Environmental exposures can alter maternal physiology in a manner that results in “programming” effects on the fetus. This programming is understood in terms of adaptations to the prenatal environment, which may or may not be beneficial after birth. That is, the course of fetal development is altered as a function of critical environmental conditions in a manner that shapes growth and health outcomes into adulthood. According to Hochberg et al. (Reference Hochberg, Feil, Constancia, Fraga, Junien, Carel and Albertsson-Wikland2011) and others, offspring faces risk in the form of vulnerability to adversity and/or a lack or resilience if in utero programming is discordant with the postnatal environment. This programming effect was first noted in the context of undernutrition and is often referred to as Barker's hypothesis. Barker and Osmond (Reference Barker and Osmond1986) relied on epidemiological data to ascertain that gestational undernutrition was correlated with adult onset heart disease. Multiple investigations have since identified fetal programming contributions to adult disorders (e.g., Tobi et al., Reference Tobi, Goeman, Monajemi, Gu, Putter, Zhang and Heijmans2014), and interest in developmental origins of health and disease (DOHaD; Wadhwa, Buss, Entringer, & Swanson, Reference Wadhwa, Buss, Entringer and Swanson2009) continues to grow. This research encompasses prenatal effects associated with exposure to stress, toxicants, substance use/psychotropic medication, and nutrition. Programming effects of these environmental factors linked with temperament development will be considered in the present review, focused on the underlying biological mechanisms.
Fetal programming is thought to transform the structure and function of tissues/organs, shaping physiological outcomes associated with adult disease. Biological processes underling these programming effects represent a more recent emphasis, as DOHaD research has become increasingly concerned with the role of epigenetic mechanisms that alter gene expression (Hochberg et al., Reference Hochberg, Feil, Constancia, Fraga, Junien, Carel and Albertsson-Wikland2011). Understood to mediate the effects of environmental input on the developing organism by shaping gene expression, epigenetics has been defined as “molecular factors/processes around the DNA that regulate genome activity independent of DNA sequence, and are mitotically stable” (Skinner, Reference Skinner2014). Epigenetic mechanisms play a critical role in fetal programming, providing the basis for either healthy outcomes or disruption of physical and mental health. Environmental epigenetics encompasses a variety of environmental factors that serve to establish and maintain epigenetic modifications, influencing gene expression and the resulting phenotype. Fetal programming associated with maternal stress, toxicants, substance/psychotropic medication use, and nutrition reflects diverse biological pathways. All these exposures are nonetheless relevant to environmental epigenetics, as epigenetic processes provide conduits for conferring their effects to the gestating organism.
Consequences of fetal programming in humans have been documented most widely for physical health/medical outcomes (e.g., Roseboom et al., Reference Roseboom, Painter, de Rooij, van Abeelen, Veenendaal, Osmond and Barker2011), with behavioral effects often examined in animal models (Babenko, Kovalchuk, & Metz, Reference Babenko, Kovalchuk and Metz2015). Outcomes have not been typically framed as temperament per se, but rather discussed in terms of stress reactivity, susceptibility to anxiety, or impulsivity, without making links to this overarching theoretical framework. Connecting these disparate findings under the umbrella of the psychobiological model of temperament makes it possible to view these in terms of their implications for social–emotional development and developmental psychopathology, and to provide prevention/intervention related recommendations. Understanding developmental origins of temperament via fetal programming potentiated through epigenetic mechanisms could make earlier preventative efforts possible. Considering prenatal effects of maternal stress, toxicant exposure, substance/psychotropic medication use, and nutrition in the context of the environmental epigenetics framework provides an opportunity to compare resulting offspring epigenetic and behavioral signatures. These exposures often work in tandem (e.g., inadequate nutrition coupled with stress), and advances in environmental epigenetics will inform whether similar epigenetic mechanisms, overlapping genomic regions, and/or interacting gene networks are responsible for compound effects.
This review begins with epigenetic mechanisms critical to current research, and those likely to gain importance going forward. Links between temperament and developmental psychopathology are addressed next. We then turn to environmental factors that shape the intrauterine milieu and fetal development: stress, toxicant, substance use/psychotropic medication, and nutritional exposures. Finally, evidence linking DNA methylation and temperament, and implications for behavioral/emotional health are described, followed by conclusions and recommendations for future research.
Epigenetic Mechanisms
A number of epigenetic mechanisms have been identified, including DNA methylation, histone modifications, chromatic structure/remodeling, and noncoding RNAs (Figure 1).
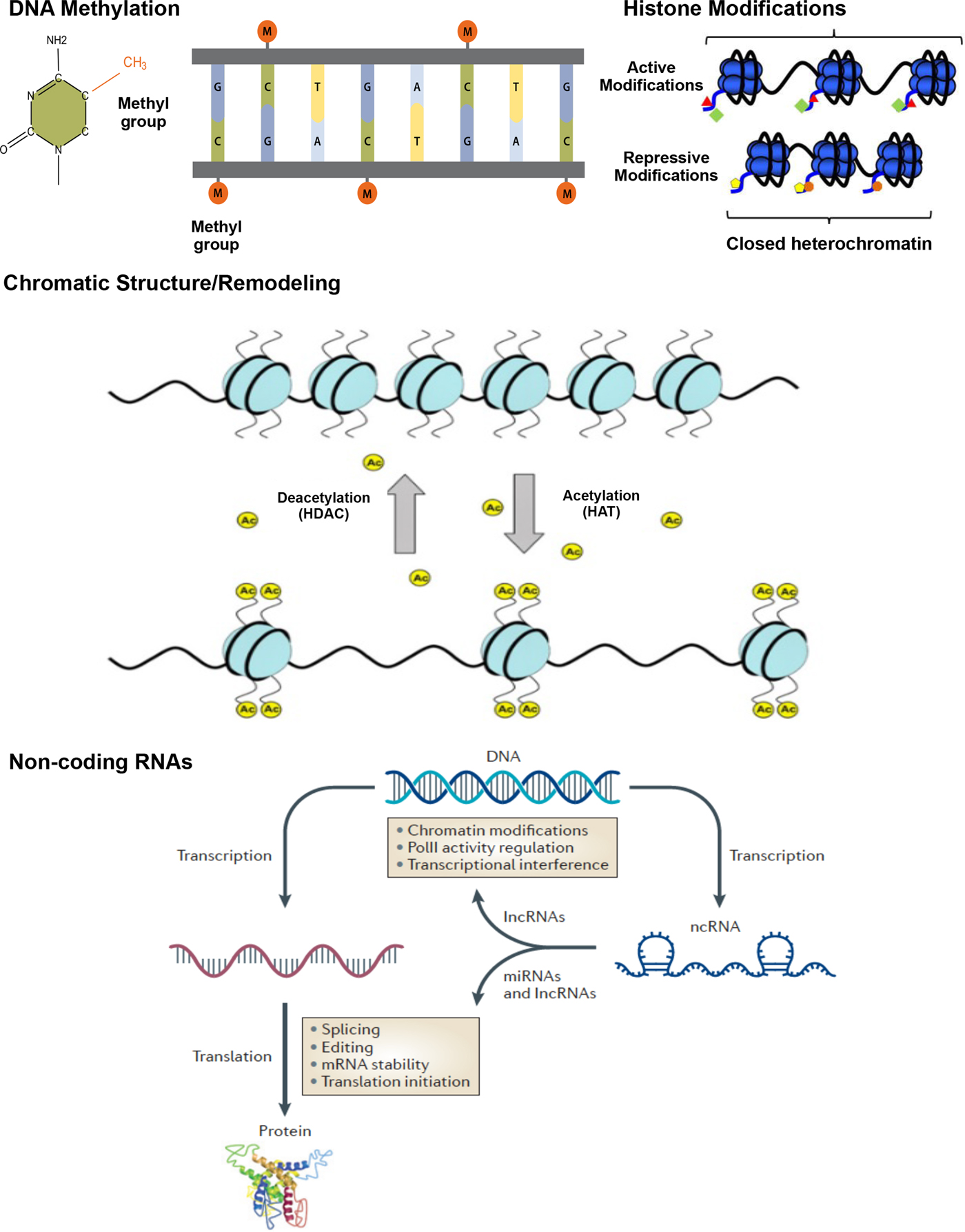
Figure 1. (Color online) Epigenetic mechanisms: DNA methylation, histone modification, and noncoding RNA. Methylation: DNA methylation is the addition of a methyl group (M) to the DNA base cytosine (C) in a CpG sequence; CH3, the methyl group, is added to a carbon of the cytosine ring via a covalent bond, resulting in 5-hydroxymethyl cytosine. Chromatic structure/remodeling: Chromatin structure in heterochromatin states, silencing transcriptional activities, euchromatin HAT catalyzes histone tails leading to active transcription. Histone modifications: histone modifications occurs via different enzymes. Active modifications result in permissive effects promoting transcription. Repressive modification marks serve to halt transcriptional activity in the gene, resulting in closed heterochromatin. Histone deacetylation (HDACs) and acetylation (HAT) represent repressive and permissive modification marks, respectively. Acetylation refers to an introduction of an acetyl functional group (Ac) into a chemical compound, whereas deacetylation reflects the removal of an acetyl group. Noncoding RNAs: noncoding RNAs perform a variety of functions related to gene expression. Long noncoding RNAs (lnc RNAs) can interfere in transcription and affect chromatin stability, also playing a role in translation. Noncoding RNAs can directly bind to promoters and interfere with polymerase II (PolII), one of three nuclear, DNA-dependent RNA polymerases. Posttranscription, microRNAs (miRNAs) are able to regulate gene expression by editing, splicing, and affecting stability of mRNA and protein synthesis. This figure is based on previous literature (Bagga, Reference Bagga2012; Suter, Takahashi, Grove, & Aagaard, Reference Suter, Takahashi, Grove and Aagaard2013; Wahlestedt, Reference Wahlestedt2013).
DNA methylation
DNA methylation occurs when a small (methyl) chemical group is attached to the DNA base cytosine (C) in a cytosine nucleotide–phosphate–guanine nucleotide (CpG) sequence. Covalent modification of DNA by methylation of cytosine residues serves to alter transcription and genome activity. DNA methylation is responsible for silencing of transposable elements and pericentromeric repeats to ensure genome integrity (Chen, Tsujimoto, & Li, Reference Chen, Tsujimoto and Li2004; Kaneko-Ishino & Ishino, Reference Kaneko-Ishino and Ishino2010; Xu, Bai, Collins, & Ghishan, Reference Xu, Bai, Collins and Ghishan1999), inactivation of the X-chromosome (Lock, Takagi, & Martin, Reference Lock, Takagi and Martin1987; Sado, Okano, Li, & Sasaki, Reference Sado, Okano, Li and Sasaki2004), and genomic imprinting (Feil & Khosla, Reference Feil and Khosla1999; Reik, Collick, Norris, Barton, & Surani, Reference Reik, Collick, Norris, Barton and Surani1987). Critical to multiple developmental outcomes, DNA methylation is also emerging as important for temperament development (e.g., Fuemmeler et al., Reference Fuemmeler, Lee, Soubry, Iversen, Huang, Murtha and Hoyo2016).
Histone modifications
Histones represent the chief protein component of chromatin, functioning as beads around which DNA winds, and is compressed into nucleosomes. Histones, and their various modifications, or marks, play an important role in regulating gene expression. Covalent posttranslational modifications to histone proteins including methylation, phosphorylation, acetylation, ubiquitylation, and sumoylation impact gene expression by altering chromatin structure and/or recruiting histone modifiers. Whereas some result in transcription activation, others have a repressive effect, effectively shutting down transcription. Lysine acetylation, for example, results in more accessible chromatin and tends to be associated with active transcription. Conversely, deacetylases remove acetyl groups from the histones, allowing the DNA to be wrapped more tightly and repressing transcription activity. Histone methylation effects depend on the location and number of methyl groups involved, potentially leading to activation or repression of gene expression (Barski et al., Reference Barski, Cuddapah, Cui, Roh, Schones, Wang and Zhao2007). Exposure to adverse environments has been shown to result in alterations in histone acetylation and methylation status in animals (Weaver et al., Reference Weaver, Cervoni, Champagne, D'Alessio, Sharma, Seckl and Meaney2004). Specifically, histone acetylation of the glucocorticoid receptor, nuclear receptor subfamily 3, group C, member 1 (NR3C1) gene differentiated offspring born to high and low licking and grooming mothers along with DNA methylation.
Chromatin structure/remodeling
A number of factors come into play as DNA is packaged into progressively larger units, starting with the assembly of nucleosomes and concluding with mitotic condensation, resulting in chromosomes. A variety of factors (e.g., stage of cell life) affect chromatin state, resulting in either euchromatin or heterochromatin. The euchromatin, “beads-on-a-string” structure makes DNA more accessible, promoting transcription and gene expression. The euchromatin state is associated with activating histone marks and remodeling complex recruitment that facilitate transcription factor binding. Heterochromatin, in contrast, represents a repressed state with respect to gene expression, and is associated with repressive histone and DNA methylation.
Noncoding RNAs
Noncoding RNAs include short microRNAs, Piwi-interacting RNAs, and long noncoding RNAs, regulating genes translation/transcription and chromatin stability (Zhou, Hu, & Lai, Reference Zhou, Hu and Lai2010). Noncoding RNAs can directly bind to promoters and interfere with polymerases by mobilizing transcriptionally repressive complexes conducive to heterochromatin (Zaratiegui, Irvine, & Martienssen, Reference Zaratiegui, Irvine and Martienssen2007) and through posttranscriptional binding (Filipowicz, Bhattacharyya, & Sonenberg, Reference Filipowicz, Bhattacharyya and Sonenberg2008). Noncoding RNAs are involved in a number of activities, with current evidence suggesting the largest class may be that of chromatin modifiers (Horabin, Reference Horabin2013). Whereas some noncoding RNA actions are sequence dependent, and thus not epigenetic in nature, examples of sequence-independent effects have been reported (Cao, Reference Cao2014).
Although a number of epigenetic mechanisms have been documented, initial implementation in DOHaD research has focused primarily on methylation. Additional epigenetic processes will likely be implicated in the future, likely working in concert, for example, as histone modifications contribute to chromatin remodeling (Figure 1). Accumulating evidence from animal studies supports the important role of epigenetic mechanisms in shaping physiological maturation and behavioral development, conferring effects of various exposures (e.g., Skinner, Anway, Savenkova, Gore, & Crews, Reference Skinner, Anway, Savenkova, Gore and Crews2008). The human literature lags behind and is subject to greater methodological challenges. Nonetheless, epigenetic effects have been identified utilizing brain tissue and peripheral cells (Nieratschker et al., Reference Nieratschker, Massart, Gilles, Luoni, Suderman, Krumm and Szyf2014; Szyf & Bick, Reference Szyf and Bick2013). That is, signatures of environment triggers that lead to epigenetic/molecular changes in the brain can also be identified in peripheral tissues, because of systemic consequences of exposure (Lester, Conradt, & Marsit, Reference Lester, Conradt and Marsit2016; Szyf & Bick, Reference Szyf and Bick2013), and afford identification in humans. Moreover, issues around cell specificity of epigenetic programming can be addressed by comparative studies, wherein human peripheral cell epigenetic signature is compared to a brain tissue signature obtained from animals, with the focus on conserved genomic regions and/or gene networks (Nieratschker et al., Reference Nieratschker, Massart, Gilles, Luoni, Suderman, Krumm and Szyf2014). This environmental epigenetics review will focus on stress, toxicant, substance use/pharmaceutical, and nutritional prenatal exposures. Pathways that involve maternal physiology and offspring brain development will be discussed as the foundation for temperament and risk/protection with respect to psychopathology. Epigenetic mechanisms mediate environmental effects on temperament phenotypes. Effects set in motion during gestation likely contribute to developmental psychopathology by impacting temperament growth and could also play a role as risky temperament profiles transform into symptoms and disorders (e.g., infant fear/behavioral inhibition culminate in childhood social anxiety). Temperament-related effects are likely to be especially salient in early childhood, as behavioral attributes “come online.”
Temperament Development and Symptoms of Psychopathology
We now turn to temperament research addressing negative emotionality, surgency/extraversion, regulatory capacity/effortful control, and links with developmental psychopathology. The study of temperament has a long-standing history. Childhood research began with the work of Thomas and Chess (Reference Thomas and Chess1977) focused on “difficult temperament,” which reflects high levels of negative affects, coupled with few manifestations of positive emotions and poor regulation. A number of temperament definitions and frameworks have been proposed, several emphasizing biological underpinnings. The study of behavioral inhibition and exuberance, for example, has linked approach and avoidance tendencies to an asymmetry in frontal brain activation (Hane, Fox, Henderson, & Marshall, Reference Hane, Fox, Henderson and Marshall2008). The polyvagal theory (Porges, Reference Porges2007) addressed how physiological states support different classes of behavior (e.g., vagal withdrawal supports mobilization, fight, and flight) and primary emotions are related to autonomic functioning. Currently, Rothbart's psychobiological model (Rothbart & Derryberry, Reference Rothbart, Derryberry, Lamb and Brown1981), an integration of existing temperament and personality frameworks with other relevant areas, such as neuroscience and comparative studies, represents the most widely referenced approach.
According to the psychobiological model, temperament represents constitutionally based individual differences in emotional and motor reactivity, as well as self-regulation, demonstrating consistency across situations and relative stability over time (Rothbart & Derryberry, Reference Rothbart, Derryberry, Lamb and Brown1981). Emotional reactivity encompasses fear, anger, sadness, and positive emotions. Processes serving to modulate reactivity, including attentional focus, shifting, and inhibitory control, provide the basis for self-regulation. The psychobiological approach identifies unique domains of temperament, mapping these onto underlying neurobehavioral systems, and outlines their developmental pathways and interactions. The term “constitutional” emphasizes the connection between temperament and biology. Historically, individual differences in temperament have been linked to the constitution of the organism, as it was understood at the time. Although “constitutionally based” has been typically interpreted as genetically driven, accumulating evidence suggests epigenetic processes make important contributions and have been incorporated in the definition of temperament more recently (Putnam & Gartstein, Reference Putnam and Gartstein2016). The psychobiological temperament framework applies across the life span, with reactive and regulatory domains developing especially rapidly in early childhood. Temperament attributes are understood to encompass physiological reactivity, including elements of the hypothalamus–adrenal–pituitary (HPA) axis response and patterns of brain activation. These are generally measured along with behavioral markers, typically ascertained through direct observations, and in humans also via caregiver report (Gartstein, Putnam, Aaron, & Rothbart, Reference Gartstein, Putnam, Aaron, Rothbart and Matzman2016).
Structurally, temperament encompasses negative emotionality, surgency/extraversion, and orienting regulation/effortful control. Distress proneness is the first to emerge (e.g., Putnam, Rothbart, & Gartstein, Reference Putnam, Rothbart and Gartstein2008; Rothbart, Reference Rothbart, Kohnstamm, Bates and Rothbart1989). At the same time, negative emotionality has strong conceptual and empirical links to the adult personality trait of neuroticism (Evans & Rothbart, Reference Evans and Rothbart2007). Fearful reactivity/behavioral inhibition, a component of negative emotionality, tends to occur in the context of relative right-hemisphere dominance (right dorsolateral prefrontal cortex and temporal regions), associated with limbic system (i.e., amygdala, basal ganglia, and hypothalamus) activation (Davidson, Reference Davidson1998; Sutton & Davidson, Reference Sutton and Davidson1997). HPA axis functioning alterations marked by higher cortisol were also noted for children demonstrating behavioral inhibition/fear (Perez-Edgar, Schmidt, Henderson, Schulkin, & Fox, Reference Perez-Edgar, Schmidt, Henderson, Schulkin and Fox2008).
Surgency in infancy is largely manifested through displaying pleasure (e.g., smiling and laughing) and approach behaviors (Gartstein & Rothbart, Reference Gartstein and Rothbart2003; Rothbart, Reference Rothbart, Kohnstamm, Bates and Rothbart1989), incorporating characteristics of activity and enthusiasm (Rothbart & Ahadi, Reference Rothbart and Ahadi1994). Individuals higher in positive affect have the tendency to be engaged, rather than disengaged, with their environment (Lonigan, Phillips, & Hooe, Reference Lonigan, Phillips and Hooe2003), as approach tendencies are potentiated by increased behavior activation system input (Gray, Reference Gray, van Goozen, Van de Poll and Sergeant1994; Rothbart & Hwang, Reference Rothbart, Hwang, Elliot and Dweck2005). The corticolimbic–striatal–thalamic network that operates via dopaminergic projections from the ventral tegmental area to the subcortical and frontal cortical regions (Depue & Collins, Reference Depue and Collins1999) favors left frontal regions (Coan & Allen, Reference Coan and Allen2004) and provides the foundation for surgency/extraversion. In infancy, relative left frontal asymmetry was associated with approach behaviors, including positive vocalization and facial expressions of joy (Diaz & Bell, Reference Diaz and Bell2012; Hane et al., Reference Hane, Fox, Henderson and Marshall2008).
Infant regulatory/attentional skills set the stage for the more flexible, frontally mediated effortful control (e.g., Gartstein, Bridgett, Young, Panksepp, & Power, Reference Gartstein, Bridgett, Young, Panksepp and Power2013). The emergence of effortful control coincides with rapid development of the executive attention system, including the lateral prefrontal cortex and anterior cingulate regions (Rothbart, Derryberry, & Posner, Reference Rothbart, Derryberry, Posner, Bates and Wachs1994; Rueda, Reference Rueda, Zentner and Shiner2012). This critical milestone involves a shift from reliance on the alerting attention network, supported by parietal lobe areas and governed by the norepinephrine system, to a more flexible executive attention network. Anterior cingulate, basal ganglia, and areas of the prefrontal cortex are included in the executive attention network, modulated by dopamine (Posner, Rothbart, Sheese, & Voelker, Reference Posner, Rothbart, Sheese and Voelker2012). Whereas the alerting system is responsible for filtering sensory input, the executive network is engaged in conflict resolution and selecting between alternative response options (Posner & Peterson, Reference Posner and Peterson2011).
A growing body of research indicates that the risk for externalizing problems (conduct difficulties, hyperactivity, and impulsivity; Campbell, Reference Campbell1995; Kovacs & Devlin, Reference Kovacs and Devlin1998) is greatest for highly reactive children with pronounced negative emotionality and/or surgency, coupled with deficits in attention and regulatory functioning (Diaz et al., Reference Diaz, Eisenberg, Valiente, VanSchyndel, Spinrad and Southworth2015; Gartstein & Fagot, Reference Gartstein and Fagot2003; Martel, Gremillion, & Roberts, Reference Martel, Gremillion and Roberts2012; Muris & Ollendick, Reference Muris and Ollendick2005). Internalizing difficulties that involve personal distress and are affective in nature (e.g., depression/anxiety) can occur as a function of high negative emotionality and low surgency (Fowles, Reference Fowles1994; Lonigan et al., Reference Lonigan, Phillips and Hooe2003; Murray & Kochanska, Reference Murray and Kochanska2002), with nontemperament pathways also relevant for depressive symptoms (Hammen, Reference Hammen2005).
Thus, early manifestations of temperament not only are important as key components of social–emotional development but also are significant as early markers of psychopathology risk (Figure 2). A number of explanations for the relationship between temperament and psychopathology have been proposed. Vulnerability and pathoplasty models generally assign temperament a causal role in psychopathology. Vulnerability explanations cast temperament as increasing the probability of symptom onset (e.g., Perez-Edgar et al., Reference Perez-Edgar, Schmidt, Henderson, Schulkin and Fox2008). In contrast, temperament and psychopathology can have independent etiology in pathoplasty models, wherein temperament (or personality) is viewed as impacting the course and/or severity of disorders (e.g., De Bolle, De Clercq, De Caluwe, & Verbeke, Reference De Bolle, De Clercq, De Caluwe and Verbeke2016). Alternative differential susceptibility (Belsky & Pluess, Reference Belsky and Pluess2009) and biological sensitivity to context (Boyce & Ellis, Reference Boyce and Ellis2005) models are relevant primarily to negative emotionality, viewed as a plasticity, rather than a vulnerability factor. Thus, the differential susceptibility/biological sensitivity to context models make “for better or for worse” predictions with respect to negative emotionality. There is growing empirical support for the positive response of those high in negative emotionality to good environments and interventions (e.g., Hentges, Davies, & Cicchetti, Reference Hentges, Davies and Cicchetti2015), and new evidence that negative affectivity primarily confers adjustment risk (e.g., Moran et al., Reference Moran, Lengua, Zalewski, Ruberry, Klein, Thompson and Kiff2016). Predictive links between temperament and symptoms were also framed in terms of underlying neurobiology. Whittle, Allen, Lubman, and Yucel (Reference Whittle, Allen, Lubman and Yücel2006) proposed a model focused on six brain structures: amygdala, hippocampus, nucleus accumbens, orbitofrontal cortex, anterior cingulate cortex, and the dorsolateral prefrontal cortex, describing joint effects for negative emotionality, surgency/extraversion, regulation, and related symptoms/disorders. Environmental epigenetics can elucidate biological underpinnings of temperament development, in turn expanding our understanding of developmental psychopathology and DOHaD more broadly, as temperament attributes represent key contributing factors.

Figure 2. Links between temperament attributes and developmental psychopathology. Depicted are the most prominent relationships between child temperament traits and broad symptom/behavior problem clusters (internalizing and externalizing). (–) A negative relationship and (---) a less well-established relationship.
Human and animal studies showing connections between prenatal environmental exposures and temperament-related phenotypes suggest these exposures, and the cascades of epigenetic effects that follow, impact how reactivity and regulation “come online.” Studies addressing the impact of maternal stress response and HPA axis functioning make some of the least ambiguous connections between prenatal exposure and alteration in offspring temperament across species, elucidating epigenetic processes that mediate prenatal environment–postnatal behavior relationships. Evidence linking maternal stress during pregnancy and offspring HPA axis dysregulation also suggests the latter likely manifests in reactive and regulatory components of temperament, as well as their contributions to symptoms/disorders (Figure 2).
Environment Effects That Shape Intrauterine Environment and Fetal Development
As environmental epigenetics concerns itself with a spectrum of exposures capable of exerting developmental effects, we now describe these, and established links with behavioral phenotypes relevant to temperament. Stress exposure has been one of the most widely studied prenatal adversities. Human and animal studies indicate behavioral consequences for the offspring typically involve fear/anxiety and/or behavioral/emotional dysregulation.
Stress: Mechanisms, Definitions, Critical Developmental Periods, and Epigenetic Effects
Stress response: The neuroendocrine system
In response to stress, paraventricular nucleus of the hypothalamus produces corticotropin-releasing hormone (CRH). CRH stimulates the anterior pituitary to synthesize proopiomelanocortin. Adrenocorticotropic hormone (ACTH), a peptide derived from proopiomelanocortin, is subsequently released into the bloodstream. ACTH induces the adrenal glands to produce cortisol in humans (corticosterone in rodents), the end product of the HPA axis, often labeled the stress hormone (Charmandari, Tsigos, & Chrousos, Reference Charmandari, Tsigos and Chrousos2005). A negative feedback loop is created as cortisol binds to glucocorticoid receptors in the hippocampus. This modulation of the HPA axis response results in downregulation of CRH and ACTH (Deppermann, Storchak, Fallgatter, & Ehlis, Reference Deppermann, Storchak, Fallgatter and Ehlis2014).
A mechanism wherein information from the environment is conferred to the offspring via maternal stress hormones during gestation appears to be conserved across multiple species (Table 1). For instance, predation risk was shown to cause female fish to produce larger eggs with higher concentrations of cortisol (Giesing, Suski, Warner, & Bell, Reference Giesing, Suski, Warner and Bell2011). Behavioral effects were also noted, as juveniles exposed to prenatal stress formed denser shoals, an effective antipredator strategy. In hares, in utero predator stress was positively correlated to offspring physiological stress reactivity, compromising subsequent reproductive fitness (Sheriff, Krebs, & Boonstra, Reference Sheriff, Krebs and Boonstra2009, Reference Sheriff, Krebs and Boonstra2010). High maternal cortisol concentrations were linked with nonviable births, smaller offspring size, increased cortisol reactivity, vigilance, and anxiety-like behaviors. Prenatally stress-exposed hares also dispersed more effectively, promoting juvenile survival at a cost of reproductive output, in an example of the “increasing glucocorticoid concentrations to survive at the cost of reproduction” strategy (Sheriff et al., Reference Sheriff, Krebs and Boonstra2009).
Table 1. Maternal neuroendocrine system response: Examples of effects on offspring development in animal and human studies

Note: Sample characteristics are provided in a different manner for human and animal studies, because of differences in design and presentation format. The number of participants per condition (n) is indicated for animal experimental investigations, whereas the overall number of participants (N) is presented for human correlational studies. Additional information concerning numbers of male versus female participants is also provided when both sexes were included/specified. Developmental timing of exposure indicators varies across studies. For humans, the timing is provided in terms of the number of gestational weeks. In animals these indicators are typically more specific, often presented in terms of the number of days. (↑) Increases in offspring physical/structural and functional characteristics; (↓) decreases in offspring physical/structural and functional characteristics.
Stress-related prenatal effects reflect a “calculation” aimed at improving the odds of survival and are not uniformly maladaptive. These adaptations are of interest precisely because of an attempt to balance the needs of the developing organism: the need for optimal development along a species-typical timeline versus faster development advantageous for immediate survival. The speeded-up gestation translates into alterations in cellular structure/function. Short-term benefits (i.e., faster gestation and survival) are self-evident, yet adverse health consequences in the long term are also likely. DOHaD research with humans suggests that gestational adversity, presumably resulting in fetal development shortcuts, translates into compromised health in adulthood, including mental health (e.g., Froehlich et al., Reference Froehlich, Lanphear, Auinger, Hornung, Epstein, Braun and Kahn2009; Roseboom, de Rooij, & Painter, Reference Roseboom, de Rooij and Painter2006).
The range of prenatal adversity is notably broader in humans, including stressful or life-threatening situations and maternal reactivity to these circumstances (e.g., posttraumatic stress disorder [PTSD]), signaled to the fetus by maternal HPA axis response (Babenko et al., Reference Babenko, Kovalchuk and Metz2015). Certain stressors can be considered normative, as these generally occur in the context of daily life. Even routine hassles can become problematic, however, especially in concert with symptoms of depression and anxiety (Diego et al., Reference Diego, Jones, Field, Hernandez-Reif, Schanberg, Kuhn and Gonzalez-Garcia2006). Although the majority of maternal cortisol (80%–90%) in the placenta is metabolized, higher concentrations of cortisol can disrupt this process, exposing the fetus to higher levels (Meyer, Reference Meyer1985). Increased maternal HPA axis activity and resultant cortisol production is thought to downregulate placental corticosteroid 11-β-dehydrogenase isozyme 2 (11β-HSD-2), which metabolizes cortisol into inactive cortisone (O'Donnell et al., Reference O'Donnell, Bugge Jensen, Freeman, Khalife, O'Connor and Glover2012). This reduced ability to increase placental 11β-HSD-2 with an acute stressor is likely critical to intergenerational transmission. That is, women who do not present with elevated cortisol during pregnancy, demonstrating a “blunted” response to chronic stress (Keeshin, Strawn, Out, Granger, & Putnam, Reference Keeshin, Strawn, Out, Granger and Putnam2014; Yehuda & Seckl, Reference Yehuda and Seckl2011), can nonetheless transmit exceedingly high levels of cortisol to the fetus with 11β-HSD-2 downregulation.
Prenatal stress exposure: Evidence of behavioral/temperament risk
Human conceptualizations of stress and empirical studies
Highly conceptual definitions of human stress evolved to include cognitive and allostatic processes, vulnerabilities that result from early experiences and impact adaptation (Maccari et al., Reference Maccari, Polese, Reynaert, Amici, Morley-Fletcher and Fagioli2016), yet research examining prenatal effects of stress has generally relied on unidimensional operational definitions. In fetal programming research, operationalizations of stress include traumatic events (e.g., exposure to interpersonal violence or natural disasters), psychosocial stress (e.g., daily hassles/stressful events), physiological stress reactivity (e.g., cortisol concentrations), and symptoms of anxiety and depression (Table 2). Multiple studies rely on broad definitions of stress, for example, combining indicators of psychosocial stress and symptoms of psychopathology (e.g., anxiety and depression; Nieratschker et al., Reference Nieratschker, Massart, Gilles, Luoni, Suderman, Krumm and Szyf2014). Thus, the literature does not currently provide the basis for making meaningful distinctions with respect to the type of stressor in terms of consequences for the offspring. Another challenge in examining stress with human populations is that exposures are often confounded, such as psychosocial/sociodemographic stress being accompanied by maternal substance use. Confounding effects are somewhat mitigated in quasi-experimental studies wherein stress response/adaptation data are collected in the aftermath of a disaster (Tees et al., Reference Tees, Harville, Xiong, Buekens, Pridjian and Elkind-Hirsch2010; Yong Ping et al., Reference Yong Ping, Laplante, Elgbeili, Hillerer, Brunet, O'Hara and King2015). This approach takes advantage of events that impact large numbers of individuals in a manner that is random with respect to potential confounds. However, stress in the aftermath of a disastrous event does not impact all affected to the same extent, and individuals subject to prior socioeconomic or psychosocial stress likely fare worse.
Table 2. Examples of in utero stress exposure effects: Temperament-related outcomes for human and animal studies

Note: Sample characteristics are provided in a different manner for human and animal studies, beause of differences in design and presentation format. For human correlational studies, the overall number of participants (N) is presented, whereas the number of participants per condition (n) is indicated for animal experimental investigations. Additional information concerning numbers of male versus female participants is provided, when both sexes were included/specified. Developmental timing of exposure indicators vary across studies. For humans, the timing is generally provided in terms of the number of gestational weeks, whereas in animals these indicators are typically more specific, counting embryonic days. (↑) Increases in temperament-related behaviors/displays and/or stress exposure; (↓) decreases in temperament-related behaviors/displays and/or stress; HPA, hypothalamus–pituitary–adrenal; PTSD, posttraumatic stress disorder; E, embryonic day; PND, postnatal day.
In one study making use of this quasi-experimental design, women exposed to the World Trade Center attacks during pregnancy were followed, along with their offspring. This research focused on PTSD and physiological stress reactivity in the wake of this human-made disaster (Yehuda, Reference Yehuda2002; Yehuda et al., Reference Yehuda, Engel, Brand, Seckl, Marcus and Berkowitz2005). Maternal PTSD symptoms were associated with lower infant cortisol concentrations, with most pronounced effects in their third trimester (Yehuda et al., Reference Yehuda, Engel, Brand, Seckl, Marcus and Berkowitz2005). Toddlers whose mothers were exposed to Iowa floods during pregnancy demonstrated increased cortisol reactivity to a separation. Higher subjective distress was linked with greater HPA axis reactivity for girls only, and more pronounced effects were associated with later term exposure (Yong Ping et al., Reference Yong Ping, Laplante, Elgbeili, Hillerer, Brunet, O'Hara and King2015). Prenatal stress effects were also noted earlier in gestation, with higher self-reported maternal anxiety at 12–22 weeks predicting child HPA axis alterations. Female offspring only exhibited depression in association with hyporeactivity (Van den Bergh, Van Calster, Smits, Van Huffel, & Lagae, Reference Van den Bergh, Van Calster, Smits, Van Huffel and Lagae2008). In the short term, stress is expected to produce an increased HPA axis response that translates into higher cortisol concentrations, yet the HPA axis downregulates after chronic periods of elevated stress (Gunnar & Vazquez, Reference Gunnar and Vazquez2001). Child stress reactivity is associated with negative emotionality (e.g., fear/behavioral inhibition; Perez-Edgar et al., Reference Perez-Edgar, Schmidt, Henderson, Schulkin and Fox2008), which can be measured via behavioral and physiological indicators (e.g., cortisol), and has been examined in conjunction with a spectrum of maternal prenatal symptoms.
A number of studies indicated associations between maternal anxiety and depression during pregnancy and infant negative emotionality as well as dysregulation. Mothers with higher prenatal emotional stress were more likely to have infants lower in fearfulness (Mohler, Parzer, Brunner, Wiebel, & Resch, Reference Mohler, Parzer, Brunner, Wiebel and Resch2006). This diminished fear was interpreted as potential behavioral risk, possibly contributing to externalizing disorders, such as attention-deficit/hyperactivity disorder (ADHD; Mohler et al., Reference Mohler, Parzer, Brunner, Wiebel and Resch2006). Maternal anxiety during pregnancy was associated with poor infant self-regulation, likely setting the stage for later behavioral and emotional difficulties (van den Heuvel, Johannes, Henrichs, & Van den Bergh, Reference van den Heuvel, Johannes, Henrichs and Van den Bergh2015). In the Davis et al. (Reference Davis, Glynn, Dunkel Schetter, Hobel, Chicz-DeMet and Sandman2007) study, prenatal anxiety and depression averaged across pregnancy were both significantly correlated with infant negative reactivity. Della Vedova (Reference Della Vedova2014) obtained somewhat divergent results controlling for socioeconomic and partner/relationship factors in predicting difficult temperament, a construct reflecting high negative emotionality. Prenatal depression and state anxiety in the postnatal periods emerged as independent predictors (Della Vedova, Reference Della Vedova2014).
Whereas late-term effects of stress exposure are thought to interfere with critical steps in fetal HPA axis development, early gestation effects likely result from cortisol impacting cell migration and interacting with additional pathways (e.g., serotonin signaling pathway) involved in brain development (Beijers, Buitelaar, & de Weerth, Reference Beijers, Buitelaar and de Weerth2014). Recent reviews have noted consistent links between exposure to prenatal stress and compromised neurodevelopment (e.g., neurogenesis; Antonelli, Pallares, Ceccatelli, & Spulber, Reference Antonelli, Pallares, Ceccatelli and Spulber2016; Fatima, Srivastav, & Mondal, Reference Fatima, Srivastav and Mondal2017). Stress-related fetal programming research in humans is limited by its correlational nature and reliance on quasi-experimental designs that inherently lack internal validity afforded by the animal models, with the latter serving to further inform these connections.
Animal models of prenatal stress exposure
Investigations of prenatal stress effects in animals provide an opportunity to directly manipulate key parameters (i.e., frequency, severity, and duration) of stress induction procedures, such as exposure to novel environments, subcutaneous injections of saline, restraint, and predator proximity. Injections of synthetic glucocorticoids have also been utilized to induce conditions that parallel high levels of physiological stress reactivity. These exposures impact the developing fetus, altering offspring neurobiology and behaviors into adulthood (Table 2).
A widely used restraint stress procedure resulted in anxiety-like behavior (decreased time spent in open arms of the elevated plus maze) for prenatally stressed males, with downregulation of glucocorticoid receptors described as a potential mechanism behind these effects and long-term HPA axis alterations (Maccari et al., Reference Maccari, Darnaudery, Morley-Fletcher, Zuena, Cinque and Van Reeth2003). Another prenatal stress paradigm involves the use of electric shock as an analogue for human trauma exposure (Golub, Kaufman, Campbell, Li, & Donald, Reference Golub, Kaufman, Campbell, Li and Donald2006). Prenatally stress-exposed offspring demonstrated increased duration and frequency of ultrasonic calls (sign of distress) during separation from mothers and spent less time in open arms of the elevated plus maze, compared to controls. Another stress induction manipulation relies on a forced swim test in the physical stress condition, with the psychological stress group observing those experiencing physically stressful conditions. Female, but not male, rat offspring exposed to both stress conditions demonstrated a decreased number of entrances into open arms of the elevated plus maze indicative of anxiety, relative to controls (Nazeri et al., Reference Nazeri, Shabani, Ghotbi Ravandi, Aghaei, Nozari and Mazhari2015). Abe at al. (Reference Abe, Hidaka, Kawagoe, Odagiri, Watanabe, Ikeda and Ishida2007) induced psychological stress in pregnant rats during the last trimester of gestation by having animals observe an electric shock administration procedure. In comparison with control animals, exposed male offspring showed enhanced emotionality in an open field test, depression-like behavior in a forced swim test, and increased HPA axis activity (higher basal plasma corticosterone levels). In nonhuman primates, administration of dexamethasone resulted in an exaggerated corticosterone response to a mild stressor (blood sampling) for the offspring of mothers who received the highest dose (de Vries et al., Reference de Vries, Holmes, Heijnis, Seier, Heerden, Louw and Seckl2007). In this paradigm, there is no environmental exposure to stress per se; however, the synthetic glucocorticoid administration is intended to mimic stress exposure effects, specifically HPA axis reactivity. Although this approach in a sense provides a “short cut” eliminating the need for an exposure manipulation, it does not reflect complexities of the physiological stress response, which may also involve dampening of HPA axis reactivity with prolonged exposure.
Thus, prenatal stress has been shown to significantly alter offspring development across human and animal studies, with a range of physiological and behavioral effects. Whereas animal studies suggest primarily anxiety-like behavioral effects, human outcomes were more varied, with some indication of risk for externalizing symptoms (Mohler et al., Reference Mohler, Parzer, Brunner, Wiebel and Resch2006). Prominent late-gestation effects were reported (e.g., Yehuda et al., Reference Yehuda, Engel, Brand, Seckl, Marcus and Berkowitz2005) and suggest disruption of fetal HPA axis development, yet early gestational impact of stress was noted as well (Golub et al., Reference Golub, Canneva, Funke, Frey, Distler, von Horsten and Solati2016). Crews (Reference Crews2010) argued neuronal and behavioral plasticity, or the genotype's ability to produce variable phenotypes in response to environmental inputs, peaks during embryonic development. Thus, early and later term gestational exposure to stress appears to impact behavioral phenotypes related to temperament, albeit via different developmental pathways. Sex differences were documented (e.g., Nazeri et al., Reference Nazeri, Shabani, Ghotbi Ravandi, Aghaei, Nozari and Mazhari2015); however, a consistent pattern has not emerged. Further study is required in part because females have not always been included. Epigenetic mechanisms are critical to connections between prenatal stress and subsequent behavioral/temperament development. We now turn to the emerging literature demonstrating epigenetic processes play a key role in the intergenerational transmission of stress-related risk, conferring environmental adversity to the offspring.
Prenatal stress exposure: Epigenetic effects
Human studies
Consistent with the environmental epigenetics perspective, multiple studies indicate that epigenetic processes, DNA methylation in particular, provide a critical bridge between prenatal stress exposure, offspring brain development, and shifts in physiology/behavior relevant to temperament. Maternal depression and anxiety during pregnancy were associated with increased infant methylation of NR3C1, which predicted higher infant salivary cortisol stress response (Oberlander et al., Reference Oberlander, Grunau, Mayes, Riggs, Rurak, Papsdorf and Weinberg2008). Maternal pregnancy-related anxiety was also positively associated with infant NR3C1 methylation (Hompes et al., Reference Hompes, Izzi, Gellens, Morreels, Fieuws, Pexsters and Claes2013). There is some indication of sex differences in the NR3C1 stress-related methylation shifts. Methylation levels were elevated as a function of prenatal stress for female, but not male, infants, and increased methylation was positively associated with fearfulness for females only (Ostlund et al., Reference Ostlund, Conradt, Crowell, Tyrka, Marsit and Lester2016). Methylation of the serotonin transporter gene (solute carrier family C6, member 4 [SLC6A4]) was also affected by neonatal intensive care unit-related stress. Increased methylation of the SLC6A4 gene was noted for preterm, compared to full-term, infants. SLC6A4 methylation status was negatively correlated with mother report of infant duration of orienting and approach for the preterm infants only (Montirosso et al., Reference Montirosso, Provenzi, Fumagalli, Sirgiovanni, Giorda, Pozzoli and Borgatti2016). However, NR3C1 and SLC6A4 are not the only genomic conduits of prenatal stress effects, and a recent review implicated altered expression of 31 genes in prenatal origins of anxiety-like behavior (Nieto, Patriquin, Nielsen, & Kosten, Reference Nieto, Patriquin, Nielsen and Kosten2016). Perhaps in the most definitive study to date, Nieratschker et al. (Reference Nieratschker, Massart, Gilles, Luoni, Suderman, Krumm and Szyf2014) examined the impact of prenatal stress on methylation in human infants, monkeys, and rats, utilizing a genome-wide approach and identifying conserved differentially methylated genes. Across species, 30 genes were associated with differential methylation as a result of prenatal stress. The protein coding the microrchidia homolog (mouse) family CW-type zinc finger protein 1 gene (MORC1) was emphasized as a candidate genetic marker of prenatal stress, due to its prior associations with major depressive disorder (Nieratschker et al., Reference Nieratschker, Massart, Gilles, Luoni, Suderman, Krumm and Szyf2014). Thus, most human research to date addressed methylation of candidate genes, and the only genome-wide study did not make any connections with behavioral outcomes, which remains to be accomplished in future investigations.
Animal studies
In animal studies, DNA methylation changes explained the influence of maternal prenatal stress on the density of glucocorticoid receptors in the fetal brain (hippocampus in particular), thought to alter sensitivity to stress throughout the life of the offspring (Babenko et al., Reference Babenko, Kovalchuk and Metz2015; Weaver et al., Reference Weaver, Cervoni, Champagne, D'Alessio, Sharma, Seckl and Meaney2004). Restraint stress during gestation resulted in altered CRH DNA methylation in the hypothalamus and increased anxiety-like behavior (e.g., decreased entries into open maze arm; Xu, Sun, Gao, Cai, & Shi, Reference Xu, Sun, Gao, Cai and Shi2014). Restraint stress during the last 10 days of gestation also resulted in anxiety-like behavior for adult offspring and altered RLN gene expression. Specifically, higher levels of DNA methylation in the promoter region were associated with decreasing mRNA expression in the cortex following exposure. The latter is significant because RLN is responsible for the protein Reelin, which regulates brain development through cytoskeleton modifications. Thus, DNA methylation appears to provide a critical bridge between prenatal stress, offspring brain development, and shifts in physiology/behavior relevant to temperament.
Toxicant Exposure: Pesticides and Environmental Contaminants
A similar picture emerges for toxicant in utero programming, wherein epigenetic processes link exposure to temperament outcomes. A variety of environmental contaminants, such as lead, dichlorodiphenyltrichloroethane (DDT), polychlorinated biphenyls (PCBs), and bisphenol A (BPA), have been investigated. As noted in a recent review, multiple animal studies and a more limited human literature suggest that prenatal toxicant exposure alters neurodevelopment, setting the stage for potentially risky temperament and symptoms/behavior problems (Antonelli et al., Reference Antonelli, Pallares, Ceccatelli and Spulber2016).
Endocrine disruptors (BPA, Vinclozolin, DDT, diethylstilbestrol [DES], PCB): Developmental effects
Endocrine disruptors are exogenous substances capable of dysregulating the endocrine system primarily by binding to hormone receptors, disrupting cell signaling pathways, and inhibiting hormone synthesis. These contaminants interfere with physical development, especially of the reproductive and central nervous systems, which translates into alterations in emotional functioning, attentional, and behavioral regulation components of temperament. Epigenetic mechanisms are critical to the profound effects of endocrine disruptors on developmental cascades, as exposure to these toxic agents introduces changes in gene expression (Manikkam, Guerrero-Bosagna, Tracey, Haque, & Skinner, Reference Manikkam, Guerrero-Bosagna, Tracey, Haque and Skinner2012). Existing studies with humans indicate consistent links between gestational endocrine disruptor exposure and behavioral shifts, albeit typically without addressing epigenetics.
Human studies
Prenatal BPA exposure and child behavior problems were linked, controlling for postnatal BPA effects, with a sex-dependent pattern of results (Perera et al., Reference Perera, Vishnevetsky, Herbstman, Calafat, Xiong, Rauh and Wang2012). Boys with greater exposure received elevated emotionally reactive and aggressive ratings (Table 3). Among girls, higher exposure was associated with significantly lower anxious/depressed and aggressive scores. Casas et al. (Reference Casas, Forns, Martínez, Avella-García, Valvi, Ballesteros-Gómez and Vrijheld2015) examined programming effects of prenatal BPA exposure and reported increased ADHD symptoms at 4 years of age.
Table 3. Examples of in utero toxicant exposure effects: Temperament-related outcomes in human and animal studies

Note: Sample characteristics are provided in a different manner for human and animal studies, because of differences in design and presentation format. For human correlational studies, the overall number of participants (N) is presented, whereas the number of participants per condition (n) is indicated for animal experimental investigations. Additional information concerning numbers of male versus female participants is provided, when both sexes were included/specified. Developmental timing of exposure indicators vary across studies. For humans, the timing is generally provided in terms of the number of gestational weeks, although in some instances measures are just taken at birth, or not specified beyond a particular trimester or month. For animals, these indicators are more specific, counting embryonic or postnatal days. (↑) Increases in temperament-related behaviors/displays; (↓) decreases in temperament-related behaviors; BPA, bisphenol A; ADHD, attention-deficit/hyperactivity disorder; DDT, dichlorodiphenyltrichloroethane; DES, diethylstilbestrol; PCB, polychlorinated biphenyls; E, embryonic day; PND, postnatal day.
Stronger associations were observed for boys, compared to girls, with greater third, rather than with first, trimester effects. These results suggest sex-specific mechanisms of BPA exposure may in part be determined by the timing of prenatal effects.
DDT is arguably the most widely used agricultural insecticide, with adverse health effects. Technical grade DDT includes a number of impurities and its breakdown product dichlorodiphenyldichloroethylene (DDE) is able to cross the placenta and pass into breast milk (You et al., Reference You, Gazi, Archibeque-Engle, Casanova, Conolly and Heck1999). We located one study linking DDE exposure and emotional/behavioral indicators. Sioen et al. (Reference Sioen, Den Hond, Nelen, Van de Mieroop, Croes, Van Larebeke and Schoeters2013) reported a significant positive association between prenatal DDE exposure and overall emotional/behavioral difficulties for 7- to 8-year-old girls, but not boys.
DES, an estrogenic drug, was wrongly administered to millions of pregnant women (until 1971 in the United States) in an effort to prevent spontaneous abortions and promote healthy development (Swan & vom Saal, Reference Swan, vom Saal and Metzler2001). In humans, adverse effects of DES exposure were documented for the women as well their offspring (Giusti, Iwamoto, & Hatch, Reference Giusti, Iwamoto and Hatch1995; Greenberg et al., Reference Greenberg, Barnes, Resseguie, Barrett, Burnside, Lanza and Colton1984). Most relevant with respect to temperament, adult offspring exposed to DES during most of gestation were more likely to experience symptoms of depression and anxiety, along with other psychiatric concerns, compared to those not exposed in utero (Vessey, Fairweather, Norman-Smith, & Buckley, Reference Vessey, Fairweather, Norman-Smith and Buckley1983).
PCBs are the most common among endocrine disruptors (Casati, Sendra, Sibilia, & Celotti, Reference Casati, Sendra, Sibilia and Celotti2015) because of their extensive use in dielectric and coolant fluids, no longer produced due to their toxicity and persistence. Chronic exposure to low levels of PCBs is of particular concern given evidence of cumulative endocrine, metabolic, and behavioral effects (Casati et al., Reference Casati, Sendra, Sibilia and Celotti2015; Colciago et al., Reference Colciago, Casati, Mornati, Vergoni, Santagostino, Celotti and Negri-Cesi2009; Stewart et al., Reference Stewart, Lonky, Reihman, Pagano, Gump and Darvill2008). Disruption of neurodevelopment for prenatally exposed offspring was associated with manifestations relevant to temperament: decreased novelty preference in infancy (Boucher, Bramoullé, Djebbari, & Fortin, Reference Boucher, Bramoullé, Djebbari and Fortin2014) and higher ADHD problems for school-age children (Sagiv et al., Reference Sagiv, Thurston, Bellinger, Tolbert, Altshul and Korrick2010).
Although most often examined in the context of physical development and cognitive functioning, associations with behavioral changes reflective of temperament were also noted for all of the considered contaminants.
Animal studies
The animal literature speaks to similar links between toxicant exposures and behavioral/temperament outcomes, indicating that prenatal effects can be transgenerational in nature. BPA exposure induced largely sexually dimorphic behavioral changes in young adult mice (Table 3). Chasing behavior in males was reduced to levels similar to females. Anxiety-like behaviors in an open field increased in females, decreasing in males, with elevations in aggression noted for both sexes as a result of prenatal BPA exposure (Kundakovic et al., Reference Kundakovic, Gudsnuk, Franks, Madrid, Miller, Perera and Champagne2013).
André and Markowski (Reference Andre and Markowski2006) examined learning behavior following perinatal exposure to vinclozolin, an endocrine disruptor with antiandrogenic effects. Acquisition of a reinforced response was not affected. However, males exposed to vinclozolin perinatally did not demonstrate any response extinction once food was no longer available, suggesting an alteration in sensitivity to reward/approach. In addition, Colbert et al. (Reference Colbert, Pelletier, Cote, Concannon, Jurdak, Minott and Markowski2005) demonstrated an effect of perinatal vinclozolin exposure on social behaviors. Males exposed during gestation and lactation demonstrated an increase in social play marked by more frequent initiation behavior, interpreted as a sign of hyperactivity and developmental delay.
Behavioral consequences of DES exposure were characterized in animal studies investigating developmental effects, also using this compound as a positive control. (Palanza, Parmigiani, Liu, and vom Saal (Reference Palanza, Parmigiani, Liu and vom Saal1999) reported that prenatal exposure to DDT and DES increased the frequency of same-sex aggression for male and female mice, at low doses only. There was a difference in DDT and DES effects, as males exposed to the lowest DDT dose only exhibited less intense attacks than controls.
PCB exposure in rodents has been largely studied perinatally, with animals affected during lactation as well as gestation, because this time frame corresponds to a critical brain sex differentiation period (Colciago et al., Reference Colciago, Casati, Mornati, Vergoni, Santagostino, Celotti and Negri-Cesi2009). Perinatal PCB exposure was shown to result in a dimorphic pattern of hypothalamic testosterone activating enzyme expression. A learning deficit on a passive avoidance test was also noted, as exposed males failed to develop an increased latency to enter an area associated with a previously delivered foot shock (Colciago et al., Reference Colciago, Casati, Mornati, Vergoni, Santagostino, Celotti and Negri-Cesi2009). This apparent decrease in sensitivity to cues of danger represents an aspect of fearful reactivity and reflects PCB effects on temperament development.
Prenatal endocrine disruptor exposure resulted in temperament development alterations, including increased anxiety-like and playful/aggressive behavior. There is also indication of sex differences. Learning paradigms are not directly applicable to temperament; however, sensitivity to cues of reward versus punishment can be a function of relative approach and avoidance activation. Both neurobehavioral systems appear compromised by prenatal exposure to androgen disruptors. Before we consider epigenetic mediation of environmental toxicant in utero effects, other contaminant exposures should be noted.
Lead and mercury prenatal exposure: Developmental effects
Human studies
In humans, prenatal exposure effects of lead have been demonstrated into adolescence, with more frequent/severe behavior problems, delinquent and antisocial behaviors, considering multiple covariates (Dietrich, Ris, Succop, Berger, & Bornschein, Reference Dietrich, Ris, Succop, Berger and Bornschein2001). Sioen et al. (Reference Sioen, Den Hond, Nelen, Van de Mieroop, Croes, Van Larebeke and Schoeters2013) reported a significant positive association between prenatal lead exposure and hyperactivity for 7- to 8-year-old children, controlling for other contaminants. Prenatal lead exposure effects included increased activity and deficits in focused attention at 12 months, as well as greater behavioral difficulties in the toddler period (Vermeir & Viaene, Reference Vermeir and Viaene2002–2007). Lead exposure was associated with compound effects, acting in concert with prenatal substance use. Specifically, the risk for ADHD increased for children prenatally exposed to nicotine along with lead (Froehlich et al., Reference Froehlich, Lanphear, Auinger, Hornung, Epstein, Braun and Kahn2009).
Thus, in utero lead exposure in humans has been well characterized in terms of contributing to impulsivity/approach aspects of temperament and difficulties with attention/regulation.
Animal studies
Animal studies offer more conclusive links, examining prenatal mercury as well as other contaminant exposures, noting potential sex differences and potency of low-dose exposures. Perinatal methylmercury (derived from mercury) exposure has been studied primarily in tandem with PCB, as these contaminants are typically traced to the same source (Newland & Paletz, Reference Newland and Paletz2000). Learning-related effects of prenatal exposure have been the main focus of research, with some findings relevant to temperament (Table 3). Specifically, prenatal mercury exposure in rats resulted in elevated rates of responding in an operant learning procedure, even when selenium intended to ameliorate adverse effects of methylmercury was administered to the animals. High rates of responding during reinforcement omission trials indicated prenatal mercury exposure enhanced the efficacy of reinforcement (Reed & Newland, Reference Reed and Newland2007), potentially leading to manifestations of impulsivity and/or reward dependency relevant to temperament. Male, but not female, mice demonstrated decreased spontaneous motor activity, yet increased amphetamine-induced motor activity, and altered dopamine levels. With respect to prenatal lead exposure, related sequelae were consistently more notable in animals assigned to the low-dose condition (Leasure et al., Reference Leasure, Giddabasappa, Chaney, Johnson, Pothakos, Lau and Fox2008).
The range of prenatal contaminant effects is extensive, with a number of behavioral consequences relevant to temperament. Animal studies demonstrate fearful/anxious behaviors, disruptions of social behavior, and reinforcement learning in exposed animals, with human research showing an increased risk for ADHD and behavior problems more broadly (Dietrich et al., Reference Dietrich, Ris, Succop, Berger and Bornschein2001; Kundakovic et al., Reference Kundakovic, Gudsnuk, Franks, Madrid, Miller, Perera and Champagne2013; Piedrafita, Erceg, Cauli, & Felipo, Reference Piedrafita, Erceg, Cauli and Felipo2008). This pattern of results implicates reactivity (e.g., reward/approach orientation vs. fearfulness/behavioral inhibition) and regulation (i.e., deficits in emotional/behavioral control), indicating temperament is impacted by maternal contact with toxicants during gestation. There is evidence that male offspring may be more affected (Andre & Markowski, Reference Andre and Markowski2006; Colciago et al., Reference Colciago, Casati, Mornati, Vergoni, Santagostino, Celotti and Negri-Cesi2009), and lower concentrations of contaminants, as opposed to higher levels of exposure, may be more detrimental in some instances (Leasure et al., Reference Leasure, Giddabasappa, Chaney, Johnson, Pothakos, Lau and Fox2008; Palanza, Parmigiani, & vom Saal, Reference Palanza, Parmigiani and vom Saal2001). Critical shifts in the epigenome were shown to occur as a function of prenatal environmental contaminant exposure.
Environmental contaminants: Epigenetic effects
Human studies
A recent review considered 23 studies linking environmental contaminant exposure and offspring DNA methylation changes to autism spectrum disorders (Keil & Lein, Reference Keil and Lein2016). Consistent with the environmental epigenetics framework, methylation changes were described as mediating environmental risk and conferring these effects onto behavioral phenotypes via alterations in gene expression and brain development. Shifts in the methylome and brain development that result from in utero contaminant exposures and contribute to risk for autism spectrum disorders can also be expected to affect temperament.
Animal studies
Animal studies provide additional insights into epigenetics bridging toxicant exposure in the womb and behavioral effects. Studies using BPA and vinclozolin provide evidence of transgenerational inheritance via permanent alterations in the epigenome of the germ line (Anway & Skinner, Reference Anway and Skinner2008). Skinner et al. (Reference Skinner, Anway, Savenkova, Gore and Crews2008) reported a sexually dimorphic disruption of transcription in the hippocampus and amygdala, and associated behavioral effects. Third-generation female rats from the vinclozolin-exposed line exhibited increased anxiety-like behaviors, whereas male rats displayed a decrease. Crews et al. (Reference Crews, Gore, Hsu, Dangleben, Spinetta, Schallert and Skinner2007) examined rat mate preference in a task that engaged multiple brain regions including amygdala, hippocampus, cingulated cortex, and anterior hypothalamus. Third-generation vinclozolin lineage males were less attractive to females, reflecting a differential mate preference. This study offers evidence of epigenetic transgenerational inheritance with evolutionary implications, as exposure effects translated into methylome shifts, in turn producing changes critical to reproductive success.
Luo et al. (Reference Luo, Xu, Cai, Tang, Ge, Liu and Wang2014) investigated histone modifications as the mediator of lead-related effects in rats, linking prenatal exposure to ADHD-like behaviors. A dose–response relationship was observed, as increased lead exposure resulted in greater locomotor activity, an analogue of human hyperactivity. Histone acetylation was significantly increased in the hippocampus as a result of chronic lead exposure, supporting the role of this epigenetic mechanism as a mediator between toxicant exposure and offspring symptoms. Thus, the Luo et al. (Reference Luo, Xu, Cai, Tang, Ge, Liu and Wang2014) investigation suggests prenatal lead exposure alters a temperamental phenotype associated with developmental psychopathology, namely, activity level, via an epigenetic mechanism. Together, these studies are compelling in pointing to epigenetic mechanisms as the primary vehicle of environmental exposure effects, as changes in the epigenome alter gene expression, with subsequent developmental shifts often related to temperament.
Substance Use and Effects of Psychotropic Medication
Turning to the substance use/psychotropic medication literature, again there is evidence that environmental exposures alter the epigenome, disrupting brain development and inducing temperament risk for later psychopathology. Epigenetic processes are just now being studied in this context, yet maternal substance use during pregnancy is known to markedly impact offspring development, with public health implications prompting consumption warnings.
Substance (alcohol, tobacco, marijuana, cocaine) use
Human studies
The hazards of prenatal substance use exposures are well documented. There is a wealth of human research linking in utero exposures and offspring outcomes, including temperament, with some longitudinal studies following affected children into adulthood.
Alcohol passes through the placenta, directly affecting the developing fetus and impacting placental functioning (e.g., Ahluwalia et al., Reference Ahluwalia, Wesley, Adeyiga, Smith, Da-Silva and Rajguru2000). Fetal brain development effects are considerable, evidenced by population-based estimates indicating that maternal alcohol use during pregnancy was associated with 2.84%–4.89% of intellectual disabilities cases (O'Leary et al., Reference O'Leary, Leonard, Bourke, D'Antoine, Bartu and Bower2013). The range of effects caused by prenatal alcohol exposure in totality is referred to as fetal alcohol spectrum disorders (FASD), associated with deficits in intelligence and adaptive functioning, memory, language, attention/executive functions, and motor skills (e.g., Mattson & Riley, Reference Mattson and Riley1998). Most relevant for this review, prenatal alcohol exposure was linked with temperament development (Table 4). Haley, Handmaker, and Lowe (Reference Haley, Handmaker and Lowe2006) evaluated infant HPA axis response and heart rate indictors in the context of a social stressor: a Still Face paradigm. Percentage of prenatal drinking days from conception to pregnancy recognition (extending several weeks into gastrulation) was related to increased infant cortisol reactivity, heart rate, and negative affect, controlling for maternal depression and income. In a subsequent study, maternal weekly binge drinking (≥5 drinks) during the first 6 weeks of pregnancy predicted infant difficult temperament and sleeping problems, controlling for confounds (Alvik, Torgersen, Aalen, & Lindemann, Reference Alvik, Torgersen, Aalen and Lindemann2011). Prenatally alcohol-exposed infants also exhibited increased emotional withdrawal and lower activity level, especially those later diagnosed with FASD (Molteno, Jacobson, Carter, Dodge, & Jacobson, Reference Molteno, Jacobson, Carter, Dodge and Jacobson2014).
Table 4. Examples of in utero substance/psychotropic Medication exposure effects: Temperament-related outcomes in human and animal studies
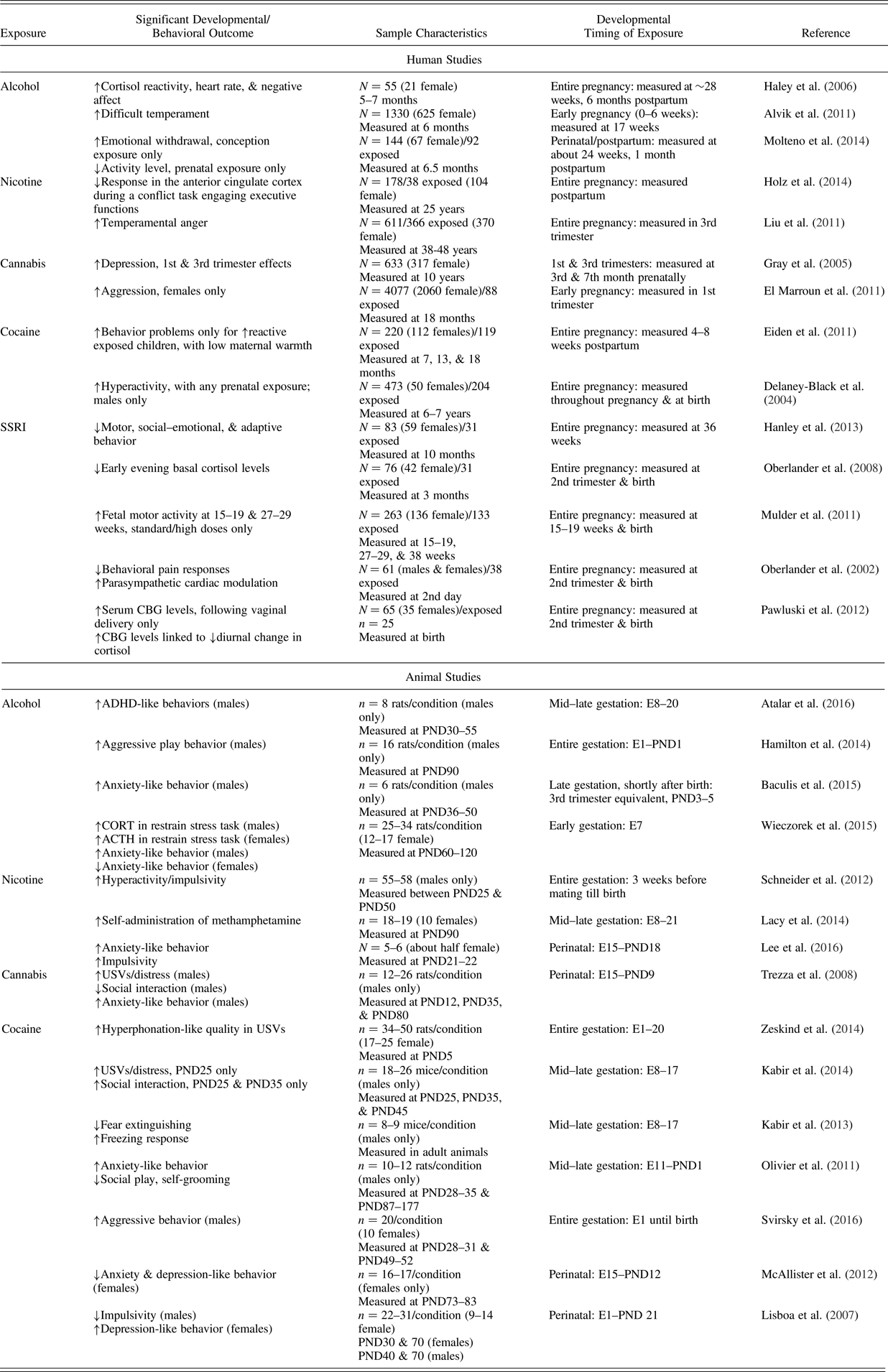
Note: Sample characteristics are provided in a different manner for human and animal studies, because of differences in design and presentation format. For human correlational studies, the overall number of participants (N) is typically presented. When indicated, the number of participants in the exposed subsample (n) is also included. The number of participants per condition (n) is always indicated for animal experimental investigations. Additional information concerning numbers of male versus female participants is provided when both sexes were included/specified. Developmental timing of exposure indicators varies across studies. For humans, the timing is provided in terms of the number of gestational weeks, although in some instances measures are just taken at birth, or not specified beyond a particular trimester or month. In animals these indicators are typically more specific, counting embryonic days or postnatal days. (↑) Increases in temperament-related behaviors/displays; (↓) decreases in temperament-related behaviors/displays; SSRI, selective serotonin reuptake inhibitor; CBG, corticosteroid-binding globulin; ADHD, attention-deficith/hyperactivity disorder; E, embryonic day; PND, postnatal day; CORT, corticosterone; ATCH, adrenocorticotropin hormone; USV, ultrasonic vocalization.
Tobacco use during pregnancy was also implicated in adverse offspring temperament outcomes. Holz et al. (Reference Holz, Boecker, Baumeister, Hohm, Zohsel, Buchmann and Laucht2014) performed functional magnetic resonance imaging, examining novelty seeking and lifetime ADHD symptoms for adults prenatally exposed to tobacco. Participants with a history of in utero exposure exhibited a weaker response in the anterior cingulate cortex to a conflict task engaging the executive attention system, coupled with novelty seeking/approach tendencies. Liu et al. (Reference Liu, Gatsonis, Baylin, Kubzansky, Loucks and Buka2011) also studied adults prenatally exposed to maternal tobacco use and demonstrated increased anger proneness.
The main psychoactive component of cannabis, Δ9-tetrahydrocannabinol, is able to cross the placenta during gestation (Hutchings, Gamagaris, Miller, & Fico, Reference Hutchings, Gamagaris, Miller and Fico1989). According to a recent review, chronic/heavy cannabis use during pregnancy appears to disrupt brain maturation, predisposing the offspring to neurodevelopmental disorders (Alpar, Di Marzo, & Harkany, Reference Alpar, Di Marzo and Harkany2016). Six- and 10-year-old children prenatally exposed to cannabis presented with more frequent/severe externalizing symptoms, impulsivity and hyperactivity in particular (Goldschmidt, Day, & Richardson, Reference Goldschmidt, Day and Richardson2000). Chronic maternal marijuana use in the first and third trimesters was associated with offspring depression at 10 years of age (Gray, Day, Leech, & Richardson, Reference Gray, Day, Leech and Richardson2005). Sex differences were noted, as prenatally exposed girls (but not boys) exhibited higher aggression and inattention at 18 months of age (El Marroun et al., Reference El Marroun, Hudziak, Tiemeier, Creemers, Steegers, Jaddoe and Huizink2011).
Child behavior problems were examined in the aftermath of prenatal cocaine exposure, with mixed results. Several studies reported significant associations between prenatal cocaine exposure and child behavior problems (Bada et al., Reference Bada, Das, Bauer, Shankaran, Lester, LaGasse and Higgins2007; Richardson, Goldschmidt, & Willford, Reference Richardson, Goldschmidt and Willford2009), yet others did not find direct links (Delaney-Black et al., Reference Delaney-Black, Covington, Nordstrom, Ager, Janisse, Hannigan and Sokol2004; Eiden, Granger, Schuetze, & Veira, Reference Eiden, Granger, Schuetze and Veira2011).
Thus, prenatal alcohol exposure clearly disrupts neurodevelopment in humans. Nicotine and marijuana programming effects have also been described. Finally, somewhat equivocal findings were reported with respect to maternal cocaine use during pregnancy.
Animal studies
Animal studies inform this literature, suggesting similar differences in the level of developmental impact for the substances examined. Prenatal alcohol effects have been widely studied via animal models, intended to serve as analogues for FASD (Table 4). Atalar, Uzbay, and Karakas (Reference Atalar, Uzbay and Karakas2016) demonstrated ADHD-like behaviors in rats following prenatal alcohol exposure throughout gestation. Exposed pups required more sessions to reach criterion relative to the control group on a reversal learning task, because of difficulty inhibiting a previously reinforced response in favor of an unreinforced/inhibited response. Moderate levels of prenatal exposure across pregnancy achieved through voluntary consumption of ethanol resulted in offspring play behavior changes (e.g., increased wrestling) reflective of aggression (Hamilton et al., Reference Hamilton, Barto, Rodriguez, Magcalas, Fink, Rice and Savage2014).
Increased anxiety-like behavior in rodents was demonstrated across multiple studies considering effects of prenatal ethanol exposure. High ethanol doses (delivered in vapor) during the rat equivalent to the human third trimester of pregnancy resulted in anxiety-like behavior (Baculis, Diaz, & Valenzuela, Reference Baculis, Diaz and Valenzuela2015). Moreover, basolateral amygdala, glutamatergic, and GABAergic synaptic transmission was implicated in the observed behavioral alteration (Baculis et al., Reference Baculis, Diaz and Valenzuela2015). Maternal alcohol treatment limited to very early stages of pregnancy (equivalent to the third week postfertilization in humans) also disrupted brain development (Godin, Poizat, Hickey, Maschat, & Humbert, Reference Godin, Poizat, Hickey, Maschat and Humbert2010) and HPA axis functioning in mice, increasing anxiety-related behaviors (Wieczorek, Fish, O'Leary-Moore, Parnell, & Sulik, Reference Wieczorek, Fish, O'Leary-Moore, Parnell and Sulik2015). Sex differences were noted: males showed elevated corticosterone following an alcohol injection, whereas females displayed a blunted adrenocorticotropic response. Anxiety-like behavior declined in exposed female mice, whereas males demonstrated heightened anxiety marked by decreased exploration (Wieczorek et al., Reference Wieczorek, Fish, O'Leary-Moore, Parnell and Sulik2015). Altering the timing of alcohol administration resulted in different brain malformations and variable patterns of behavioral disruption (Murawski, Moore, Thomas, & Riley, Reference Murawski, Moore, Thomas and Riley2015). Animal models suggest that even moderate levels of prenatal exposure lead to behavioral changes that parallel human symptomatology (Valenzuela, Morton, Diaz, & Topper, Reference Valenzuela, Morton, Diaz and Topper2012).
In utero exposure to nicotine produced deleterious behavioral effects in rats. Exposed males showed increased hyperactivity (more frequent entries) and impulsivity (increased anticipatory responses; Schneider, Bizarro, Asherson, & Stolerman, Reference Schneider, Bizarro, Asherson and Stolerman2012). Increased responding and larger doses of self-administered methamphetamine were interpreted as signs of neurobehavioral changes in the exposed offspring’ motivational systems linked with reward and vulnerability to substance use (Lacy, Morgan, & Harrod, Reference Lacy, Morgan and Harrod2014). Nicotine exposure resulted in anxiety-like and impulsive behaviors, reducing exploration and increasing instant reward choices (Lee, Chung, & Noh, Reference Lee, Chung and Noh2016).
Several studies provide evidence of increased hyperactivity and altered emotionality following prenatal cannabinoid exposure, consistent with human research. Exposed rat pups emitted more frequent ultrasonic vocalizations (USVs) indicative of distress and anxiety when removed from the nest (Trezza, Cuomo, & Vanderschuren, Reference Trezza, Cuomo and Vanderschuren2008). Adult animals also demonstrated anxiety-like behavior, spending more time in the closed arms of the maze, compared to controls (Trezza et al., Reference Trezza, Cuomo and Vanderschuren2008). Prenatal cannabinoid exposure is thought to alter offspring emotional functioning via serotonergic and dopaminergic systems (Trezza et al., Reference Trezza, Campolongo, Manduca, Morena, Palmery, Vanderschuren and Cuomo2012).
Zeskind and Stephens (Reference Zeskind and Stephens2004) examined the impact on prenatal cocaine exposure on distress vocalizations in human infants and rat pups. A shift in the acoustic structure of rodent USVs subsequent to in utero cocaine exposure paralleled hyperphonation (high-pitched cry sound) observed in humans. Prenatal cocaine exposure effects on social interactions and vocalizations were studied in mice (Kabir, Kennedy, Katzman, Lahvis, & Kosofsky, Reference Kabir, Kennedy, Katzman, Lahvis and Kosofsky2014). Exposed mice emitted more frequent USVs and demonstrated increased interactions with other animals during initial evaluations. These effects were not observed during follow-up testing and thus may not be long term (Kabir et al., Reference Kabir, Kennedy, Katzman, Lahvis and Kosofsky2014). Kabir, Katzman, and Kosofsky (Reference Kabir, Katzman and Kosofsky2013) evaluated the impact of prenatal cocaine exposure on rodent recall of extinguished cue-conditioned fear using foot shock and observed significant increases in freezing for prenatally exposed mice. According to Kabir et al. (Reference Kabir, Katzman and Kosofsky2013), prenatal cocaine exposure could increase the risk for PTSD by disrupting fear cue learning.
These animal studies compliment the human literature, indicating profound neurodevelopmental effects as a result of in utero exposure to alcohol. Less prominent disruptions were noted for prenatal cocaine administration; nonetheless, temperament alterations increasing the risk for psychopathology were observed. Prenatal cannabinoid and nicotine exposures were also implicated in long-term effects on offspring emotional functioning/temperament, potentially signaling life-long mental health risk.
Psychotropic medication/selective serotonin reuptake inhibitor (SSRI) use
Human studies
Prescribed psychotropic medications, SSRIs in particular, have also been linked with adverse offspring developmental effects when administered during pregnancy. Prenatal SSRI exposure has been linked with multiple fetal, neonatal, and infant behavioral disturbances (Table 4). For example, SSRI-exposed infants displayed decreased physiological and behavioral responses relevant to negative emotionality in the context of a typical neonatal painful event (Oberlander et al., Reference Oberlander, Eckstein Grunau, Fitzgerald, Ellwood, Misri, Rurak and Riggs2002). Altered neonatal cortisol levels were also reported (Pawluski, Brain, Underhill, Hammond, & Oberlander, Reference Pawluski, Brain, Underhill, Hammond and Oberlander2012), controlling for maternal symptoms. In light of these observations, Gur, Kim, and Epperson (Reference Gur, Kim and Epperson2013) suggested that prenatal SSRI exposure could buffer infants from increased HPA reactivity.
Temperament in SSRI-exposed and nonexposed children was compared. Nulman et al. (Reference Nulman, Rovet, Stewart, Wolpin, Gardner, Theis and Koren1997) did not identify significant differences in temperament for children of mothers using SSRI medication during pregnancy (ages 16–86 months) and those not exposed. However, temperament was only assessed directly among children younger than 24 months, with behavior problems examined for older participants. Nulman et al. (Reference Nulman, Rovet, Stewart, Wolpin, Pace-Asciak, Shuhaiber and Koren2002) again failed to identify differences with a very small sample (n = 18) of SSRI-exposed children, using similarly limited measures of temperament.
Animal studies
SSRI exposure has also been evaluated in animals, often with perinatal/neonatal administration (Table 4). Rodent studies demonstrate lifelong biobehavioral alterations following perinatal exposure, including disruption of the serotonin signaling pathway development (Maciag et al., Reference Maciag, Simpson, Coppinger, Lu, Wang, Lin and Paul2006) and increased depression-like behavior in adulthood (Ansorge, Zhou, Lira, Hen, & Gingrich, Reference Ansorge, Zhou, Lira, Hen and Gingrich2004; Olivier et al., Reference Olivier, Valles, van Heesch, Afrasiab-Middelman, Roelofs, Jonkers and Homberg2011).
Olivier at al. (Reference Olivier, Valles, van Heesch, Afrasiab-Middelman, Roelofs, Jonkers and Homberg2011) examined SSRI exposure from midgestation till birth. Prenatally exposed animals responded with increased anxiety, demonstrating greater latency to begin feeding in a novel setting and increased avoidance in a conditioned aversion test. Juvenile SSRI-treated animals engaged in fewer social play behaviors. Svirsky, Levy, and Avitsur (Reference Svirsky, Levy and Avitsur2016) also noted prenatal SSRI exposure effects on social behavior, with increased aggression for adult males, but not females. Smit-Rigter et al. (Reference Smit-Rigter, Noorlander, von Oerthel, Chameau, Smidt and van Hooft2012) provided evidence of a potential mechanism for serotonergic developmental effects, as SSRI exposure influenced dendritic complexity in the cortex, specifically serotonin receptors linked with anxiety.
McAllister, Kiryanova, and Dyck (Reference McAllister, Kiryanova and Dyck2012) reported a decrease in anxiety-like behavior, as perinatally SSRI exposed mice spent less time in the closed arms in the elevated plus maze. Depression-like behavior, reflected in longer latencies to immobility in the forced swim test, declined as a result as exposure as well. However, perinatal SSRI exposure was also associated with increased immobility (Lisboa, Oliveira, Costa, Venancio, & Moreira, Reference Lisboa, Oliveira, Costa, Venancio and Moreira2007). These discrepant results could be attributed to the SSRI delivery differences. SSRIs were administered orally in the McAllister et al. (Reference McAllister, Kiryanova and Dyck2012) study and expected to reach the offspring in relatively lower concentrations.
Thus, the totality of human and animal research addressing in utero exposure to maternal substance use indicates considerable impact on offspring emotional/behavioral functioning relevant to temperament. Emotional withdrawal and lower activity level in the context of prenatal alcohol exposure (Molteno et al., Reference Molteno, Jacobson, Carter, Dodge and Jacobson2014), as well as novelty seeking and ADHD symptoms for those prenatally exposed to tobacco (Holz et al., Reference Holz, Boecker, Baumeister, Hohm, Zohsel, Buchmann and Laucht2014), have temperament implications. Cannabinoid exposure during gestation was associated with adverse behavioral effects primarily related to the dopaminergic system (Alpar et al., Reference Alpar, Di Marzo and Harkany2016) involved in approach/positive affectivity and regulation/effortful control. Sex differences were noted, with increased aggression in SSRI-exposed males (Svirsky et al., Reference Svirsky, Levy and Avitsur2016). Exposure effects vary by substance in terms of strength and consistency. Most detrimental outcomes were reported for prenatal alcohol use, followed by nicotine and marijuana, with less consistent effects for in utero exposure to cocaine and SSRIs. Additional data concerning the timing of most pronounced prenatal effects is required, yet maternal alcohol use creates adversities for offspring health/development throughout gestation.
Prenatal substance and psychotropic medication use: Epigenetic effects
Human studies
We now consider the extent to which epigenetic processes are responsible for conferring prenatal substance use exposure risk. Consistent with the environmental epigenetics framework, prenatal maternal substance/medication use has been associated with offspring methylation changes. Portales-Casamar et al. (Reference Portales-Casamar, Lussier, Jones, MacIsaac, Edgar, Mah and Kobor2016) examined genome-wide DNA methylation patterns, identifying differentially methylated regions (DMR) in buccal cells of children with FASD and typically developing controls. Of 658 DMR, 41 displayed differences in percentage methylation change >5%. Children were between 5 and 18 years of age; thus, possible confounding postnatal variables were introduced. Laufer et al. (Reference Laufer, Kapalanga, Castellani, Diehl, Yan and Singh2015) also identified DMR associated with FASD in a comparative study, assessing methylation patterns in children (3–6 years of age) and mice prenatally exposed to alcohol. A total of 269 DMR were reported, with 21 involved in regulation of clustered protocadherin genes responsible for synaptic complexity in the developing brain (Kalmady & Venkatasubramanian, Reference Kalmady and Venkatasubramanian2009). In their review of the literature, Kobor and Weinberg (Reference Kobor and Weinberg2011) described critical developmental windows for alcohol exposure epigenetic effects: prior to conception on the paternal side and maternal alcohol consumption between fertilization and implantation.
A number of studies considered the role of prenatal tobacco exposure in altering DNA methylation in humans. Tobacco exposure was linked with a decrease in methylation of Sat2, one of the repetitive elements commonly used as a proxy for measuring genomic DNA methylation changes (Flom et al., Reference Flom, Ferris, Liao, Tehranifar, Richards, Cho and Terry2011). Genome-wide methylation differences in cord blood cells of prenatally tobacco exposed and nonexposed infants were identified (Ivorra et al., Reference Ivorra, Fraga, Bayon, Fernandez, Garcia-Vicent, Chaves and Lurbe2015). Significant differences for 31 CpG sites associated with 25 genes were observed, adjusting for multiple comparisons. Ladd-Acosta et al. (Reference Ladd-Acosta, Shu, Lee, Gidaya, Singer, Schieve and Daniele Fallin2016) also identified a methylation signature of prenatal tobacco exposure via a machine learning classification model, reliably differentiating exposed and nonexposed groups. Methylation specific to 26 sites was described as a potential biomarker of in utero tobacco exposure. Ivorra et al. (Reference Ivorra, Fraga, Bayon, Fernandez, Garcia-Vicent, Chaves and Lurbe2015) concluded that DNA methylation status at birth could serve as a marker of risk associated with prenatal environmental adversity. However, future studies are required to discern clinical significance of these epigenetic findings, as methylation changes identified in humans thus far have not been linked with behavioral alterations.
DNA methylation differences between prenatally exposed to SSRIs and nonexposed neonates were recently reported. In a genome-wide investigation, Gurnot et al. (Reference Gurnot, Martin-Subero, Mah, Weikum, Goodman, Brain and Hensch2015) identified cytochrome P450, family 2, subfamily E, polypeptide 1 (CYP2E1), the gene encoding an ethanol-metabolizing enzyme by the same name, as providing the greatest discrimination between SSRI-exposed and nonexposed neonates. The role of prenatal SSRI exposure and SLC6A4 promoter methylation status in shaping soothability, an early aspect of temperamental regulation, was examined (Gartstein, Hookenson, et al., Reference Gartstein, Hookenson, Brain, Devlin, Grunau and Oberlander2016). For SSRI-exposed infants only, more advanced development of soothability was observed with higher SLC6A4 methylation levels.
Animal studies
Animal studies inform regarding epigenetic mechanisms as bridges between in utero substance use/exposure and offspring development, especially with respect to epigenetic shifts impacting gene expression in the brain. Rodent models developed to understand the etiology of FASD in humans enable links between analogue phenotypes to epigenetic mechanisms. Immediate and long-lasting alterations of DNA methylation and microRNAs, crucial for synaptic plasticity and behavioral/emotional functioning, have been reported. Marjonen et al. (Reference Marjonen, Sierra, Nyman, Rogojin, Gröhn, Linden and Kaminen-Ahol2015) noted shifts in offspring DNA methylation, gene expression, and brain structure following chronic moderate ethanol exposure in early gestation, corresponding to the first 3–4 weeks of human pregnancy. Gene expression analyses conducted with mouse hippocampal tissue of ethanol-exposed adolescent offspring revealed altered expression of 23 genes and three microRNAs. However, no connections were made between gene expression changes and brain structure/function changes.
Methylation of the insulin-like growth factor II (IGF-II), an imprinted gene with a role in memory consolidation and enhancement, was examined in the context of prenatal cocaine exposure with mice (Zhao et al., Reference Zhao, Hou, Chen, Shao, Zhu, Bu and Cen2015). Offspring of mothers administered cocaine during pregnancy demonstrated increased anxiety, along with impaired memory. Hippocampal IGF-II mRNA and protein expressions were significantly decreased, likely contributing to the phenotypic changes (Zhao et al., Reference Zhao, Hou, Chen, Shao, Zhu, Bu and Cen2015). Prenatal administration of cocaine to mice was also shown to alter genome-wide DNA methylation in the offspring hippocampal tissue (Novikova et al., Reference Novikova, He, Bai, Cutrufello, Lidow and Undieh2008). Exposed and nonexposed mice were differentiated by methylation of 492 CpG islands, a number associated with repetitive elements (e.g., LINE, Alu; Novikova et al., Reference Novikova, He, Bai, Cutrufello, Lidow and Undieh2008).
Epigenetic alterations associated with prenatal cannabis exposure have also been considered, albeit not as extensively. DiNieri et al. (Reference DiNieri, Wang, Szutorisz, Spano, Kaur, Casaccia and Hurd2011) examined fetal brain tissue and reported decreased dopamine receptor D2 (DRD2) mRNA expression in the human ventral striatum (nucleus accumbens). DRD2 mRNA levels were negatively associated with maternal report of prenatal cannabis use. Morris, DiNieri, Szutorisz, and Hurd (Reference Morris, DiNieri, Szutorisz and Hurd2011) reviewed contributions of cannabis and tobacco exposures, as these in utero effects commonly co-occur in humans. Consistent with the environmental epigenetics perspective, a critical role of epigenetic mechanisms, methylation in particular, was noted in shaping offspring neurodevelopment.
Thus, existing research provides evidence of gene expression changes, modulated via methylation and noncoding RNA mechanisms sensitive to prenatal substance use. The extent of epigenetic alterations may be related to the level of developmental disruption, with alcohol exposure expected to trigger greater gene expression changes. Multiple downstream behavioral effects relevant to temperament have been noted. However, in humans, connections between prenatal substance use exposure, epigenetic changes, and alterations in temperament development have not been made in a convincing manner.
Nutrition: Undernutrition, overnutrition, and micronutrients
Human studies
These links were somewhat better established in the context of studies addressing prenatal nutritional exposures, in part because of an opportunity for a natural experiment created by a World War II humanitarian disaster. Effects of gestational undernutrition in humans have been studied most extensively following individuals who were prenatally exposed to famine during the Dutch Hunger Winter (1944–1945): a period of severe food shortage in the western region of the Netherlands during the last winter of World War II (Barua & Junaid, Reference Barua and Junaid2015). The Dutch Famine Birth Cohort Study is a population-based investigation with volunteers prenatally affected by undernutrition (Roseboom et al., Reference Roseboom, de Rooij and Painter2006). In utero undernutrition was predictive of psychopathology, insofar as the role of exposure in affective disorders was documented based on a large-scale archival study (Table 5). Undernutrition as a result of famine in middle or late gestation resulted in a higher risk for hospitalization for unipolar and bipolar depression, with a stronger effect observed for men (Brown, van Os, Driessens, Hoek, & Susser, Reference Brown, van Os, Driessens, Hoek and Susser2000). Famine and undernutrition exposure early in gestation increased the risk for anxiety in adulthood (de Rooij et al., Reference de Rooij, Painter, Phillips, Raikkonen, Schene and Roseboom2011). Males exposed to famine during early gestation were again affected to a greater extent, self-reporting more frequent/severe symptoms of anxiety and depression. This pattern of results is consistent with the evidence indicating males may be more vulnerable to nutritional deprivation during certain periods of fetal development because of faster growth relative to females (Eriksson, Kajantie, Osmond, Thornburg, & Barker, Reference Eriksson, Kajantie, Osmond, Thornburg and Barker2010). Other cohorts exposed to undernutrition and nutritional interventions have been evaluated; however, behavioral/emotional outcomes were not addressed. Interest in fetal programming related to overnutrition has grown as a result of the “obesity epidemic.” Connections between excessive prenatal nutrition and behavioral/temperament outcomes are starting to emerge.
Table 5. Examples of in utero nutrition exposure effects: Temperament-related outcomes in human and animal studies

Note: Sample characteristics are provided in a different manner for human and animal studies, because of differences in design and presentation format. For human correlational studies, the overall number of participants (N) is typically presented, whereas the number of participants per condition (n) is indicated for animal experimental investigations. Additional information concerning numbers of male versus female participants is also provided when both sexes were included/specified. Developmental timing of exposure indicators varies across studies. For humans, the timing is provided in terms of the number of gestational weeks. In animals these indicators often refer to whether the nutritional exposure was periconceptional, beginning around conception and including the postnatal/lactation period, or starting at pregnancy recognition, with a number of studies providing specific weeks/days. (↑) Increases in temperament-related behaviors/displays; (↓) decreases in temperament-related behaviors/displays; E, embryonic day; PND, postnatal day; USV), ultrasonic vocalization; MK-801, N-methyl-d-aspartate receptor (NMDA-R) antagonist.
Prenatal nutrition characterized as “unhealthy” (high in fat) was predictive of multiple emotional/behavioral problems later in childhood (Jacka et al., Reference Jacka, Ystrom, Brantsaeter, Karevold, Roth, Haugen and Berk2013). Increased maternal intake of unhealthy foods (e.g., processed meat products) during pregnancy predicted child externalizing problems at 5 years of age, controlling for covariates including childhood diet. Most relevant to this review, Gustafsson, Kuzava, Werner, and Monk (Reference Gustafsson, Kuzava, Werner and Monk2016) reported associations between maternal prenatal dietary fat intake and offspring temperament. Specifically, maternal total fat intake was linked with infant surgency and regulatory capacity at 4 months of age, so that higher fat content translated into lower positive affectivity and regulation indicative of temperament risk. Together, human studies indicate temperament alterations and an increased risk for behavior problems/symptoms as a consequence of prenatal exposure to under- and overnutrition.
Prenatal effects of multiple nutrients have been reported for child neurodevelopment (e.g., B vitamins, zinc, protein, iodine, etc.; Georgieff, Reference Georgieff2007) and can be expected to influence temperament as a result. For example, Hernández-Martínez et al. (Reference Hernández-Martínez, Canals, Aranda, Ribot, Escribano and Arija2011) reported that first- and second-trimester iron deficiency predicted neonatal autonomous nervous system functioning, including signs of stress reactivity. In a recent review, poor nutrition during pregnancy was described as contributing to deficits in neurocognitive development (e.g., sensory–perceptual skills, memory, an learning), especially in the context of maternal distress (Monk, Georgieff, & Osterholm, Reference Monk, Georgieff and Osterholm2013).
Animal studies
Connections between unhealthy in utero nutrition and subsequent adverse behavioral/temperament outcomes for the exposed offspring are further supported by animal research. A variety of animal models have been utilized to examine fetal programming associated with nutritional factors (Table 5), as these allow precisely timed diet manipulations inducing nutritional accesses and deficits. Levay et al. (Reference Levay, Paolini, Govic, Hazi, Penman and Kent2008) examined effects of moderate maternal calorie restriction in preconception and the perinatal period on adult offspring anxiety-like behaviors. Preconception deprivation (50% caloric intake for 3 days) exerted the strongest effect with respect to most offspring anxiety-like behavior (e.g., less exploration in the open field). Nonhuman primates exposed to maternal undernutrition during gestation demonstrated greater variability in activity level and less emotional arousal (Keenan et al., Reference Keenan, Bartlett, Nijland, Rodriguez, Nathanielsz and Zurcher2013). Females, but not males, showed lower levels of attention/persistence, ascertained based on computerized task engagement and performance. Keenan et al. (Reference Keenan, Bartlett, Nijland, Rodriguez, Nathanielsz and Zurcher2013) concluded that prenatal diet interventions could be effective in preventing common behavior problems in children.
Preconceptional/perinatal maternal high-fat diet effects on anxiety-related behaviors, as well as expression of GABAergic, serotonergic, and brain-derived neurotrophic factor (BDNF) receptors involved in anxiety and depression, were examined in mice. Offspring in the high-fat diet condition exhibited increased anxiety-like behaviors, with expression alterations noted in the hippocampus (i.e., increased BDNF and GABA receptors; Peleg-Raibstein, Luca, & Wolfrum, Reference Peleg-Raibstein, Luca and Wolfrum2012). Adult offspring exposed to a perinatal high-fat diet showed increased expression of corticosterone receptors in the amygdala and inflammatory gene expression in the hippocampus and amygdala. These changes were linked with increased anxiety behavior (e.g., less time in the open field; Sasaki, de Vega, St.-Cyr, Pan, & McGowan, Reference Sasaki, de Vega, St.-Cyr, Pan and McGowan2013). Males subjected to a high-fat diet through gestation and lactation demonstrated increased corticosterone serum levels. Anxiety-related effects were mixed, with offspring from the pregestation dietary intervention group (mothers returned to a healthy diet) showing partial recovery (Rodriguez et al., Reference Rodriguez, Rodriguez-Gonzalez, Reyes-Castro, Ibanez, Ramirez, Chavira and Zambrano2012). Substance-use related behavioral effects of prenatal high-fat exposure have been documented in rats (Karatayev et al., Reference Karatayev, Lukatskaya, Moon, Guo, Chen, Algava and Leibowitz2015). Exposed offspring engaged in more extensive nicotine and ethanol self-administration, relevant to temperament because of associated impulsivity/behavioral undercontrol. This connection between prenatal high-fat diet exposure and offspring deficits in regulation was further supported by a recent review. Sullivan, Nousen, and Chamlou (Reference Sullivan, Nousen and Chamlou2014) noted that primary offspring outcomes of perinatal high-fat diet programming involved behavioral/emotional dysregulation, further suggesting serotonergic system suppression as the likely mechanism.
The impact of a calorie-dense perinatal diet (high in saturated fat, refined sugar, and cholesterol) on the development of piglets was examined (Clouard, Gerrits, Kemp, Val-Laillet, & Bolhuis, Reference Clouard, Gerrits, Kemp, Val-Laillet and Bolhuis2016). Prenatal exposure resulted in decreased basal salivary cortisol levels, relative to controls. Animals exposed to the high-fat/sugar/cholesterol diet spent more time walking in their pens, with control group animals inactive longer.
Micronutrient deficiencies have been studied extensively in animals. Iron deficiency during gestation was examined in nonhuman primates, with exposed offspring demonstrating reduced activity. Ratings of “fearful” affect emerged as the most critical temperament descriptor differentiating prenatally iron-deprived animals from controls (Golub et al., Reference Golub, Kaufman, Campbell, Li and Donald2006). In rodents, females exposed to zinc deprivation throughout gestation exhibited higher levels of aggression, relative to females born to typically fed mothers and those exposed to overall undernutrition (Halas, Reynolds, & Sandstead, Reference Halas, Reynolds and Sandstead1977). Adult offspring perinatally exposed to vitamin D deficiency engaged in more frequent premature responses on a learning task, relative to control animals, interpreted as impulsivity induced by the deprivation (Turner et al., Reference Turner, Dalton, Inaoui, Fogelman, Fraser and Hampson2013). Hyperlocomotion in rats following vitamin D deficiency was also reported. Full and late term, but not early gestational, deprivation resulted in this behavioral phenotype (O'Loan et al., Reference O'Loan, Eyles, Kesby, Ko, McGrath and Burne2007). Late gestation was described as the likely “critical window” for vitamin D deprivation, corresponding to midgestation in humans (Clancy, Darlington, & Finlay, Reference Clancy, Darlington and Finlay2001). Pan et al. (Reference Pan, Jin, Chatterjee-Chakraborty, Halievski, Lawson, Remedios and Fleming2014) considered effects of both deficient and excessive perinatal levels of vitamin D, following exposed offspring into adulthood. Exposure to vitamin D deficiency resulted in lower offspring activity and increased anxiety (e.g., fewer open arm entries). Vitamin D excess was also linked with adverse effects, and Pan et al. (Reference Pan, Jin, Chatterjee-Chakraborty, Halievski, Lawson, Remedios and Fleming2014) suggested high concentrations may be toxic.
Folate deficiency during gestation was associated with more frequent anxiety-related behavior in the elevated plus maze (Ferguson et al., Reference Ferguson, Berry, Hansen, Wall, White and Antony2005). At the same time, offspring exposed to high levels of folic acid exhibited increased USVs, anxiety, and hyperactivity-like behaviors (Barua et al., Reference Barua, Chadman, Kuizon, Buenaventura, Stapley, Ruocco and Junaid2014). This pattern of result prompted Barua et al. (Reference Barua, Chadman, Kuizon, Buenaventura, Stapley, Ruocco and Junaid2014) to recommend moderation in folic acid supplementation.
Overall, behavioral effects as a result of prenatal under- and overnutrition have been observed across human and animal studies, with some indication of sex differences (de Rooij et al., Reference de Rooij, Painter, Phillips, Raikkonen, Schene and Roseboom2011; Keenan et al., Reference Keenan, Bartlett, Nijland, Rodriguez, Nathanielsz and Zurcher2013). Both sets of investigations implicated symptoms of anxiety, relevant to temperament as fear/behavioral inhibition, represents a prominent risk factor. Moreover, animal studies provide evidence of brain development alterations (e.g., expression of receptors involved in anxiolytic medication effects). Behavioral/emotional dysregulation emerged as another consequence of prenatal nutritional adversity, with temperament-related outcomes implicated in studies addressing prenatal deficiencies/accesses of micronutrients. Impulsivity and activity-level increases were noted, with some indication of a critical window with respect to vitamin D effects. These effects are not surprising, as brain development is altered with under- and overnutrition, in part due to sensitivity to deficits or overabundance of specific nutrients (Georgieff, Reference Georgieff2007). Cunha et al. (Reference Cunha Fda, Dalle Molle, Portella, Benetti Cda, Noschang, Goldani and Silveira2015) argued for the “similarities in the inequalities” model, as offspring prenatally exposed to both undernutrition and high-fat conditions demonstrated similar effects (e.g., lower birth weight and decreased activity level), compared to controls. In a recent review, Langley-Evans (Reference Langley-Evans2015) suggested: “a limited number of common mechanisms may link nutritional ‘stressors’ to development changes that result in later disease.” Prenatal nutritional effects are both unique and co-acting with respect to maternal stress and mood, leading to alterations in the offspring dopaminergic and serotonergic systems (Sullivan, Smith, & Grove, Reference Sullivan, Smith and Grove2011). Psychosocial stress is a known contributor to unhealthy eating during pregnancy (Hurley, Caulfield, Sacco, Costigan, & Dipietro, Reference Hurley, Caulfield, Sacco, Costigan and Dipietro2005) and can alter the manner in which nutrients are metabolized (e.g., accelerating protein breakdown), with further negative consequences for the fetus (Monk et al., Reference Monk, Georgieff and Osterholm2013). As noted earlier, co-occurrence with additional exposures (e.g., substance use) should be addressed in the future. Connecting these overlapping environment exposures to their epigenetic signatures will be important going forward.
Prenatal Nutritional Exposure in Humans and Animals: Epigenetic Mechanisms
The role of epigenetic processes in conferring effects of prenatal nutrition are already being addressed and will be discussed next. Prenatal undernutrition exposure research in humans provides further support for the environmental epigenetics framework. Results indicate a variety of epigenetic changes, in some cases linked with disease-related phenotypes, but not behavior/temperament (Heijmans et al., Reference Heijmans, Tobi, Stein, Putter, Blauw, Susser and Lumey2008; Tobi et al., Reference Tobi, Goeman, Monajemi, Gu, Putter, Zhang and Heijmans2014). Periconceptional exposure to famine during the Dutch Hunger Winter was associated with a lifelong methylation signature linked with physiological phenotypes. For example, methylation of DMR associated with the physical growth and insulin signaling gene (insulin receptor [INSR]) was positively correlated with birth weight. Fatty acid oxidation gene (carnitine palmitoyltransferase 1A [CPT1A]) methylation was positively correlated with low-density lipoprotein cholesterol levels, after adjusting for multiple covariates (e.g., age and smoking; Tobi et al., Reference Tobi, Goeman, Monajemi, Gu, Putter, Zhang and Heijmans2014). One study with humans utilized a genome-wide approach, demonstrating that micronutrient supplementation was associated with decreased methylation, interpreted as important to “programming gene activity” later in life (Khulan et al., Reference Khulan, Cooper, Skinner, Bauer, Owens, Prentice and Affara2012). Unfortunately, behavioral outcomes relevant to temperament were not examined.
In a mouse model, perinatal exposure to a high-fat diet was linked with DNA hypomethylation along with alterations in dopamine and opioid-related gene expression (Vucetic, Kimmel, Totoki, Hollenbeck, & Reyes, Reference Vucetic, Kimmel, Totoki, Hollenbeck and Reyes2010). Micronutrient deficits can also influence gene expression, as perinatal iron deficiency was associated with altered mRNA expression in the brain (Clardy et al., Reference Clardy, Wang, Zhao, Liu, Chase, Beard and Connor2006). Konycheva et al. (Reference Konycheva, Dziadek, Ferguson, Krägeloh, Coolen, Davison and Breier2011) examined prenatal methyl donor deficiency effects on offspring hippocampal methylation of candidate genes involved in glucocorticoid metabolism and anxiety-related behaviors. Exposed animals demonstrated increased anxiety. However, methylation of candidate genes was not associated with any of these behavioral changes, suggesting the need for a genome-wide strategy. Nonhuman primates prenatally exposed to a chronic maternal high-fat diet evidenced epigenetically driven serotonin signaling pathway alterations linked with fearful/anxious behavior (Sullivan et al., Reference Sullivan, Grayson, Takahashi, Robertson, Maier, Bethea and Grove2010). Sex differences were noted in responses to threatening novel objects, as female high-fat exposed offspring exhibited increased anxiety, and males demonstrated higher levels of aggression. Overall 78% of animals born to mothers subjected to a chronic high-fat diet exhibited disordered behavior. Consistent with the environmental epigenetics perspective, deficits and accesses in prenatal nutrition were shown to result in epigenetic shifts for the offspring, yet only a limited number of studies demonstrated links between these alternations and behavioral outcomes.
In summary, the totality of existing studies suggests important links between environmental exposures, epigenetic alterations, and shifts in behavioral outcomes relevant to temperament (e.g., fear/anxiety). However, connections among these levels of analysis have not been made frequently, especially in humans. In addition, questions remain regarding optimal developmental widows and sex differences in programming effects. In terms of stress, human candidate gene studies suggest methylation of NR3C1 and SLC6A4 are critical to conferring prenatal effects for several temperament outcomes (e.g., infant HPA axis reactivity; Montirosso et al., Reference Montirosso, Provenzi, Fumagalli, Sirgiovanni, Giorda, Pozzoli and Borgatti2016; Oberlander et al., Reference Oberlander, Grunau, Mayes, Riggs, Rurak, Papsdorf and Weinberg2008; Ostlund et al., Reference Ostlund, Conradt, Crowell, Tyrka, Marsit and Lester2016). However, enthusiasm for these as potential markers of exposure effects is dampened by the number of other genes implicated in stress-related methylation shifts, as evidenced by a genome-wide approach (Nieratschker et al., Reference Nieratschker, Massart, Gilles, Luoni, Suderman, Krumm and Szyf2014). Toxicant studies provide consistent evidence of environmental exposures translating into epigenetic shifts. These alterations of the epigenome affect developmental cascades relevant to temperament phenotypes (Keil & Lein, Reference Keil and Lein2016; Skinner et al., Reference Skinner, Anway, Savenkova, Gore and Crews2008). Among notable substance-use related findings, Zhao et al. (Reference Zhao, Hou, Chen, Shao, Zhu, Bu and Cen2015) reported transcription alterations in the hippocampus and increased anxiety-like behavior following prenatal cocaine exposure. Existing prenatal nutrition research indicates connections between methylation status and temperament, as expected based on the environmental epigenetic framework. Nutrition affects maternal health/physiology, a key determinant of the intrauterine milieu, shaping the progression from environmental exposure to changes in offspring epigenome, brain, and temperament development.
DNA Methylation and Temperament: Implications for Behavioral/Emotional Health
The course of prenatal development is altered by exposure to maternal stress, contact with toxicants, substance/psychotropic medication use, and nutrition. These environmental factors impact maternal physiology during gestation and program the offspring. As anticipated by the environmental epigenetics perspective, prenatal exposures appear to confer subsequent behavioral phenotypes via epigenetic mechanisms. Brain development is sensitive to multiple exposures during gestation, and this epigenetically driven fetal programming shapes regions/systems relevant to temperament. Even subtle alterations in brain structure/function during fetal development can become magnified over time, producing lifelong deficits (Buss et al., Reference Buss, Davis, Shahbaba, Pruessner, Head and Sandman2012). Development of the amygdala and hippocampus, relevant to fear/avoidance and other temperament-related behaviors, was disrupted by prenatal stress (Barros, Myers, Van Driesche, & Tzagoloff, Reference Barros, Myers, Van Driesche and Tzagoloff2006), environmental contaminants (Skinner et al., Reference Skinner, Anway, Savenkova, Gore and Crews2008), maternal alcohol use (Baculis et al., Reference Baculis, Diaz and Valenzuela2015), and a high-fat diet (Sasaki et al., Reference Sasaki, de Vega, St.-Cyr, Pan and McGowan2013). Prenatal stress effects on the synthesis and transport of dopamine, as well as relevant receptors, were also noted (Antonelli et al., Reference Antonelli, Pallares, Ceccatelli and Spulber2016). Development of the dopaminergic system can be disrupted by prenatal lead and cannabinoid exposure, as well as maternal nutritional state (DiNieri et al., Reference DiNieri, Wang, Szutorisz, Spano, Kaur, Casaccia and Hurd2011; Leasure et al., Reference Leasure, Giddabasappa, Chaney, Johnson, Pothakos, Lau and Fox2008; Sullivan et al., Reference Sullivan, Nousen and Chamlou2014). The dopaminergic system plays multiple roles relevant to temperament as it functions to prioritize stimuli or responses, facilitating selection of alternatives in either a perceptual-cognitive or a motor-behavioral context (Trofimova & Robbins, Reference Trofimova and Robbins2016). The serotonin signaling pathway also pertains to temperament given links to depression and anxiety associated with negative emotionality. Serotonergic effects were most pronounced in studies examining in utero SSRI exposure (Smit-Rigter et al., Reference Smit-Rigter, Noorlander, von Oerthel, Chameau, Smidt and van Hooft2012). Changes in serotonin signaling were also observed in the context of prenatal restraint stress (Miyagawa, Tsuji, Fujimori, Saito, & Takeda, Reference Miyagawa, Tsuji, Fujimori, Saito and Takeda2011). Compound effects of maternal diet, stress, and mood, as well as sleep and exercise, can be expected to shape offspring serotonergic and dopaminergic neurotransmitter systems (Sullivan et al., Reference Sullivan, Smith and Grove2011), and thus should be explored in future research. Overall, epigenetically mediated prenatal exposure effects on brain development have considerable implications for temperament, especially stress reactivity, fear/anxiety, impulsivity, and attention/regulation.
Epigenetic mechanisms have been linked with behavioral phenotypes relevant to temperament by multiple investigations. In one such study, genome-wide DNA methylation and mRNA expression analyses were conducted with primate amygdala tissue collected from juveniles exhibiting a fearful temperament (freezing, increased levels of cortisol; Kalin & Shelton, Reference Kalin and Shelton2003). Transcriptome analyses distinguished 22 genes with active expression changes, of which two glutamate receptor genes (glutamate ionotropic receptor NMDA type subunit 1 [GRIN1] and metabotropic glutamate receptor 5 [GRM5]) play a role in fear and anxiety-like behaviors. NR3C1 and SLC6A4 methylation has been linked with infant duration of orienting, approach, soothability, and fear, measured via parent report (Gartstein, Hookenson, et al., Reference Gartstein, Hookenson, Brain, Devlin, Grunau and Oberlander2016; Montirosso et al., Reference Montirosso, Provenzi, Fumagalli, Sirgiovanni, Giorda, Pozzoli and Borgatti2016; Ostlund et al., Reference Ostlund, Conradt, Crowell, Tyrka, Marsit and Lester2016). Methylation of imprinted genes was also associated with infant temperament: higher methylation of maternally expressed gene intergenic (MEG3-IG) linked with greater surgency and of the progression elevated gene 3 (PEG3) with higher levels of negative emotionality and externalizing problems (Fuemmeler et al., Reference Fuemmeler, Lee, Soubry, Iversen, Huang, Murtha and Hoyo2016). In adulthood, DNA methylation of the oxytocin structural gene (OXT) was associated with sociability and greater superior temporal sulcus activity during social–cognitive functional MRI tasks (Haas et al., Reference Haas, Filkowski, Cochran, Denison, Ishak, Nishitani and Smith2016). Existing evidence points to the importance of epigenetic processes as mediators of prenatal effects, conferring environmental risk onto temperament-related phenotypes. As indicated by the environmental epigenetics perspective, a variety of environmental adversities impact maternal health/physiology, resulting in a cascade of epigenetic changes that shape central nervous system maturation and temperament, in turn, often translating into similar phenotypes across exposures.
Prenatal exposure to multiple adversities results in temperament profiles marked by high levels of fear/behavioral inhibition and risk for anxiety, with these effects mediated by epigenetic processes. On the animal side, exposure to prenatal stress resulted in a number of offspring “fearful” behaviors, such as higher levels of inhibition in response to foot shock, decreased time spent in the center of an open field and in open arms of the elevated-plus maze, increased defensive withdrawal, and conditioned fear (Dickerson, Lally, Gunnel, Birkle, & Salm, Reference Dickerson, Lally, Gunnel, Birkle and Salm2005; Griffin & Evans, Reference Griffin and Evans2003; Ward, Johnson, Salm, & Birkle, Reference Ward, Johnson, Salm and Birkle2000). Epigenetic mechanisms were implicated in the risk for increased fear/anxiety observed in the context of prenatal toxicant exposure (e.g., Skinner et al., Reference Skinner, Anway, Savenkova, Gore and Crews2008) and for humans prenatally exposed to undernutrition (de Rooij et al., Reference de Rooij, Painter, Phillips, Raikkonen, Schene and Roseboom2011).
With respect to depression, temperament risk primarily involves increased negative emotionality, along with decreased approach/positive affectivity (Lonigan, Carey, & Finch, Reference Lonigan, Carey and Finch1994; Lonigan et al., Reference Lonigan, Phillips and Hooe2003). This profile resulted from several reviewed exposures shown to operate via epigenetic processes. Alvik et al. (Reference Alvik, Torgersen, Aalen and Lindemann2011) demonstrated that maternal binge drinking in early pregnancy predicted offspring temperament marked by high negative affect, low regulatory capacity, and positive affectivity in infancy, which could bode future depressive symptoms. Prenatal high-fat diet was associated with lower infant surgency (Gustafsson et al., Reference Gustafsson, Kuzava, Werner and Monk2016), which could translate into long-term risk for depression given high negative emotionality. Prenatal stress and maternal high-fat diet were shown to disrupt serotonin neuronal development (Miyagawa et al., Reference Miyagawa, Tsuji, Fujimori, Saito and Takeda2011; Peleg-Raibstein et al., Reference Peleg-Raibstein, Luca and Wolfrum2012), likely contributing to temperament risk for depression. In utero exposure to SSRIs was associated with offspring serotonergic effects (Oberlander, Reference Oberlander2012), although additional research is required to determine their long-term significance.
Tentative conclusions can be drawn regarding disruptive behaviors/disorders. General maternal anxiety during pregnancy was associated with increased infant surgency, negative affectivity, and poorer self-regulation (van den Heuvel et al., Reference van den Heuvel, Johannes, Henrichs and Van den Bergh2015), linked with risk for conduct disturbance. Offspring behavioral/emotional dysregulation reported following prenatal trauma-related exposures (i.e., trauma occurrence or maternal PTSD; Yong Ping et al., Reference Yong Ping, Laplante, Elgbeili, Hillerer, Brunet, O'Hara and King2015) is also consistent with temperament risk for disruptive behaviors. Studies that measure offspring ADHD-like behaviors (Atalar et al., Reference Atalar, Uzbay and Karakas2016; Holz et al., Reference Holz, Boecker, Baumeister, Hohm, Zohsel, Buchmann and Laucht2014; Luo et al., Reference Luo, Xu, Cai, Tang, Ge, Liu and Wang2014; Sagiv et al., Reference Sagiv, Thurston, Bellinger, Tolbert, Altshul and Korrick2010) suggest multiple prenatal toxicant and maternal substance use effects. Luo et al. (Reference Luo, Xu, Cai, Tang, Ge, Liu and Wang2014) demonstrated histone modifications mediated prenatal lead exposure effects on ADHD-like behavior in juvenile rats, serving as a bridge between environmental toxicant exposure and offspring symptomatology.
Biologically speaking, phenotypes are considered beneficial to the extent that these increase survival and subsequent reproductive success. These need not be socially desirable or health promoting in the long term (Frankenhuis, Panchanathan, & Belsky, Reference Frankenhuis, Panchanathan and Belsky2016). Children who experience adverse fetal programming effects likely present with temperament profiles aimed at increasing their immediate probability of survival (e.g., greater reactivity/fearfulness), which may not align with caregivers’ and societal demands/expectations. These temperament trajectories speak to long-term risk, increasing the likelihood of developing psychopathology/behavior problems that typically emerge after the first year of life. Alternatively, these phenotypes may be reflective of increased sensitivity to context, with superior outcomes under optimal environmental conditions. Selection pressures may favor differential plasticity when environmental conditions vary across time, with epigenetic mechanisms explaining “bet hedging” that leads to varying offspring plasticity (Frankenhuis et al., Reference Frankenhuis, Panchanathan and Belsky2016). As DOHaD research has already demonstrated, environmental epigenetic modifications immediately protective in adverse circumstances (e.g., inadequate prenatal nutrition and stress) result in a spectrum of risk-promoting developmental shifts relevant to temperament and mental health in the long term (Hochberg et al., Reference Hochberg, Feil, Constancia, Fraga, Junien, Carel and Albertsson-Wikland2011).
Conclusion
Considerable evidence points to the importance of in utero environmental exposures in shaping offspring temperament development, with epigenetic mechanisms conferring the risk, as indicated by the environmental epigenetics perspective. Greater understanding of these connections is critical for advancing DOHaD research and specifically for elucidating prenatal origins of developmental psychopathology, which could lead to more effective preventative efforts. Additional study is required to make these connections for eventual use of epigenetic information in targeted interventions.
First, research that starts with environmental adversities and considers epigenetic alterations as well as brain/behavior effects related to temperament has been limited to date. Investigations that link all components of the developmental progression and levels of analysis are needed, especially with humans. Animal studies provide an opportunity to directly manipulate independent variables related to environmental exposure, ensuring internal validity, yet their external validity with respect to human applications is necessarily limited. Thus, efforts with humans are required, translating animal findings in a manner that provides support for generalizability. Future research could focus on links between epigenetic mechanisms and temperament development in high- and low-risk populations. Despite a quasi-experimental design, multiple fetal programming pathways described in this review could be examined. For example, recruiting samples of pregnant women from the same community that differ in terms of psychosocial and/or physiological stress could provide comparisons with respect to effects of the HPA axis activation. It would be possible to examine other environmental exposures, such as healthy versus unhealthy prenatal nutrition, in a similar fashion. This approach could elucidate reliable connections between environmental adversity, maternal health, epigenetic mechanisms, and offspring behavioral outcomes with human participants, given appropriate statistical control of covariates (e.g., postpartum variables).
Second, some conclusions can be made about developmental windows associated with stronger effects; however, additional research is required to clarify these across species for different environmental exposures. Overall, earlier stages of prenatal development afford greater malleability of organ structure and function and are more “open” to environmental influences via epigenetic modifications (Crews, Reference Crews2010). At the same time, a number of reviewed exposure effects occurred later in gestation (e.g., Yehuda et al., Reference Yehuda, Engel, Brand, Seckl, Marcus and Berkowitz2005), presumably because this timing coincides with the maturation of brain regions relevant to temperament. Comparative studies including animal and human participants with parallel exposure windows are likely to be especially informative, given divergent sensitive period findings thus far.
Third, interactions among different in utero exposure conditions should be examined more closely in future research, as compound effects are likely relevant to temperament. Epigenetic modifications invoked by different prenatal environmental circumstances may have substantial overlap, leading to diverse developmental and health risks. Comparative studies are likely to inform this effort. Multiple exposures can be manipulated with respect to dose, timing, and duration with animals, in a manner that parallels human prenatal effects. For example, comparative research could address joint effects of prenatal stress and inadequate nutrition, as well as maternal polysubstance use.
Fourth, the pattern emerging from existing studies suggests that males may be more vulnerable to physiological and behavioral effects of prenatal environmental exposures (Andre & Markowski, Reference Andre and Markowski2006; Colciago et al., Reference Colciago, Casati, Mornati, Vergoni, Santagostino, Celotti and Negri-Cesi2009; Eriksson et al., Reference Eriksson, Kajantie, Osmond, Thornburg and Barker2010; Leasure et al., Reference Leasure, Giddabasappa, Chaney, Johnson, Pothakos, Lau and Fox2008). Sex differences are generally predicted in the direction of females exhibiting greater anxiety/depression and males presenting with higher aggression/impulsivity, with a number of studies providing support (Nazeri et al., Reference Nazeri, Shabani, Ghotbi Ravandi, Aghaei, Nozari and Mazhari2015; Sullivan et al., Reference Sullivan, Grayson, Takahashi, Robertson, Maier, Bethea and Grove2010; Svirsky et al., Reference Svirsky, Levy and Avitsur2016). However, males born to mothers exposed to psychological stress during pregnancy demonstrated more depression-like behavior (Abe et al., Reference Abe, Hidaka, Kawagoe, Odagiri, Watanabe, Ikeda and Ishida2007) and females increased in aggression following DET/DES and zinc deficiency in utero exposure (Halas et al., Reference Halas, Reynolds and Sandstead1977; Palanza et al., Reference Palanza, Parmigiani, Liu and vom Saal1999). Interpretation of sex differences is complicated by the fact that a number of studies were conducted with male animals only (e.g., Abe et al., Reference Abe, Hidaka, Kawagoe, Odagiri, Watanabe, Ikeda and Ishida2007; Maccari et al., Reference Maccari, Darnaudery, Morley-Fletcher, Zuena, Cinque and Van Reeth2003). Nonetheless, existing evidence suggests fetal programming may have sexually dimorphic effects (Baillargeon, Keenan, & Cao, Reference Baillargeon, Keenan and Cao2012; Hyde, Mezulis, & Abramson, Reference Hyde, Mezulis and Abramson2008; Skinner et al., Reference Skinner, Anway, Savenkova, Gore and Crews2008). Thus, offspring sex should be considered as a moderator of links between prenatal exposures, epigenetic shifts, and behavioral outcomes.
Fifth, potential transgenerational effects linked with epigenetic mechanisms addressed in this review (e.g., Skinner, Reference Skinner2014) highlight the need for additional study of etiology, as well as preventative efforts. It is critical to understand biological/developmental processes involved in the chain of events that starts with prenatal environmental exposures. Subsequent changes in maternal health/physiology, offspring epigenome, and gene expression result in temperament profiles that typically bode risk for symptoms/behavior problems. It will be important to determine if certain exposures result in epigenetic shifts that afford plasticity, rather than risk. Studies that make connections between methylation signatures of prenatal environmental exposures and temperament profiles linked to risk could facilitate screening and preventative interventions. Szyf, Tang, Hill, and Musci (Reference Szyf, Tang, Hill and Musci2016) noted that DNA methylation could be used as a biomarker for effects of prenatal exposures on individuals’ risk and a measure of treatment progress. Thus, screening/assessment applications could be implemented once sufficient empirical evidence is generated. Links between methylation patterns resulting from in utero exposures and risky temperament phenotypes represent the first step to enable targeted selection for preventative treatment. A noninvasive biomarker (e.g., buccal cell methylation signature) could enable this intervention targeting early in infancy, rather than awaiting behavioral testing at a later time point. Thus, preventative programs could address parenting/parent–child interactions, modifiable contributors to risk for psychopathology, before maladaptive patterns become the norm. Research that identifies connections between exposures, their epigenetic signatures, and behavioral outcomes is needed to support this preventative approach. Temperamental precursors of symptoms/psychopathology offer advantages relative to the study of full-blown disorders, as these temperament risk factors can be reliably measured in the first year of life. Thus, fetal programming/epigenetic investigations with temperament outcomes will not require lengthy longitudinal efforts, although the latter are important as well. Environmental epigenetics can make a substantial contribution to the study of temperament development, informing our understanding of debilitating mental health symptoms/disorders, as well as efforts aimed at their prevention.
Supplementary Material
To view the supplementary material for this article, please visit https://doi.org/10.1017/S0954579417001730.