, Reference McIntosh and Olliaro31, Reference Sinclair32). Reports of major adverse effects have been minimal, especially considering the volume of available data and the ubiquity of the drug (Refs Reference van Agtmael, Eggelte and van Boxtel15, Reference Efferth and Kaina33). In addition to its place as the most important antimalarial, limited clinical success has also been reported for the role of artemisinin in other diseases such as colorectal cancer, and research is ongoing for the re-purposing of artemisinin for various nonmalarial roles (Refs Reference Efferth34, Reference Ho35, Reference Krishna36, Reference Efferth37, Reference Li38).
Despite the widespread usage of artemisinin, the current molecular understanding of its mechanism of action remains incomplete. The artemisinin compounds are sesquiterpene lactones sharing the 1,2,4-trioxane pharmacophore, which contains an endoperoxide bridge that is thought to be essential for its pharmacological activity against both malaria as well as cancer (Ref. Reference Posner and O'Neill39). Artemisinin itself is a prodrug that must be activated via cleavage of this endoperoxide bridge for drug activity, although the mechanism of this activation remains an issue of some debate (Ref. Reference O'Neill40). Both free ferrous iron (Fe2+) as well as haem released from haemoglobin digestion have been proposed to activate artemisinin (Refs Reference Stocks41, Reference Wang42, Reference Zhou43). Reductive ion transfer (from iron or haem) is proposed to induce homolytic cleavage of the endoperoxide bridge, producing oxygen-centred radicals that subsequently isomerise to form reactive carbon-centred radicals (Refs Reference Posner44, Reference Jefford45, Reference Wu46). In an alternative theory, free Fe2+ functions as a Lewis acid in catalysing the heterolytic cleavage of the endoperoxide, generating cationic intermediates and hydroperoxides that can subsequently generate reactive hydroxyl radicals (Ref. Reference Haynes47). While free ferrous iron has been understood to be responsible for artemisinin activation, recent evidence from mass spectrometry and proteomics approaches have demonstrated that redox active haem could also play an important and possibly principal role in the process (Refs Reference Wang42, Reference Zhou43, Reference Zhang and Gerhard48, Reference Meunier and Robert49). It will be important to clearly elucidate the activation mechanism of artemisinin as this could directly relate to its remarkable specificity.
Following activation, the downstream mechanisms by which artemisinin achieves its antimalarial properties are likewise incompletely understood (Ref. Reference O'Neill40). Malaria parasites break down host cell haemoglobins, releasing large amounts of haem in the process (Refs Reference Lew50, Reference Klonis51). Haem build-up then leads to haematin formation which can induce oxidative and lytic damage to the parasite (Ref. Reference Fitch52). As a defence mechanism, the parasites are able to crystallise toxic haematin to the nontoxic haemozoin (Ref. Reference Egan53). Activated artemisinin has been shown to be able to bind and alkylate haem both in vitro and in vivo, possibly preventing haemozoin formation and causing a toxic accumulation of haem (Refs Reference Cazelles54, Reference Robert55, Reference Loup56, Reference Bousejra-El Garah57). Likewise, activated artemisinin is also known to be able to alkylate protein targets, which could interfere with critical biological functions and contribute to toxicity (Refs Reference Yang58, Reference Meshnick59). The identification of such protein targets of artemisinin has been a topic of great interest, and well-described examples include TCTP (translationally controlled tumour protein) and PfATP6 (Refs Reference Bhisutthibhan60, Reference Eckstein-Ludwig61). In particular, PfATP6 (a sarco/endoplasmic reticulum Ca2+-ATPase or SERCA) was reported to be bound and inhibited by artemisinin, making it a putative target of interest (Ref. Reference Arnou62). Recent reports using mass spectrometry-based proteomics approaches have highlighted a potentially promiscuous and indiscriminate targeting mechanism of artemisinin, where cellular targets including proteins are nonselectively alkylated in a proximity-dependent manner (Refs Reference Wang42, Reference Zhou43, Reference Li63). Our 2015 study identified over 100 binding targets of artemisinin using chemical probes (Fig. 2), implicating multiple cellular functions, including biosynthetic and metabolic pathways (Ref. Reference Wang42). Under such a model, a large number of proteins may be affected simultaneously and many critical biological pathways could be disrupted. The specificity of artemisinin, in this case, could then be largely attributed to the conditions and the extent of drug activation, rather than the specific targeting of protein effectors (Fig. 3). A combination of haem- and protein alkylation by artemisinin (which is specifically activated in high-haem, high-iron parasite conditions) could possibly explain both the specificity and potency of the drug. Nevertheless, it should be considered that certain proteins or other cellular targets could play a disproportionately large role in the downstream effects of artemisinin. The elucidation of such targets remains a challenge for further understanding and development of this important drug. Ultimately, the story of artemisinin's discovery and development is a classic example of the value held in traditional medicine and traditional literature. Considering the long history of TCM as well as other systems of traditional medicine, it would surely not be surprising that other hidden gems already described and used by our predecessors yet remain to be discovered.
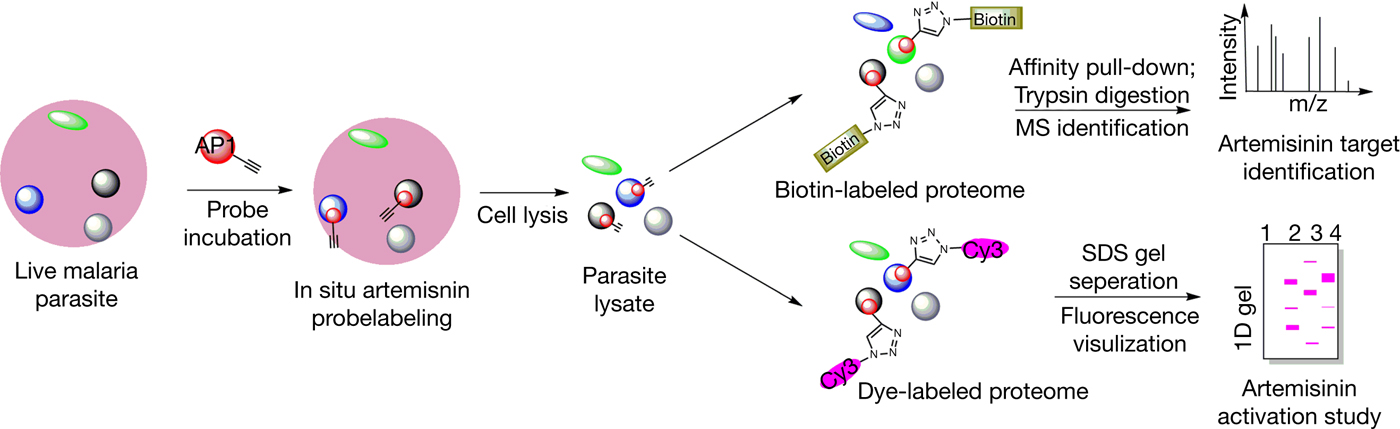
Figure 2. General workflow of a chemical biology approach to study the targets and activation of artemisinin using artemisinin-based chemical probes.

Figure 3. The proposed mechanism of action of artemisinin for its anti-malaria effects. Artemisinin is activated by haem which is released during haemoglobin digestion by the malaria parasite. This generates reactive radicals which alkylate a range of parasite proteins, eventually killing the parasite. Ca2+-ATPase (ATP6), the translationally controlled tumour protein (TCTP), ornithine aminotransferase (OAT), pyruvate kinase (PyrK), L-lactate dehydrogenase (LDH), spermidine synthase (SpdSyn) and S-adenosylmethionine synthetase (SAMS).
Arsenic trioxide in the treatment of acute promyelocytic leukaemia
Arsenic compounds, though well known for their toxicity, have been used for therapeutic purposes for possibly thousands of years. In accordance with the TCM principle of ‘attacking poison with poison’, the toxic compound arsenic trioxide (ATO, As2O3) or ‘Pi Shuang’ has been used both topically and orally in TCM as a medication for fevers, skin conditions and many other diseases (Ref. Reference Kwong and Todd64). In TCM as well as pre-modern Western medicine, ATO has also been used in the treatment of cancer, including leukaemia, and ATO was, in fact, a common treatment for chronic myeloid leukaemia in the early 20th century (Ref. Reference Jolliffe65). With the advent of more sophisticated cancer therapeutics including chemotherapy and the concerns of toxicity, however, the therapeutic use of ATO outside of China was eventually largely phased out (Ref. Reference Emadi and Gore66).
In 1996 and 1997, clinical and in vitro data from China reported by Shen et al. and Chen et al. were published in Blood detailing the application of ATO to the treatment of acute promyelocytic leukaemia (APL), drawing international attention to the compound which has already been in development in China for over a decade (Refs Reference Chen67, Reference Chen68, Reference Shen69). The efforts could be traced to the work of Dr Zhang Tingdong and colleagues at Harbin Medical University during the 1970s, who studied the first TCM-derived formulation of ATO in the treatment of leukaemia and identified ATO as the principal active component (Ref. Reference Rao70). APL itself was an acute and aggressive form of leukaemia that saw considerable improvement in treatment outcomes following the development of all-trans retinoic acid (ATRA) therapy, which was also developed and reported in the late 1980s by Chinese researchers (Refs Reference Huang71, Reference Wang and Chen72). However, ATRA treatment alone suffered from a high rate of relapse, necessitating novel and complementary therapeutic approaches (Ref. Reference Griffin73). The development of ATO for APL treatment by Zhang and others continued in China with promising clinical results, and the 1997 publications were followed up by Soignet et al. in their 1998 clinical report (Ref. Reference Soignet74). Much like artemisinin, the outstanding clinical properties of ATO led to rapid adoption and development by the international community. ATO in combination with ATRA therapy is currently a standard APL treatment, and the clinical success of the treatment in both newly diagnosed and relapsed cases has been well demonstrated and comprehensively reviewed in several authoritative works (Refs Reference Niu75, Reference Shen76, Reference Estey77, Reference Lo-Coco78, Reference Efficace79, Reference Zhang80, Reference Breccia and Lo-Coco81).
The core mechanisms of ATO in APL treatment are currently understood to be a concentration-dependent dual effect by the induction of cancer cell differentiation and apoptosis. In initial in vitro testing on APL NB4 cell lines, Chen et al. reported robust apoptosis induction by ATO at higher concentrations between 0.5–2 µm and partial differentiation at lower (0.1–0.25 µm) over longer time periods up to 10 days (Ref. Reference Chen67). ATO-induced apoptosis is mediated by the canonical mitochondrial pathway, inducing mitochondrial membrane potential collapse by targeting mitochondrial membrane proteins and demonstrating characteristic cytochrome c release, caspase 8 activation and cleavage of poly (ADP-ribose) polymerase (PARP) (Refs Reference Jing82, Reference Larochette83, Reference Kitamura84, Reference Cai85, Reference Belzacq86). Notably, ATO-induced apoptosis is associated with downregulated Bcl-2 expression and can be inhibited by Bcl-2 overexpression (Refs Reference Chen67, Reference Larochette83). Cellular redox conditions have also been shown to be important for ATO-induced apoptosis, possibly by interfering with the ability of ATO to bind protein effectors through interactions with exposed sulfhydryl (–SH) groups of cysteine residues (Refs Reference Emadi and Gore66, Reference Miller87). Accordingly, modulation of the antioxidant glutathione (GSH) system has been reported to affect cancer cell sensitivity to ATO (Ref. Reference Dai88). Other reported mechanisms of ATO-induced apoptosis include ROS production and oxidative damage through the modulation of ROS-related genes, inhibition of NF-κB through the binding of IκB kinase (IKK), and further effects on many other signalling pathways, including MAPK, JAK-STAT and JNK which have been extensively reviewed elsewhere (Refs Reference Breccia and Lo-Coco81, Reference Miller87, Reference Kapahi89, Reference Davison90).
The mechanisms by which ATO induces differentiation are less well understood but are likely related to its interactions with the PML–RARα fusion protein which is characteristic of the vast majority of APL cases. This oncoprotein is caused by a particular t(15;17) chromosomal translocation which results in a fusion of the retinoic acid receptor alpha (RARα) gene and promyelocytic leukaemia (PML) gene (Refs Reference de Thé91, Reference Dyck92). Endogenous PML is a tumour suppressor that localises to the nucleus and forms distinct macromolecular structures known as PML nuclear bodies (PML-NB) which are involved in transcriptional regulation (Refs Reference Wang93, Reference Melnick and Licht94). Functional PML-NB prevents the development of malignancy and is required for terminal differentiation. In the case of APL, expression of the PML-RARα fusion protein disrupts the normal PML-NB structure, resulting in errant protein-protein interactions, failure of differentiation and eventual leukemogenesis (Ref. Reference Wang and Chen72). Through the induction of ROS formation and direct interaction with PML–RARα, ATO is reported to restore endogenous PML-NB structure and function through the induction of PML–RARα multimerisation, SUMOylation and eventual proteasomal degradation (Refs Reference Zhu95, Reference Lallemand-Breitenbach96, Reference Jeanne97, Reference Zhang98). This ability of ATO to target a key oncogenic protein (as a so-called oncogene-directed therapy) underlies its remarkable specificity and efficacy. In this regard, the mechanism appears to be related to ATRA therapy (and hence the RAR pathway) which also induces differentiation and PML–RARα degradation, although ATO and ATRA are known to interact with different regions of the fusion protein (Refs Reference Zhu95, Reference Shao99). This distinction could partly explain the synergism between ATO and ATRA in combination, as well as the effectiveness of ATO in ATRA-resistant and relapsed cases (Ref. Reference Miller87). In addition to the restoration of PML-NB function and extensive effects on gene regulation and cancer cell stemness, other factors such as miRNA have also recently been reported to contribute to ATO-induced differentiation (Refs Reference Ghaffari100, Reference de Thé and Chen101).
Beyond ATO, the general study of TCM-derived natural products and compound formulations for cancer therapy is an area of immense interest both within and outside China. Apart from the ongoing discovery and characterisation of active antineoplastics, the use of TCM as an effective adjuvant therapy in combination with conventional treatments as well as the role of TCM in pain management and palliative care should also not be overlooked (Refs Reference Efferth102, Reference Youns103, Reference Li104, Reference Li105, Reference Chung106, Reference Chung107). For instance, the four-herb formulation PHY906 currently undergoing multiple clinical trials has been identified as an effective modulator of chemotherapy toxicity for multiple chemotherapy treatments (Refs Reference Liu and Cheng108, Reference Farrell and Kummar109, Reference Saif110). Given the recent resurgence of interest in natural products and the success of artemisinin and ATO in their respective roles, it is not difficult to imagine that more drugs and treatments of great potential remain within the TCM materia medica (Ref. Reference Cragg and Newman9). It will be of great interest moving forward to observe the potential contributions of TCM to evidence-based cancer therapy.
Berberine in the treatment of type 2 diabetes mellitus
The use of TCM for the prevention and treatment of diabetes in China dates back over 2000 years where diabetic symptoms were known as ‘Xiaoke’, or increased thirst (Ref. Reference Tong111). Many herbs and formulations have been developed and remain widely in use in China for TCM treatment of type 2 diabetes mellitus in the present day (Ref. Reference Li112). Among those treatments, the isoquinone alkaloid known as berberine (Fig. 4) has stood out for its clinically demonstrated hypoglycemic and hypolipidemic properties. Berberine is an active component of Coptis chinensis rhizomes, a Chinese herbal used for the relief of diabetes as well as gastrointestinal disorders (Ref. Reference Yin113). Traditionally known for a wide spectrum of antimicrobial activities, the application of berberine to diabetic symptoms was first reported and followed up in a Chinese study in the late 1980s, when berberine used in an antidiarrhoeal capacity for diabetic patients was shown to lower blood sugar (Refs Reference Chen and Xie114, Reference Ni115).

Figure 4. The structure of berberine.
Compared with well-established treatments such as artemisinin or ATO, clinical study of berberine as an antidiabetic is at a relatively early stage with pilot trials based in China first being reported in 2008. In comparative studies between berberine and the first-line treatment metformin in 36 newly-diagnosed type 2 diabetics, Yin et al. reported comparable effects on the regulation of glucose metabolism with significant (P < 0.01) decreases in indicators such as haemoglobin A1c, fasting blood glucose and postprandial blood glucose. Berberine outperformed metformin in the regulation of lipid metabolism, effecting a decrease in plasma triglycerides and cholesterol over 13 weeks at a significant improvement over metformin (P < 0.05). In parallel, a study was conducted on the use of berberine in combination therapy with existing antidiabetic treatment for 48 patients with poorly controlled diabetes. The use of berberine significantly improved both glycemic and lipidemic parameters compared with baseline, and treatments were generally well tolerated with limited and transient adverse gastrointestinal reactions in the combination group but no functional liver or kidney damages (Ref. Reference Yin116). In a double-blind randomised controlled trial in 110 patients published in the same year, Zhang et al. likewise reported the efficacy and safety of berberine in the treatment of newly diagnosed type 2 diabetics. Both glycaemic and lipidaemic indicators exhibited significant improvements compared to the placebo, and secondary outcomes including reduced body weight and blood pressure were also positive (Ref. Reference Zhang117).
As reviewed in meta-analyses published 2012 and 2015, subsequent randomised clinical trials (14 and 27 studies reviewed respectively) continued to provide positive results, with significant support for the efficacy of berberine in improving glycaemic control that is at least comparable or not inferior to current first-line treatments (Refs Reference Dong118, Reference Lan119). Considering that berberine is chemically distinct from other first-line options, the compound stands out as a potential novel antidiabetic both as monotherapy or in combination with other treatments for cases which respond poorly to existing treatments. Other plus points for berberine include a low cost of production, safety and a multi-faceted mechanism that appears to target multiple aspects of diabetes (Refs Reference Lan119, Reference Pang120). Berberine has been reported to target glucose metabolism through both insulin-dependent and independent pathways, increasing insulin sensitivity (partly through increased insulin receptor expression), insulin secretion, glucose uptake, and stimulating activation of the AMP-activated protein kinase (AMPK) pathway (Refs Reference Yang121, Reference Kong122, Reference Lee123, Reference Yin124). Other mechanisms including modulating liver metabolic function through regulation of gene expression, reduction of intestinal glucose uptake through the inhibition of α-glucosidase, modulation of gut microbiota composition (through antimicrobial activity) and antioxidant properties have also been reported, among others (Refs Reference Pan125, Reference Han126, Reference Zhou and Zhou127, Reference Ni128). In contrast, the antihyperlipidemic efficacy and mechanism of berberine are comparatively less understood and will require additional study. Importantly, the compound suffers from relatively poor bioavailability and novel formulations to improve its pharmacological profile will be of interest (Ref. Reference Vuddanda129). Further clinical and mechanistic studies, especially high-quality and unbiased clinical trials, will be crucial for the continued development of TCM-derived antidiabetic treatments such as berberine as well as other promising formulations (Refs Reference Ji130, Reference Lin131). In any case, the development of TCM for both the management and prevention of diabetes remains widespread and an ongoing subject of great research interest in China, and should be worthwhile of continued observation for contemporary applications.
Salvia miltiorrhiza in the treatment of cardiovascular diseases
Cardiovascular and cerebrovascular diseases, especially ischemic heart disease and stroke, remain consistently among the leading causes of death worldwide. In China, the use of TCM medication and principles in treating such conditions is among the most developed fields in TCM practice and research. Generally termed ‘huo xue hua yu’ or ‘activating blood circulation and removing blood stasis’, this branch of TCM makes use of a wide range of herbs and formulations to achieve antithrombotic, antiplatelet aggregation, vasodilative and cardioprotective effects (Ref. Reference Liu132). Among the many Chinese medicines used for such purposes, the root of the S. miltiorrhiza or ‘Danshen’ is particularly known in China as well as other parts of Asia for its historical and contemporary usage in the treatment of many cardiovascular and cerebrovascular diseases (Ref. Reference Ji133). Remarkably, a complex formulation of Danshen, the Compound Danshen Dripping Pill, holds the distinction of being the first TCM-derived product that was approved in 1997 for phase II clinical trials by the US Food and Drug Administration (FDA) (Ref. Reference Lei134). Phase II trials for the application of the product (trademarked ‘Dantonic’) for the prevention and treatment of stable angina were completed with positive results in 2010 (Ref. Reference Lei134), and phase III trials were completed in 2016. The Compound Danshen Dripping Pill, Danshen products in other forms including Danshen-based drug injections have been the subject of extensive clinical and mechanical study in China. Positive clinical findings have been reported for the application of Danshen-based medication for ischaemic stroke, angina pectoris, intercranial haematoma and acute myocardial infarction, among others (Refs Reference Sun135, Reference Liao136, Reference Wang137, 138). In particular, 16 out of 16 published meta-analyses on the use of Compound Danshen Dripping Pills for the management of coronary heart disease have reported positive findings on efficacy and safety, although additional high-quality and unbiased data remains necessary for further judgment (Ref. 138).
This recognition of Danshen and Danshen-derived formulations by the FDA is noteworthy due to an important distinction between Danshen and other previously recognised TCM-derived drugs. Unlike artemisinin, ATO or even berberine, which are clearly defined singular compounds purified from some herb, mineral or mixture, Danshen extracts and compound formulations contain multiple bioactive components with complex mechanisms of action which is characteristic of most medicinal herbs (Ref. Reference Wang139). This property is conventionally considered undesirable in modern rational drug design, which values well-defined molecular targets and mechanisms for each drug. This represents a contrast in ideology compared to Chinese medicine, which instead focuses on a holistic approach based on the overall symptoms and presentation of each individual patient and uses complex mixtures of herbs and other forms of treatment (Ref. Reference Jiang6). Compared with previous examples of successful drugs developed from Chinese medicine, the fact that the complex formulation of Danshen-based medication appears to also be the most effective is perhaps indicative that the Chinese philosophy can also be valid, at least on occasion (Refs Reference Chan140, Reference Li141).
To date, at least 50 components have been purified and identified from Danshen extracts, which can be largely categorised into hydrophilic compounds (including various polyphenolic acids) and lipophilic compounds (mostly from the tanshinone family of diterpenes) (Ref. Reference Wang139). Several of these are relatively well-studied and believed to contribute significantly to the pharmacological effects of Danshen. The phenolic acids including Danshensu, rosmarinic acid, caffeic acid and the salvianolic acids A and B have been reported to exhibit antioxidant, anticoagulant, vasodilative and cardioprotective effects, in addition to other biological activities including antineoplastic and hepatoprotective effects (Refs Reference Wang139, Reference Zhou142). In particular, Danshensu (salvianic acid A) and salvianolic acid B have both been proposed as major contributors to the vasodilative and anticoagulant effects of Danshen extracts, acting through the modulation of calcium influx and the immune response, among other mechanisms (Refs Reference Zhang143, Reference Yu144, Reference Ho and Hong145, Reference Zhou146). Among the lipophilic compounds, the tanshinones which are mostly unique to the Salvia family (represented by tanshinone I, tanshinone IIA and cryptotanshinone) are well-studied and have been shown to exhibit significant antibacterial, antiinflammatory and antioxidant properties in addition to cardioprotective effects (Ref. Reference Wang139). Outside these comparatively well-understood compounds, other constituents of Danshen have also been reported to contribute to the overall pharmacological effects of Danshen through multiple mechanisms including calcium modulation and the Akt–eNOS pathway (Refs Reference Sun147, Reference Lam148, Reference Ren-an149). The chemical and biological properties of the prominent constituents of Danshen-based medicines are comprehensively reviewed in other authoritative publications, including a recent comprehensive characterisation of Danshen-based injection by mass spectrometry (Refs Reference Wang139, Reference Li141, Reference Zhou142, Reference Cheng150). Despite the volume of available research, much remains to be understood with regards to the less-understood constituents and the interactions between the various active components of Danshen. Nevertheless, it appears evident that a combination of many bioactive components is necessary for the full efficacy of Danshen and other such herbal formulations. Pending the results of current and further clinical study, TCM-derived treatments of cardiovascular disease exemplified by Danshen should continue to be an area of great interest.
Conclusion
Evidence-based TCM and the integration of TCM principles and medication with modern science and medicine is an area of tremendous ongoing interest and effort. As an overview of the contributions of the entire TCM practice to modern medicine, we have attempted to select several representative treatments in various stages of development to illustrate the progress that has been made in each respective field. However, it is important to reiterate that this is only a tiny representation of the available data and the ongoing effort in TCM research extending to a wide range of diseases. Where possible, we have included references to comprehensive and authoritative reviews that cover the respective fields in great detail and rigor.
One of the means by which TCM contributes to world medical development is to provide effective monomer chemical drugs. TCM is characterised by individual adjustment of multiple components and multiple targets which enables the body to transform from an abnormal to normal state. More research in this respect is yet to be carried out. Moving ahead, it will be important not only to appreciate the existing successes and the potential of TCM for contributing to modern medicine but also to work towards better realising this potential. Given the widespread nature of TCM usage in China for all manners of diseases, it is unsurprising that clinical data are often available in large abundance. However, the quality of clinical studies in terms of study methodology and the elimination of bias have remained significant areas of concern for TCM clinical research (Ref. Reference Li151). High-quality clinical data in conformance with international standards such as the CONSORT and TREND statements are absolutely essential for the further development and acceptance of TCM, and the improvement of clinical data quality will be a critical step for TCM research looking forward. At the same time, the advancement of ‘omics’ and high-throughput investigative techniques such as proteomics and genomics have led to significant progress in drug target identification and the elucidation of drug mechanisms, which remain crucial to modern rational drug design (Refs Reference Ziegler152, Reference Wang153). Considering the complex, multi-component nature of most TCM herbal treatments, the application of such investigative techniques to more complex formulations should yield great insights not only to drug mechanisms but also to the understanding of TCM principles and philosophies with the potential to greatly contribute to modern medicine. While certain aspects of TCM may remain as fringe topics in the context of modern medicine, the examples covered in this review have hopefully served to illustrate the potential within TCM herbals and by extension the practice of TCM as well as traditional medicinal systems as a whole. It would be a mistake to completely discount the value of experience accumulated over thousands of years based on specific aspects of the practice. The continued application and integration of scientific principles and techniques with traditional medicine will surely continue to serve modern medicine well.
Acknowledgements
This work was supported, in whole or in part, by the projects of National Natural Science Foundation of China (grant numbers 81641002 and 81473548); Major National Science and Technology Program of China for Innovative Drug (grant no. 2017ZX09101002-00X-00X); and the Fundamental Research Funds for the Central public welfare research institutes (grant no. ZZ10-024).
Competing interests
The authors declare that they have no competing interests.